Abstract
Free full text

Identification and Characterization of Protective T Cells in hsp65 DNA-Vaccinated and Mycobacterium tuberculosis-Infected Mice
Abstract
Immunization by intramuscular injection of plasmid DNA expressing mycobacterial 65-kDa heat shock protein (hsp65) protects mice against challenge with virulent Mycobacterium tuberculosis H37Rv. During infection or after immunization, CD4+/CD8− and CD8+/CD4− hsp65-reactive T cells increased equally in spleens. During infection, the majority of these cells were weakly CD44 positive (CD44lo) and produced interleukin 4 (IL-4) whereas after immunization the majority were highly CD44 positive (CD44hi) and produced gamma interferon (IFN-γ). In adoptive transfer of protection to naive mice, the total CD8+/CD4− cell population purified from spleens of immunized mice was more protective than that from infected mice. When the cells were separated into CD4+/CD8− and CD8+/CD4− types and then into CD44hi and CD44lo types, CD44lo cells were essentially unable to transfer protection, the most protective CD44hi cells were CD8+/CD4−, and those from immunized mice were much more protective than those from infected mice. Thus, whereas the CD44lo IL-4-producing phenotype prevailed during infection, protection was associated with the CD8+/CD44hi IFN-γ-producing phenotype that predominated after immunization. This conclusion was confirmed and extended by analysis of 16 hsp65-reactive T-cell clones from infected mice and 16 from immunized mice; the most protective clones, in addition, displayed antigen-specific cytotoxicity.
Tuberculosis is a classic example of an infectious disease in which the disease process is caused by the immune response directed at the infectious agent. The bacteria and their products are, in themselves, not very toxic, and the extensive tissue damage, wasting, and death of the diseased individual largely constitute the immunopathology of the cell-mediated immune response. Nevertheless, it is also the cell-mediated response that protects against the disease by arresting, killing, and removing the multiplying bacteria. Whether this protective effect occurs early or late, and temporarily or permanently, determines disease progression by regulating the supply of antigen that drives the immunopathology. An important question that arises from this balance between the protective and harmful effects of the immune response is whether the antigens and immune responses that protect can be distinguished from those that harm. If so, they might be separately manipulated in new vaccines or in immunotherapy of the disease.
The T lymphocytes that regulate cellular immunity can be divided not only into the CD4+/CD8− and CD8+/CD4− phenotypes that primarily recognize exogenous and endogenous antigens, respectively (8), or into activated (memory) and nonactivated cells according to highly CD44-positive (CD44hi) and weakly CD44-positive (CD44lo) expression (5) but also into two major functionally distinct types on the basis of the profiles of cytokines that they produce. Type 1 cells (Th1 or TC1) favor development of cellular immunity (typified by gamma interferon [IFN-γ], interleukin 2 [IL-2], and IL-12 production). Type 2 cells (Th2 or TC2) favor development of antibody response (typified by IL-4, IL-6, and IL-10 production). Each type promotes differentiation of precursors into the same phenotype and inhibits development of the other phenotype (2, 27), and in consequence the type of response initiated in a microenvironment tends to be self-sustaining. IFN-γ is essential for the development of protective immunity (38) and is probably the most important factor that activates macrophages for antimycobacterial action, at least in mice (13, 34, 35). Therefore protection would be expected to be associated with an immune response in which the type 1 cytokine profile predominated. We have found that immunization procedures that present mycobacterial hsp65 to the immune system as an endogenous antigen generate strong protection against tuberculosis challenge and that this is associated with the presence of a splenic T-cell population in which CD8+/CD44hi IFN-γ-producing cytotoxic cells are prominent (28a). However, cells with a type 2 profile are also present in substantial numbers following infection (22, 39) or Mycobacterium bovis BCG vaccination (24), and the question of what contribution the other phenotypes make to protection arises. To help to answer this question we have used here the combined approaches of comparing the frequency of the different phenotypes in spleens of hsp65 DNA-vaccinated and Mycobacterium tuberculosis-infected mice and testing the different phenotypes, either as purified subpopulations or as T-cell clones, in adoptive transfer of protection.
MATERIALS AND METHODS
Infection and immunization procedures.
