Abstract
Background
Most cancer cells are more glycolytic even under aerobic conditions compared with their normal counterparts. Recent evidence of tumor cell metabolism, however, shows that some tumors also increase mitochondrial oxidative phosphorylation (ox-phos) at some disease states during progression and/or development of drug resistance. Our data show that anti-androgen enzalutamide (ENZA) resistant prostate cancer (PCa) cells use more mitochondrial metabolism leading to higher ox-phos as compared to the ENZA-sensitive cells and can become vulnerable to mitochondrial metabolism targeted therapies.Methods
Seahorse assay, mass spectrometry and high resolution fluorescence confocal microscopy coupled with image analysis has been used to compare mitochondrial metabolism in ENZA-treated and -untreated anti-androgen-sensitive LNCaP and -resistant C4-2, CWR22ν1, and PCa2b cells. Ex vivo fluorescence microscopy and image analysis has been standardized to monitor mitochondrial electron transport (ETS) activity that likely increases ox-phos in circulating tumor cells (CTCs) isolated fom patients undergoing AR-targeted therapies.Results
Our data show that PCa cells that are resistant to anti-androgen ENZA switch from glycolysis to ox-phos leading to an increased ETS activity. ENZA pretreated cells are more vulnerable to ETS component complex I inhibitor IACS-010759 (IACS) and mitochondrial glutaminase inhibitor CB-839 that reduces glutamate supply to tricarboxylic acid cycle. CTCs isolated from 6 of 20 patient blood samples showed relatively higher ETS activity than the rest of the patients. All six patients have developed ENZA resistance within less than 6 months of the sample collection.Conclusion
The enhanced growth inhibitory effects of mitochondrial metabolic inhibitors IACS and CB-839 in ENZA pretreated PCa cells provides a rationale for designing a drug combination trial. Patients can be selected for such trials by monitoring the mitochondrial ETS activities in their CTCs to maximize success.Free full text

Prostate cancer cells survive anti-androgen and mitochondrial metabolic inhibitors by modulating glycolysis and mitochondrial metabolic activities
Abstract
Background:
Most cancer cells are more glycolytic even under aerobic conditions compared with their normal counterparts. Recent evidence of tumor cell metabolism, however, shows that some tumors also increase mitochondrial oxidative phosphorylation (ox-phos) at some disease states during progression and/or development of drug resistance. Our data show that anti-androgen enzalutamide (ENZA) resistant prostate cancer (PCa) cells use more mitochondrial metabolism leading to higher ox-phos as compared to the ENZA-sensitive cells and can become vulnerable to mitochondrial metabolism targeted therapies.
Methods:
Seahorse assay, mass spectrometry and high resolution fluorescence confocal microscopy coupled with image analysis has been used to compare mitochondrial metabolism in ENZA-treated and -untreated anti-androgen-sensitive LNCaP and -resistant C4–2, CWR22ν1, and PCa2b cells. Ex vivo fluorescence microscopy and image analysis has been standardized to monitor mitochondrial electron transport (ETS) activity that likely increases ox-phos in circulating tumor cells (CTCs) isolated fom patients undergoing AR-targeted therapies.
Results:
Our data show that PCa cells that are resistant to anti-androgen ENZA switch from glycolysis to ox-phos leading to an increased ETS activity. ENZA pretreated cells are more vulnerable to ETS component complex I inhibitor IACS-010759 (IACS) and mitochondrial glutaminase inhibitor CB-839 that reduces glutamate supply to tricarboxylic acid cycle. CTCs isolated from 6 of 20 patient blood samples showed relatively higher ETS activity than the rest of the patients. All six patients have developed ENZA resistance within less than 6 months of the sample collection.
Conclusion:
The enhanced growth inhibitory effects of mitochondrial metabolic inhibitors IACS and CB-839 in ENZA pretreated PCa cells provides a rationale for designing a drug combination trial. Patients can be selected for such trials by monitoring the mitochondrial ETS activities in their CTCs to maximize success.
1 |. INTRODUCTION
Prostate cancer (PCa) is the second leading cause of cancer deaths among US men. In the last year, over 186,000 men were newly diagnosed with PCa and more than 30,000 of them died of the disease. Clinical management of PCa is challenging due to diverse clinical outcomes.1 While a majority of the patients, who are diagnosed at the early stage of the disease are cured by the first line of treatment, a substantial portion of them progress to advanced, metastatic disease, which responds poorly to most cancer therapies. Despite intense research during the last decade, the mechanism of developing anti-androgen-resistance remains mostly unknown.
