Abstract
Free full text

Binding of the Helicobacter pylori Vacuolating Cytotoxin to Target Cells
Abstract
The vacuolating cytotoxin of Helicobacter pylori, VacA, enters the cytoplasm of target cells and causes vacuolar degeneration by interfering with late stages of endocytosis. By using indirect immunofluorescence and flow cytometry, we have demonstrated that VacA binds to specific high-affinity cell surface receptors and that this interaction is necessary for cell intoxication.
Bacterial toxins interact with target cells either by direct interaction with the membrane lipids (1) or by first binding to specific receptors expressed on the cell surface (2, 12). In some cases, for example, diphtheria toxin, the toxin can interact directly with the lipid bilayer, but the presence of high-affinity cell surface receptors increases the sensitivity of the cells by several orders of magnitude (11). The Helicobacter pylori vacuolating cytotoxin binds target cells and is slowly internalized (6) in the cytoplasm, where its biologic activity is expressed (4). Here we demonstrate that the initial interaction of VacA with target cells is through high-affinity cell surface receptors and that this interaction is necessary for its biologic activity.
Saturable binding of VacA to HeLa cells.
Binding of VacA to HeLa cells was assessed by indirect immunofluorescence and flow cytometry. Purified VacA (9) from H. pylori CCUG17874 was incubated at 4°C for 1 h with 105 HeLa cells in 50 μl of phosphate-buffered saline (PBS). Nonbound VacA was removed by three washes with 150 μl of 2% fetal calf serum in PBS, and the cells were then incubated for 30 min at 4°C with saturating concentrations of anti-VacA polyclonal immunoglobulin G (IgG) (10 μg/ml). Following another wash as described above, cells were incubated for 30 min at 4°C with the appropriate dilution of fluorescein isothiocyanate-labelled anti-rabbit IgG and fixed with 1% paraformaldehyde. Cell-bound fluorescence was analyzed with a FACScan flow cytometer (Becton Dickinson). A total of 5,000 gated events were collected. Mean fluorescence intensity (MFI) values of cells were subtracted from the value obtained for cells treated in the same way but in the absence of VacA. Figure Figure1A1A shows the shift in fluorescence obtained by incubating HeLa cells with increasing concentrations of VacA. The MFI, which is an indirect measure of the number of VacA molecules bound to the cells, increased with the VacA concentration to a plateau indicating saturation of binding (Fig. (Fig.1B).1B). At each concentration of VacA, a single population of cells with a distribution not significantly wider than that of the control cells was observed, indicating that binding to the cells was relatively homogeneous and that all of the cells had similar numbers of binding sites.
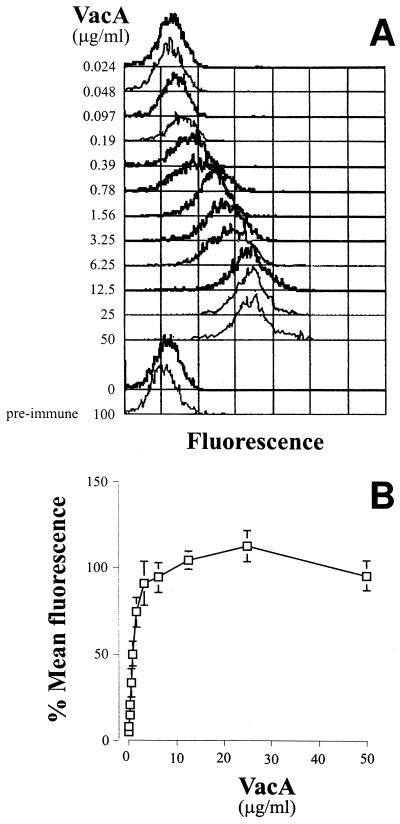
Indirect immunofluorescence and flow cytometry of VacA bound to HeLa cells. (A) Example of the curves obtained with HeLa cells incubated with increasing concentrations of VacA revealed with anti-VacA sera and fluorescein isothiocyanate-labelled anti-rabbit IgG. (B) Saturation curve of VacA binding to HeLa cells. The data are means of five independent experiments, and the bars show standard deviations. To normalize the data, each value was calculated as the percentage of the maximum MFI (average of the last three values) obtained in each respective experiment.