Young adult BALB/c mice were obtained from the vivarium of the School of Medicine of Ribeirão Preto, University of São Paulo, and were maintained under standard laboratory conditions. Infection was induced by injecting 5 × 105 viable CFU of M. tuberculosis H37Rv into a lateral tail vein. For DNA vaccination, a 3.1-kb XmnI fragment of the Mycobacterium leprae genome carrying the hsp65 gene was cloned into the EcoRV site downstream of the hydroxymethyglutaryl-coenzyme A-reductase promoter in plasmid pHMG (7). Intramuscular injection of 50 μg of plasmid DNA in 50 μl of saline into each quadriceps muscle was done on four occasions at 3-week intervals (33). For protein immunization, recombinant M. leprae hsp65 antigen (25 μg) emulsified in Freund’s incomplete adjuvant (28) was injected subcutaneously (100 μl) and then 25 μg in saline was injected intravenously twice at weekly intervals. Control animals received saline only or plasmid pHMG without an insert.
Frequencies of antigen-responsive CD4+/CD8− and CD8+/CD4− T cells.
Two weeks after completion of the immunization procedures or 30 days after infection, antigen-reactive αβ T cells with CD4+/CD8− or CD8+/CD4− phenotypes were purified from spleens by negative selection with specific monoclonal antibodies and amplification by culture (31). In brief, groups of six infected or immunized mice were killed, and splenocytes were purified from homogenized spleens by centrifugation in complete RPMI 1640 medium (RPMI-C [29]) on lympholyte M, depletion of adherent cells on tissue culture plastic, and then by two passages through nylon wool columns. Selection for subpopulations was by sequential steps of complement-mediated lysis and panning. The phenotype and purity of the T-cell subpopulations were checked with a FACScan by using fluorescein isothiocyanate immunofluorescence with rat monoclonal antibody (MAb) L3T4 or Lyt-2 against surface markers CD4 and CD8, respectively, and hamster MAb H57-597 or GL3 (Pharmingen, Uppsala, Sweden) against T-cell receptor αβ (TCR-αβ) and TCR-γδ, respectively (31). Cells purified from infected animals were found to be free from culturable bacteria. Limiting dilution analysis was used to determine the frequencies of hsp65-reactive splenocytes (31). J774-hsp65 cells were irradiated (40 Gy) and used as antigen-presenting cells in RPMI-C with 5 × 104 cells per V-bottomed well in 96-well plates. Dilutions of subpopulations of splenocytes in RPMI-C were added to give final concentrations of 2 to 4,096 T cells per well in 0.2 ml with 24 replicates. The cells were cultured in the presence of recombinant IL-2 (rIL-2) (1 ng/ml) at 37°C with 5% CO2 in air for 12 to 14 days and then pulsed with [3H]thymidine for 18 h and assessed for radiolabel incorporation (31). Wells giving counts that exceeded the counts from control (unstimulated) wells by 3 standard deviations (SD) were scored as positive. Frequencies of antigen-responsive T cells were estimated from plots of the percentage of wells that were negative against T-cell concentration, according to Poisson distribution with the minimum χ2 method. Cell concentrations at which all of the wells were positive or negative were not included in the calculations.
Cytokine ELISPOT assays.
CD4+/CD8− and CD8+/CD4− T cells prepared by negative selection from spleens as described above were cultured in the presence of irradiated (40 Gy) J774-hsp65 feeder cells (29) and rIL-2 (1 ng/ml) for 14 days and then either assayed directly or separated by fluorescence-activated cell sorting (FACS) into subpopulations of CD44lo and CD44hi cells and cultured identically for a further 12 days. These expanded hsp65-responsive T-cell subpopulations were assayed for the frequency of IFN-γ- and IL-4-producing cells by ELISPOT (18). In brief, 96-well nitrocellulose-bottomed plates (Millititre HA; Millipore Corp., Bedford, Mass.) were coated with MAb specific for IFN-γ or IL-4 (R4-GA2 or 11B11; Pharmingen) by overnight incubation at 4°C. T cells (2 × 104, 1 × 104 and 5 × 103 per 200-μl well in triplicate) were incubated with 5 × 104 irradiated J774-hsp65 cells as a source of hsp65 antigen or in the presence of J774-vector cells. After overnight incubation, cells were washed off and IFN-γ and IL-4 bound to the nitrocellulose was detected with biotinylated rat anti-mouse IFN-γ (Pharmingen) and rat anti-mouse IL-4 (BVD6-24G2; Pharmingen) followed by streptavidin-alkaline phosphatase and substrate. Spots were counted by light microscopy. The frequency of IFN-γ and IL-4-producing cells for each T-cell concentration was calculated by averaging the number of spots for triplicate wells; the overall frequency was calculated by averaging the frequencies at the different T cell-to-J774-hsp65 cell ratios.