Activation of the androgen receptor (AR) remains central to most PCa growth, recurrence and progression.1,2 Androgen-activated AR translocates to the nucleus and initiates expression of genes that are involved in PCa proliferation. Targeting the androgen-signaling axis using androgen deprivation therapies (ADT) is widely used in patients with progressing PCas.1–3 Most patients respond to such therapies for a while but eventually return to the clinic with castrate-resistant PCa (CRPC) that grows in low serum androgen. The mechanism(s) that turns androgen dependent PCa (ADPC) to CRPC remains mostly unknown. This is a major hurdle to early identification of patients likely to develop CRPC as well as developing new therapies for patients with potential to progress.
In recent years, much effort has been devoted to identifying and understanding genomic and epigenomic changes that drive PCa progression. Despite some progress in our understanding of genomic and epigenomic alterations linking to CRPC and/or metastatic CRPC such as aggressive variant (AVPC) and neuroendocrine (NEPC) variant diseases, only a few genomic markers made significant impact on clinical management of PCa, thus far. This is because many ADT and anti-androgen therapy resistant, lethal, metastatic PCas showed little genomic difference from their organ confined counterparts.4 Translational studies to better interpret the genomic data to predict disease progression and therapy resistance are only currently being planned.
Nearly a century ago Otto Warbürg and his coworkers demonstrated that most cancer cells use more glycolysis for their energy need compared with their normal counterparts incubated under identical condition (Warbürg effect).5 Based on this principle, 18F-labeled 2-deoxy-2-fluoro-D-glucose (18F-FDG) that uses cellular glucose uptake pathway was approved by FDA for cancer imaging in patients by positron emission tomography (PET). PCa, however, generally exhibits weak FDG-PET intensity except at some aggressive, therapy resistant state and/or specific organ site(s) of metastasis.6,7 This led to our current assumption that certain metabolic adaptation happens during PCa progression and/or during the development of therapy resistance, albeit the exact role of metabolic changes leading to such effect remains elusive.
In addition to glycolysis, tumor cells also engage mitochondrial ox-phos to support growth. Enhanced mitochondrial ETS activity is responsible for cell proliferation and invasion of a variety of cancer cell lines.8 In the last 5 years, the concept of ATP production by fatty acids and/or amino acid oxidation under certain conditions has been realized in cancer cells.9 Proper maintenance of mitochondrial membrane potential by the ETS is also essential to prevent apoptosis and to support proliferation.10 It has been reported that ovarian and esophageal cancer cells switch from glycolysis to ox-phos, while transitioning from proliferative to invasive state.11,12 Depending on the disease state, some tumors, including PCa, can also exhibit an intermediate metabolic phenotype performing a truncated or reversed tri-carboxylic acid (TCA) cycle in the mitochondria that export certain TCA cycle intermediates such as citrate, α-keto-glutarate (α-KG), pyruvate, and so forth to the cytoplasm13 and related references therein. These could then be utilized in fatty acid, amino acid and nucleotide biosynthesis in the rapidly dividing cells. Targeting mitochondria in these cells could be an effective strategy to block cell invasion and proliferation.
The last 5 years has seen an increased effort in developing more specific mitochondria targeting agents for anticancer drug development. Among these, targeting mutant IDH1 inhibitor, AG-120, and a glutaminase inhibitor, CB-839, are currently undergoing Phase II clinical trials either as a single agent or in combination with other approved therapies in acute myeloid leukemia (AML)14 and renal cell carcinoma,15 respectively. Several other agents targeting glucose transporter 1 (Glut1)******, hexokinase, pyruvate kinase M2 (PKM2) **** and a mitochondrial pyruvate transporter are currently undergoing preclinical development and/or entered early Phase clinical trials.16 A new agent IACS-010759 (IACS) specifically targeting mitochondrial Complex I17 in the ETS has been developed in our Institution and is undergoing a Phase I trial against AML and other solid tumors including PCa. To establish an effective mitochondrial metabolism targeting agent against PCa, it is imperative to understand the disease state, when tumors are mostly dependent on this pathway for survival and therefore, will be most vulnerable to these agents. Other therapies that can modify the tumor metabolism that is more akin to that of this disease state should also enhance the efficacies of these novel agents. Monitoring the mitochondrial ETS activity that relates to ox-phos status using minimally invasive circulating tumor cell (CTC) analysis will help identify the disease state as well as could be used as a pharmacodynamic marker for clinical trial/use of these agents.
The data presented here show that PCas adapt to anti-androgen therapies by switching from glycolysis to ox-phos for their bioenergetic need and such adaptation can be monitored in patients by analyzing mitochondrial ETS activity in CTCs in patient blood samples; we also propose that PCa tumors at this state will be most vulnerable to the mitochondrial metabolism targeted therapies.
2 |. MATERIALS AND METHODS
2.1 |. Cell lines
LNCaP and CWR22ν1 cells are obtained from American Type Culture Collection (ATCC). C4–2 cells are a kind gift from Professor Leelund Chung, University of California, Los Angeles (UCLA) and PCa2b cells are a kind gift from Nora Navone, Genitourinary Oncology, University of Texas MD Anderson Cancer Center.