The asymptotic value of the saturation curves was calculated by using double-reciprocal plots. From this, the initial concentration of VacA which gave 50% maximum binding could be calculated. From the data sets from five independent experiments, 50% saturation of binding was achieved at 0.8 ± 0.14 μg/ml. Assuming the molecular mass of the oligomeric toxin from CCUG17874 to be approximately 600 kDa (7, 8), this corresponds to a dissociation constant of 1.4 nM. This value is necessarily only an estimate, since the nonspecific binding in these experiments could not be assessed, although the fact that binding reached a plateau indicates that nonspecific binding was minimal. Furthermore, a recombinant form of VacA which does not fold correctly into the oligomeric structure and is inactive (9) failed to bind (data not shown). Hence, native VacA interacts with specific, high-affinity, saturable binding sites on the HeLa cell surface.
Replacement of the rabbit antiserum with a mouse monoclonal antibody (MAb), C1G9 (14), against native VacA in these experiments gave identical results (Fig. (Fig.2A).2A). Comparison of the shift in MFI obtained by using the MAb with standard beads coated with known numbers of MAb molecules of the same isotype [Qifikit(T); DAKO, Glostrup, Denmark] indicated that at saturation approximately 45,000 MAb molecules, corresponding to 45,000 VacA monomers, were bound.
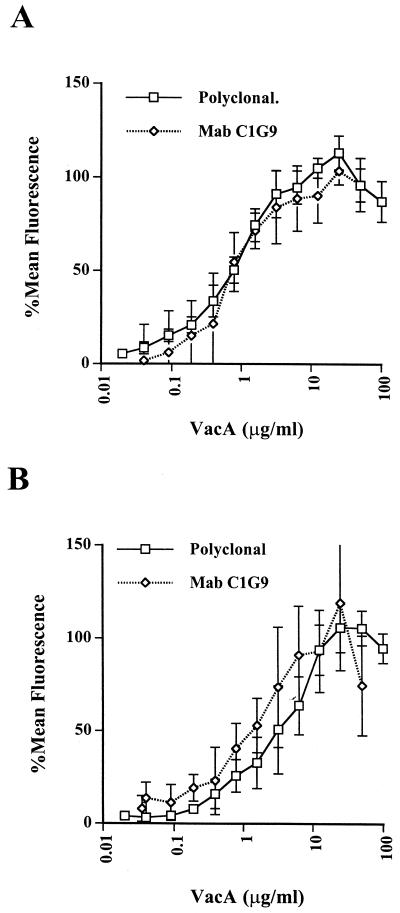
Saturation curves of native (A) or acid-treated (B) VacA binding to HeLa cells obtained by using rabbit polyclonal anti-VacA antibodies or anti-VacA MAb C1G9, as indicated. The data are from four or five independent experiments for each curve. Data were normalized as described in the legend to Fig. Fig.11B.
Binding of activated VacA to HeLa cells.
VacA purified from H. pylori culture supernatants is essentially inactive. However, treatment at a pH below 5 results in a conformational change in the molecule which is associated with a large increase in vacuolating activity (5). The increase in activity was, however, not associated with an increase in VacA binding affinity. In fact, in several experiments using either polyclonal antibodies or MAbs, 50% saturation of VacA in PBS which had been brought to pH 5.0 by the addition of HCl for 15 min at 37°C and then neutralized with NaOH was 1.8 to 2.3 μg/ml (Fig. (Fig.2B).2B). As can be seen from the error bars in Fig. Fig.2B,2B, the interexperimental variation in the data on binding of activated VacA was considerably more noticeable than in assays of the binding of native VacA. Although the difference was barely significant (P < 0.02), the slightly lower binding affinity of acid-treated VacA was consistently observed. Cover et al. (3) recently demonstrated that VacA oligomers dissociate at low pH but reoligomerize on neutralization. It is likely that the variation in binding affinity is due to the varying efficiency of VacA reoligomerization in the neutral binding buffer.