FACS analysis of CD44 expression.
CD4+/CD8− and CD8+/CD4− T-cell subpopulations were prepared by negative selection as described above. The cells were then stained immediately with fluorescein isothiocyanate-labelled anti-CD44, Lyt-2, or L3T4 MAb (Pharmingen) and analyzed by FACScan (32), or 106 cells were incubated with 5 × 105 irradiated J774-hsp65 cells per well in 12-well plates for 7 days before staining and analysis.
Isolation of T-cell clones.
CD4+/CD8− and CD8+/CD4− T-cell subpopulations were prepared by negative selection as described above, seeded at 256 cells per well into V-bottomed 96-well plates, and cultured for 14 days in the presence of irradiated (40 Gy) J774-hsp65 feeder cells (5 × 106 in 0.1 ml) and rIL-2 (1 ng/ml). The cells were then two-color stained with anti-CD44 and Lyt-2 or L3T4 MAb and sorted by FACSort (Becton Dickinson) into CD44hi and CD44lo populations and cloned at 0.3 cells/well in round-bottomed 96-well plates in the presence of 105 feeder cells and 2 ng of rIL-2/ml. Growing clones were restimulated every 2 to 3 weeks with phytohemagglutinin (0.5 μg/ml) in the presence of feeder cells. Sixteen strongly growing CD4+/CD8− clones (four CD44lo and four CD44hi from infected mice and four CD44lo and four CD44hi from DNA-immunized mice) and 16 CD8+/CD4− clones (four CD44lo and four CD44hi from infected mice and four CD44lo and four CD44hi from DNA-immunized mice) were selected for characterization. All were TCR-αβ positive by FACScan analysis (32).
Characterization of T-cell clones.
The 16 CD4+/CD8− and 16 CD8+/CD4− clones all had the features of antigen processing and presentation expected of these phenotypes, when assessed as previously described (31). In brief, antigen specificity was checked with recombinant hsp65 (rhsp65), bovine serum albumin, purified protein derivative, and concanavalin A; the antigen-processing pathway was tested with chloroquine and brefeldin A; and the antigen presentation complex was probed with MAbs L3T4, Lyt-2, anti-I-Ad, I-Ab, and anti-H-2Kd. Secretion of cytokines IFN-γ and IL-4 was stimulated with phorbolmyristate acetate and anti-CD3 MAb YCD3-1 and measured by enzyme-linked immunosorbent assay (31). Antigen-specific cytotoxicity and toxicity for cells infected with M. tuberculosis were measured by 51Cr release from appropriate J774 or J774-hsp65 cells or thioglycollate-elicited peritoneal macrophages from BALB/c and C57BL/6 mice in the presence and absence of chloroquine and brefeldin A (31). The antimycobacterial activities of the clones and their supernatants were tested against M. tuberculosis in bone marrow-derived macrophages in the presence and absence of anti-IFN-γ MAb (31).
Adoptive transfer of immunity.
Mice were gamma irradiated (50 Gy) and then injected intravenously with 5 × 106 T cells that were either bulk purified or cloned from spleens of hsp65 DNA-immunized or M. tuberculosis-infected mice as described above. Control mice received T cells purified from spleens of normal mice or were untreated. M. tuberculosis (105 CFU) was immediately injected intravenously, the mice were killed after 4 weeks, and the numbers of CFU in spleens were determined (28).
RESULTS
Both infection with M. tuberculosis and immunization with plasmid DNA expressing hsp65 caused substantial increases in the frequency of hsp65-reactive T cells in spleens (Table (Table1).1). The increase occurred equally in cells with CD4+/CD8− and CD8+/CD4− phenotypes; this contrasted with the response to immunization with the protein in adjuvant, in which the increase was almost entirely in the CD4+/CD8− cells. Both the infection and the DNA immunization procedures increased the frequency of CD44hi cells seen in freshly harvested spleens, with the appearance of a shoulder of more-intensely staining cells on the peak of CD44lo cells (Fig. (Fig.1).1). After 7-day culture with J774-hsp65, most of the cells showed the CD44hi activated, or memory, phenotype when they were from DNA-immunized mice, whereas most were CD44lo when they were from infected animals (Fig. (Fig.2).2).