Competitive inhibition of active VacA.
While it is clear that the large increase in the biological activity of acid-activated toxin is not due to an increase in receptor affinity, the possibility could not be excluded that the associated conformational change did not permit interaction of the toxin with different, functional receptors. To approach this question, we used inactive VacA to compete with acid-activated material in an assay of biologic activity. Two forms of inactive VacA were used. First, VacA which had not been treated at low pH was used. This material had low but detectable activity, probably due to a small quantity of the protein in the active state. Second, VacA which had been completely detoxified by treatment with 1.6 mM formaldehyde–25 mM lysine for 48 h at 37°C was used (10). This formaldehyde treatment has been shown to completely detoxify VacA without extensive disruption of its conformation (10). The formaldehyde-treated toxin bound to HeLa cells with an affinity similar to that of the native, nonactivated toxin (Fig. (Fig.3A).3A). Coincubation of HeLa cells with increasing concentrations of either of the inactive forms of VacA together with 20 μg of the biologically active toxin per ml resulted in a dose-dependent reduction of vacuolation as measured by the neutral red uptake assay (13) (Fig. (Fig.3B).3B). Interestingly, both inactive forms of the toxin caused a 50% reduction in activity at a ratio of approximately 1:3 of inactive to activated protein, in accordance with the higher apparent binding affinity of the inactive material. We conclude that both the active toxin and the inactive toxin bind the same receptor and that this binding is associated with biological activity.
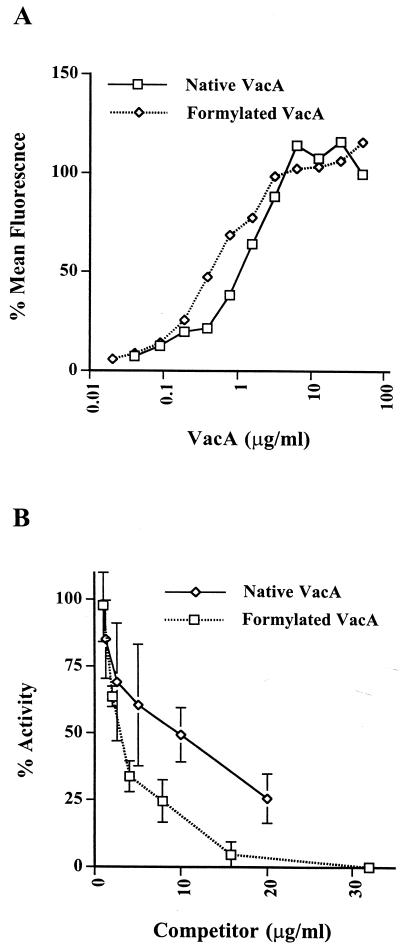
Inhibition of acid-activated VacA activity on HeLa cells by inactive VacA. (A) Curves of native and formaldehyde-inactivated VacA binding to HeLa cells. Data are normalized as described in the legend to Fig. Fig.1B.1B. (B) Inhibition of VacA activity by native or formaldehyde-inactivated VacA. The values are percent inhibition of acid-activated VacA in the HeLa cell vacuolation assay as measured by neutral red uptake.
VacA binding to different cell types.
Figure Figure44 shows curves of VacA binding to HeLa cells, a human intestinal epithelial cell line (KATO III), a mouse epithelial cell line (NIH 3T3), and a human T-lymphocyte cell line (Jurkat). All three of the epithelial cell lines bound VacA with similar affinities, indicating that the receptor structure is also conserved in mouse cells. Jurkat T-lymphoma cells, on the other hand, showed only very weak binding, which could not be distinguished from nonspecific binding since the curve did not show clear saturation. Hence, the receptor does not appear to be a ubiquitously expressed structure present on all cells, at least in large numbers.
Conclusions.
We have shown concentration-dependent binding of VacA to target cells revealed by indirect immunofluorescence and flow cytometry. The binding is specific and saturable, with an apparent dissociation constant in the nanomolar range. Taken together, these data indicate the presence of a major class of high-affinity receptors for VacA on the target cell surface. Recently, Yahiro et al. (15) described a 140-kDa cell surface protein that could be immunoprecipitated with VacA and anti-VacA antibodies which may be the receptor.
Acknowledgments
We thank C. T. Baldari for access to flow cytometry facilities and for helpful discussion and G. Corsi for artwork.
This work was supported by European Commission contract IC18CT950024.
REFERENCES
Articles from Infection and Immunity are provided here courtesy of American Society for Microbiology (ASM)
Full text links
Read article at publisher's site: https://doi.org/10.1128/iai.66.8.3981-3984.1998
Read article for free, from open access legal sources, via Unpaywall:
https://iai.asm.org/content/iai/66/8/3981.full.pdf
Free after 4 months at iai.asm.org
http://iai.asm.org/cgi/content/full/66/8/3981
Free after 4 months at iai.asm.org
http://iai.asm.org/cgi/reprint/66/8/3981
Free to read at iai.asm.org
http://iai.asm.org/cgi/content/abstract/66/8/3981
Citations & impact
Impact metrics
Citations of article over time
Smart citations by scite.ai
Explore citation contexts and check if this article has been
supported or disputed.
https://scite.ai/reports/10.1128/iai.66.8.3981-3984.1998
Article citations
Determinants of Raft Partitioning of the Helicobacter pylori Pore-Forming Toxin VacA.
Infect Immun, 86(5):e00872-17, 23 Apr 2018
Cited by: 13 articles | PMID: 29531133 | PMCID: PMC5913846
An Overview of Helicobacter pylori VacA Toxin Biology.
Toxins (Basel), 8(6):E173, 03 Jun 2016
Cited by: 93 articles | PMID: 27271669 | PMCID: PMC4926140
Review Free full text in Europe PMC
Helicobacter pylori tumor necrosis factor-α inducing protein promotes cytokine expression via nuclear factor-κB.
World J Gastroenterol, 19(3):399-403, 01 Jan 2013
Cited by: 18 articles | PMID: 23372364 | PMCID: PMC3554826
Helicobacter pylori detection and genotyping in gastric biopsy specimens from Chennai patients (India).
Can J Microbiol, 55(2):126-132, 01 Feb 2009
Cited by: 4 articles | PMID: 19295644
Integrin subunit CD18 Is the T-lymphocyte receptor for the Helicobacter pylori vacuolating cytotoxin.
Cell Host Microbe, 3(1):20-29, 01 Jan 2008
Cited by: 67 articles | PMID: 18191791
Go to all (37) article citations
Similar Articles
To arrive at the top five similar articles we use a word-weighted algorithm to compare words from the Title and Abstract of each citation.
Fluorescence resonance energy transfer microscopy of the Helicobacter pylori vacuolating cytotoxin within mammalian cells.
Infect Immun, 70(7):3824-3832, 01 Jul 2002
Cited by: 17 articles | PMID: 12065526 | PMCID: PMC128058
Acid activation of Helicobacter pylori vacuolating cytotoxin (VacA) results in toxin internalization by eukaryotic cells.
Mol Microbiol, 37(2):433-442, 01 Jul 2000
Cited by: 58 articles | PMID: 10931337
Plasma membrane cholesterol modulates cellular vacuolation induced by the Helicobacter pylori vacuolating cytotoxin.
Infect Immun, 70(8):4112-4123, 01 Aug 2002
Cited by: 52 articles | PMID: 12117919 | PMCID: PMC128184
Helicobacter pylori vacuolating cytotoxin, VacA.
Jpn J Infect Dis, 55(1):1-5, 01 Feb 2002
Cited by: 0 articles | PMID: 11971154
Review