TABLE 1
Effects of immunization or infection on hsp65-reactive T-cell frequencies inspleena
Treatment | Frequency of T-cell response to Hsp65
| |
---|---|---|
CD4 | CD8 | |
rhsp65 | 1:3,550 | 1:48,000 |
hsp65 DNA | 1:1,800 | 1:1,480 |
M. tuberculosis | 1:4,900 | 1:5,500 |
Saline (control) | <1:100,000 | <1:100,000 |
Vector DNA (control) | <1:100,000 | <1:100,000 |
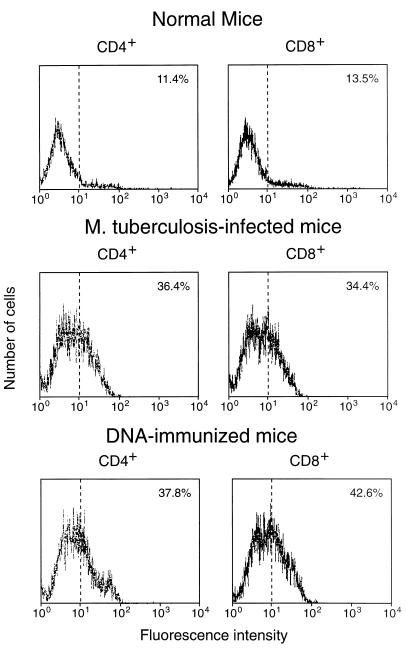
Expression of CD44 on CD4+/CD8− and CD8+/CD4− splenocytes freshly purified from hsp65 DNA-immunized, M. tuberculosis-infected, or normal control mice. The cells were stained with MAb against CD4 or CD8 (to confirm purity) and against CD44 and then analyzed by FACScan. The percentage of cells showing CD44hi fluorescence is shown in the upper right corner of each panel.
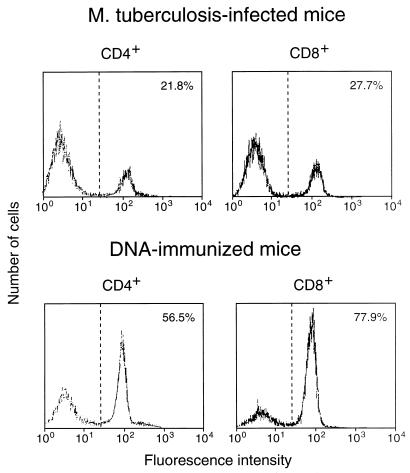
Expression of CD44 on CD4+/CD8− and CD8+/CD4− splenocytes when the cells had been amplified by 7-day culture with J774-hsp65 after purification from hsp65 DNA-immunized or M. tuberculosis-infected mice; the cells were stained with MAb against CD44 and analyzed by FACScan. The percentage of cells showing CD44hi fluorescence is shown in the upper right corner of each panel.
The hsp65-reactive cells from infected mice more often produced IL-4 than IFN-γ, irrespective of whether they were of the CD4+/CD8− or CD8+/CD4− phenotype (Fig. (Fig.3).3). The opposite was true for cells from DNA-immunized mice, where threefold more cells produced IFN-γ than IL-4, again irrespective of whether they were CD4+/CD8− or CD8+/CD4−. When the cells were further subdivided into CD44lo and CD44hi prior to assessment of the relative frequencies of IL-4- and IFN-γ-producing cells, it was seen that IL-4 production was particularly associated with CD44lo cells and IFN-γ production was particularly associated with CD44hi cells (Fig. (Fig.4).4). This was the case whether the cells had CD4+/CD8− or CD8+/CD4− phenotypes or came from infected or immunized mice. Taken together, these FACSort and ELISPOT assays indicated that in hsp65 DNA-immunized mice, the hsp65-reactive cells were more frequently CD44hi IFN-γ producers, whereas in infected mice the hsp65-reactive cells were more frequently CD44lo IL-4 producers.
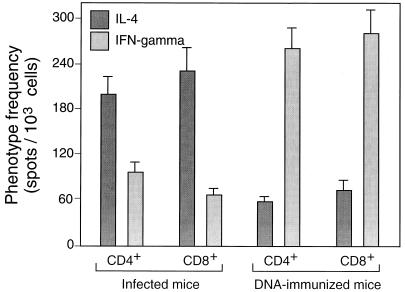
ELISPOT estimates of the frequencies of cells that produced IL-4 or IFN-γ in response to hsp65 among splenocytes from hsp65 DNA-immunized or M. tuberculosis-infected mice. Purified CD4+/CD8− and CD8+/CD4− cells were amplified by culture for 14 days on J774-hsp65 cells before assay in the presence of J774-hsp65 cells. The results shown are mean estimates (± SD) from triplicate wells at 3 different dilutions after subtraction of estimates obtained by assay in the presence of J774 vector cells (about 10 per 1,000 for CD4+/CD8− cells and 12 per 1,000 for CD8+/CD4− cells).
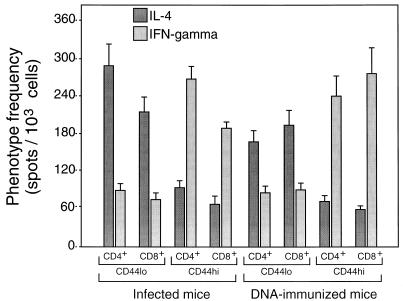
Association of IFN-γ production with CD44hi cells. Purified CD4+/CD8− and CD8+/CD4− cells were amplified by culture for 14 days on J774-hsp65 cells and then separated into CD44hi and CD44lo by FACSort and amplified for a further 12 days before assay in the presence of J774-hsp65 cells as described in the legend to Fig. Fig.33.
When tested for the ability to adoptively transfer protective immunity to naive mice, bulk CD4+/CD8− and CD8+/CD4− cells purified from spleens of either infected or DNA-immunized mice were effective (Fig. (Fig.5).5). Cells from DNA-immunized mice were more effective than those from infected mice, and CD8+/CD4− cells were more effective than CD4+/CD8− cells. Analyses of variance and t tests showed that these differences were significant (P ≤ 0.05). The CD8+/CD4− cells from mice that had been immunized with rhsp65 in adjuvant had no protective effect. When the cells for adoptive transfer experiments were further subdivided into CD44lo and CD44hi, it was seen that protection was associated with the CD44hi phenotype (Fig. (Fig.6),6), that CD44hi cells from DNA-immunized mice were more protective than CD44hi cells from infected mice, and that the most protective cells were the CD8+/CD4−/CD44hi cells from DNA-immunized mice. Analyses of variance and t tests showed that these differences were highly significant (P < 0.01).
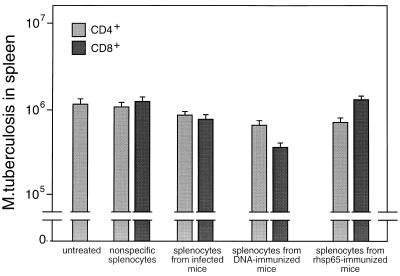
Adoptive transfer of protective immunity against tuberculosis by bulk transfer of CD4+/CD8− or CD8+/CD4− cells to naive mice. T-cell subsets were obtained by negative and positive selection from spleens of M. tuberculosis-infected or hsp65 DNA-immunized mice. Recipient mice were gamma irradiated and then injected intravenously with 5 × 106 T cells and 1 × 105 M. tuberculosis cells. The numbers of live bacteria in the spleens were determined 4 weeks after infection. Control mice were either untreated or were irradiated and reconstituted with nonspecific splenic T cells enriched from normal mice. Results are shown as mean CFU ± SD from groups of five animals.
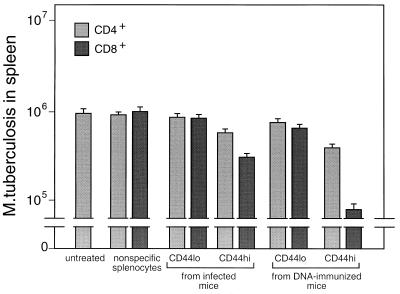
Association of protection with CD8+/CD44hi hsp65-reactive T cells. Purified CD4+/CD8− and CD8+/CD4− cells were amplified by culture for 14 days on J774-hsp65 cells and then separated into CD44hi and CD44lo types by FACSort and amplified for a further 12 days. The cells were tested for the ability to protect naive recipient mice against challenge with M. tuberculosis as described in the legend to Fig. Fig.55.
Thirty-two hsp65-reactive T-cell clones representing the CD4, CD8, and CD44 phenotypes were established, 16 from DNA-immunized mice and 16 from infected mice, and then characterized and tested for the ability to confer protection to recipient mice in adoptive transfer experiments. The 16 CD4−/CD8+ clones recognized hsp65 processed and presented via the major histocompatibility complex (MHC) class I and not the MHC class II pathway, and the 16 CD4+/CD8− clones had the converse characteristics. This was established as described in Materials and Methods by using MAbs to selectively block CD4, CD8, and specific MHC class I and II haplotypes and by using brefeldin A and chloroquine to block processing in lymphoproliferation assays (data not shown) essentially as described for clones from mice immunized with J774-hsp65 (32). As expected, most produced either IFN-γ or IL-4 (both cytokines were produced by one CD4+/CD44hi, one CD4+/CD44lo, and one CD8+/CD44lo clone from the DNA-immunized mice), some of the IFN-γ-producing clones showed antigen-dependent cytotoxicity, and they showed IFN-γ-dependent and cytotoxicity-dependent antimycobacterial activities against M. tuberculosis in macrophages (not shown). These in vitro antimycobacterial assays were done with bone marrow-derived macrophages that either were activated with clone culture supernatants and then infected or were infected and then put in cell-cell contact with a clone, both in the presence and absence of anti-IFN-γ MAb (31). Clones that produced IFN-γ and were cytotoxic were the most effective in adoptive transfer of protection (Fig. (Fig.7).7). These tended to be properties of the CD44hi clones. The CD8+/CD44hi clones with these characteristics were more effective than the corresponding CD4+/CD44hi clones. All four of the CD8+/CD44hi clones from DNA-immunized mice were of this highly protective phenotype, whereas two of the four CD8+/CD44hi clones from infected mice were almost nonprotective, were not cytotoxic, and produced IL-4.
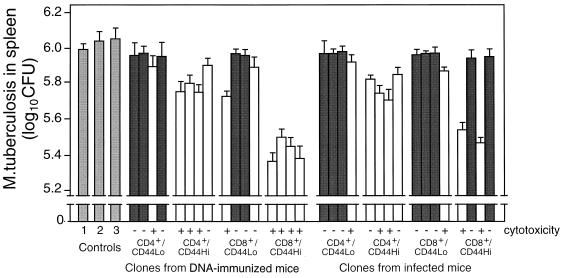
Protection by T-cell clones. Four strongly growing hsp65-responsive clones of CD4+/CD44lo and four of CD4+/CD44hi, four of CD8+/CD44lo, and four of CD8+/CD44hi phenotypes were selected from spleens of M. tuberculosis-infected and from hsp65 DNA-immunized mice. After characterization for hsp65 antigen-dependent cytotoxicity and IL-4 and IFN-γ production, they were tested for the ability to protect naive mice from challenge with M. tuberculosis as described in the legend to Fig. Fig.5.5. The numbers of live bacteria in spleens 4 weeks after challenge are shown as mean log10 ± SD for groups of five animals. Dark-shaded and unshaded bars represent data from clones that produced IL-4 and IFN-γ, respectively. Controls were as follows: 1, untreated; 2 and 3, reconstituted, respectively, with CD4+/CD8− and CD8+/CD4− clones having irrelevant antigenic specificity.
DISCUSSION
Our finding that hsp65-reactive T cells increase to remarkably high numbers during tuberculosis in mice (Table (Table1)1) is consistent with earlier studies in which up to 20% of the T cells reactive to mycobacteria were found to have this specificity (15). Clearly the antigen is an important protective immunogen that is produced in substantial amounts by the bacteria living in infected macrophages (17). Not only are mice protected against challenge with virulent M. tuberculosis by endogenous immunization with hsp65 (28, 30, 33) but we have now shown that hsp65-reactive T-cell clones from either infected or immunized mice can adoptively transfer the protection to naive animals.
In analyzing the immune responses to infection and immunization with hsp65 DNA, we have taken cells at only a single time: 30 days after infection or 2 weeks after completion of immunization. Thus the picture that we see is a “snapshot” of what are dynamic processes. Nevertheless, at these times the processes probably approximate steady-state conditions, so comparisons are meaningful; the numbers of live bacteria increase at a low but constant rate in spleens for at least 5 weeks in this infection model (28), and after DNA immunization the level of protection is essentially constant between 1 week and 8 months (unpublished data). It should be noted that the challenge model assesses the final effector stage of secondary (recall) immunity by introducing large numbers of specific T cells immediately before a large bacterial inoculum, and it is possible that some other cell is the effector for early protection in a primary response (21).
The equal increase in CD4+/CD8− and CD8+/CD4− hsp65-reactive cells seen in tuberculosis infection (Table (Table1)1) resembles that seen in mice protected by vaccination with BCG (31) or with endogenous hsp65 (reference 31 and unpublished data). Although this strong CD8+/CD4− response is consistent with the endogenous origin of the antigen and appears to be important for protection (unpublished data), there were marked differences in the profiles of memory, or activation (CD44hi), and cytokine production (IL-4 and IFN-γ) between the infected and DNA-immunized mice. Since the bulk-purified CD8+/CD4− cells from DNA-immunized mice were more efficient than those from the infected mice in transferring protection to naive animals (Fig. (Fig.5),5), we were able to assess how these differences might relate to the inadequate immunity in the infected animals, where the disease was progressing.
The lower protective efficacy of cells from infected animals was probably not due to differential entry of fewer CD8+/CD4− than CD4+/CD8− cells into the activated/memory state; the CD8-to-CD4 ratio among CD44hi hsp65-reactive cells was about 1:1 in both infected and DNA-immunized animals (Fig. (Fig.2).2). However, it might be related to a greater preponderance of IL-4-producing cells in the infected animal. This was clearly indicated by the ELISPOT assays of both CD4+/CD8− and CD8+/CD4− cells responding to hsp65 (Fig. (Fig.3).3). Furthermore, IL-4 production was associated with CD44lo cells (Fig. (Fig.4),4), suggesting a predominance of CD44lo cells in infected mice. Predominance of CD44lo cells in infected animals was also indicated by FACScan of cells cultured for 1 week with antigen (Fig. (Fig.2).2). The proportions of CD44lo and CD44hi cells in Fig. Fig.22 are a composite of the cells that have proliferated in vitro and those that have not and hence give no more than a rough guide to the quantitative differences that exist in vivo. The same considerations apply to frequencies of IL-4- and IFN-γ-producing cells. Nevertheless, there were no notable differences in the capacities of purified CD44lo and CD44hi cells or the various T-cell clones to proliferate (data not shown). Thus, it appears that the majority of hsp65-responding cells in infected mice were not memory/activated and produced IL-4, whereas the majority of hsp65-responding cells in immunized mice were activated and produced IFN-γ. Although hsp65 is only one of many antigens involved in the immune response, and others may have equal or greater roles in protective immunity, this pattern of activation and cytokine profile is likely to extend to cells with other antigen specificities through the regulatory role of the cytokines in the local environment (1, 23). The CD8+/CD44hi cells of infected animals, besides being less abundant than the CD8+/CD44hi cells of immunized mice, were also less efficient per cell in transfer of protective immunity (Fig. (Fig.6).6). The basis for this was not established. It may have been related to a lower frequency of either IFN-γ-producing cells (suggested by the data in Fig. Fig.4)4) or cytotoxic cells or to some other, unidentified factor affecting this minority population.
It should be noted that CD44hi is not a satisfactory marker for memory, or activation, in this system, since CD44lo splenocytes and clones proliferate and produce IL-4 in response to hsp65 without differentiating to CD44hi, but we have not investigated any possible specificity of the phenomenon in relation to the antigenic stimulus used. The implication is that antigen-driven proliferation and CD44hi differentiation are independent and that the trigger for differentiation was encountered in vivo but not in vitro.
The immune responses to mycobacteria, whether to BCG vaccine, M. leprae, or virulent M. tuberculosis, whether in mice or humans, are well known to show at times strong CD8+/CD4− and type 2 cytokine components, and these are associated with the later or chronic phases of the immune response (9, 24, 37). The clear preponderance of the type 2 response seen here seems unusual and might be related to the low kinetics of infection manifested in this model; whether it can occur in other animal models and in humans remains to be established. Teleologically, the CD8+/CD4− response is thought to bring cytotoxicity needed at later stages of infection to liberate residual bacteria from their intracellular havens in tissues so that they can be engulfed and killed by activated macrophages (12), and the type 2 response represents an attempt to down-regulate this to limit tissue damage (24). However, an equal balance of IFN-γ and IL-4 can potentiate the tumor necrosis factor alpha-mediated cytotoxicity of the bacteria (10). Furthermore, it is now clear that cytotoxicity can itself be associated with significant antimycobacterial action (19, 31), which makes the role of the type 2 response even more questionable.
We showed previously that although both cytotoxicity and IFN-γ can come from either CD4+/CD8−, CD8+/CD4−, or even γδ T cells, the most potent protective cell in our model is CD8+/CD4−, produces IFN-γ, and is cytotoxic (31). This conclusion is now refined by this study of new clones to include the observation that cells with that phenotype are most effective when they also express CD44hi (Fig. (Fig.7).7). It was striking that the type 2 cells had essentially no effect in our model. They might not be expected to be protective, since they produced little IFN-γ and were not cytotoxic, but their failure to interfere with expression of immunity was more remarkable. Since type 2 cytokines down-regulate T-cell differentiation for expression of type 1 cytokines in vivo and in vitro (4, 26), we may conclude that the low rate of growth of the bacteria in the model infection is not dependent on such differentiation; some other mechanism is retarding bacterial multiplication in the presence of only limited numbers of CD44hi, cytotoxic type 1 cells. The existence of such additional antimycobacterial mechanisms has been indicated in several studies, for example, in αβ T-cell depleted mice (11) and gene-deleted (knockout) mice (6, 13, 14, 16). Presumably, if the low growth rate is a consequence of residence within IFN-γ-activated macrophages, the necessary IFN-γ could be coming from alternative sources, such as γδ T cells and NK cells (3, 36), although there is no evidence that these sources are more resistant to down-regulation by type 2 cytokines than are the CD4+/CD8− or CD8+/CD4− cells (20, 25).
ACKNOWLEDGMENTS
We thank Izaira Tincani Brandão for technical assistance.
This study was supported by Fundação de Amparo à Pesquisa do Estado de São Paulo (FAPESP), Conselho Nacional de Desenvolvimento Científico e Tecnológico (CNPq), and Financiadora de Estudos e Projetos (FINEP).
REFERENCES
Articles from Infection and Immunity are provided here courtesy of American Society for Microbiology (ASM)
Full text links
Read article at publisher's site: https://doi.org/10.1128/iai.66.1.169-175.1998
Read article for free, from open access legal sources, via Unpaywall:
https://iai.asm.org/content/iai/66/1/169.full.pdf
Free after 4 months at iai.asm.org
http://iai.asm.org/cgi/reprint/66/1/169
Free after 4 months at iai.asm.org
http://iai.asm.org/cgi/content/full/66/1/169
Free to read at iai.asm.org
http://iai.asm.org/cgi/content/abstract/66/1/169
Citations & impact
Impact metrics
Citations of article over time
Alternative metrics
Article citations
Advances in the development of new vaccines for tuberculosis and Brazil's role in the effort forward the end TB strategy.
Mem Inst Oswaldo Cruz, 119:e240093, 04 Oct 2024
Cited by: 0 articles | PMID: 39383403 | PMCID: PMC11452070
Mycobacterial Hsp65 antigen upregulates the cellular immune response of healthy individuals compared with tuberculosis patients.
Hum Vaccin Immunother, 13(5):1040-1050, 06 Jan 2017
Cited by: 4 articles | PMID: 28059670 | PMCID: PMC5443371
Genomic expression catalogue of a global collection of BCG vaccine strains show evidence for highly diverged metabolic and cell-wall adaptations.
Sci Rep, 5:15443, 21 Oct 2015
Cited by: 54 articles | PMID: 26487098 | PMCID: PMC4614345
Complexity and Controversies over the Cytokine Profiles of T Helper Cell Subpopulations in Tuberculosis.
J Immunol Res, 2015:639107, 01 Oct 2015
Cited by: 18 articles | PMID: 26495323 | PMCID: PMC4606092
Review Free full text in Europe PMC
B cells expressing IL-10 mRNA modulate memory T cells after DNA-Hsp65 immunization.
Braz J Med Biol Res, 48(12):1095-1100, 18 Sep 2015
Cited by: 7 articles | PMID: 26397973 | PMCID: PMC4661025
Go to all (94) article citations
Data
Similar Articles
To arrive at the top five similar articles we use a word-weighted algorithm to compare words from the Title and Abstract of each citation.
Therapeutic efficacy of a tuberculosis DNA vaccine encoding heat shock protein 65 of Mycobacterium tuberculosis and the human interleukin 2 fusion gene.
Tuberculosis (Edinb), 89(1):54-61, 03 Dec 2008
Cited by: 23 articles | PMID: 19056317
Identification and characterization of murine cytotoxic T cells that kill Mycobacterium tuberculosis.
Infect Immun, 68(6):3269-3274, 01 Jun 2000
Cited by: 54 articles | PMID: 10816472 | PMCID: PMC97577
Immunogenicity and protective efficacy of a DNA vaccine encoding the fusion protein of mycobacterium heat shock protein 65 (Hsp65) with human interleukin-2 against Mycobacterium tuberculosis in BALB/c mice.
APMIS, 116(12):1071-1081, 01 Dec 2008
Cited by: 7 articles | PMID: 19133010
[Novel vaccines against M. tuberculosis].
Kekkaku, 81(12):745-751, 01 Dec 2006
Cited by: 8 articles | PMID: 17240920
Review