2.2 |. Seahorse analysis
Cells were cultured (20,000 cells per well) on a 96-well Seahorse plate in Dulbecco’s modified Eagle’s medium (DMEM) media supplemented with 1% FBS and 4% charcoal stripped FBS (DMEMF1C4)18 at 37°C, 5% CO2 for 48 h. Test compound at desired concentrations was then added and incubated for 24 h. Cells were then assayed following manufacturer supplied glycolysis and oxygen consumption protocols (Agilent). The extracellular acidificationa rate (ECAR) data are then corrected to measure the acidification rate exclusively due to glycolysis by subtracting the pH change due to mitochondrial metabolic activity (as measured from cellular oxygen consumption rate [OCR]) and expressed as glycolytic proton excretion rate (GlycoPER) using the manufacturer supplied algorithm.
2.3 |. Lactate/pyruvate assay
A high pH HILIC method was developed on the Agilent 6560 LC-IM-QTOF-MS. A Waters® BEH Amide column (2.1 × 100 mm, 3.5 μm) was used with a gradient of Buffer A: 95:5 H2O/ACN + 20 mM NH4AcO + 20 mM NHOH (adjusted to pH 9) and Buffer B: 5:95 H2O/acetonitrile.
2.4 |. DNA assay
Cell growth was assayed using our published DNA assay used routinely in our laboratory.18
2.5 |. MitoSOX Red (MSR) dye fluorescence with MitoTracker Green (MTG) dye
The PCa cells plated in each well of a 96-well plate were treated with desired concentrations of test agents for 96 h. Cells were then incubated with MSR for 3.5 h to stain superoxide producing mitochondria followed by MTG dye for 30 min to stain all mitochondria.19 The nonoverlapping fluorescence emission intensities of MSR and MTG were determined in a fluorescence plate reader.
2.6 |. Microscope estimation for MSR fluorescence
At the completion of the treatment, cells were treated with MSR dye that specifically binds to the mitochondrial membrane and fluoresces upon oxidation only by the superoxide generated by the mitochondrial ox-phos activity19 following manufacturer supplied protocol (Invitrogen). All microscopic images of mitochondrial fluorescence were collected at ×500 maginification using a ×100 stimulated emission depletion (STED) objective in a high resolution Leica SP8 STED fluorescence laser confocal microscope equipped with UV and white light laser using sequential scanning and observation protocol at desired excitation and emission wavelengths at 50–100 nm resolution and analyzed using Image J image analysis software. Mitochondrial intensities in cells in each focal plane at 0.5-μM-thick optical slices and mean pixel intensities of all mitochondria in the field were calculated after appropriate background subtraction to reduce the nuclear fluorescence to zero. Images of cells in 5–10 random areas (depending on cell density) on each slide containing about 600–1200 mitochondria were calculated. Each experiment was repeated twice and all data were normalized to the fluorescence of the control cells.
2.7 |. Patient samples
Patients with CRPC undergoing AR-targeted therapies such as enzalutamide (ENZA) or abiraterone in combination ADT therapy were recruited and provided written informed consent under IRB approved protocol PA15–0956 in accordance with the provisions of the Declaration of Helsinki.
2.8 |. Enrichment and immunocytochemistry (ICC) staining of CTCs
Patient blood sample (10 ml) was collected by venipuncture, stored in a chilled collection tube under an IRB approved protocol and CTCs were isolated using a Parsortix microfluidic system Angle Europe Limited. The system enriches tumor cells based on size and deformability without biasing the selection with any pre-determined surface antigen.20 After filtering blood through a 6.5-μm critical gap, reversing the flow of buffer allows for enrichment of live CTCs. CTCs were confirmed by ICC staining of nucleated cells for AR, EpCam and pan-cytokeratine.21 We used the same method to identify AR-positive nucleated PCa CTCs from the patient-derived xenograft (PDX) MDA-PCa-203 and also from a patient blood sample. The conditions for blood collection and storing in ice for less than 30 min before CTC isolation remain identical for all samples to control for any metabolic alterations during collection, transport and isolation.
2.9 |. Quantification of mitochondrial superoxide production in CTCs
CTCs were plated in a chamber slide and were blinded for patient information using a pathologist supplied coding. Cells in some chambers were incubated with MSR dye for 4 h in a 37°C CO2/air incubator. Cells are then fixed and stained with Hoechst 33342 (Molecular Probes, Inc.), primary anti-human AR (Santa Cruz Biotechnology, Inc.) with secondary AlexaFluor 488 (Invitrogen) and primary EpCAM (Abcam) with secondary AlexaFluor 680 (Invitrogen) following our standardized immunocytochemistry protocol (see above).
2.10 |. Statistical analysis
Patient disease outcome information was unblinded and subjected to rigorous statistical analysis. Mitochondrial data for CTCs were summarized using descriptive statistics. Linear mixed model was fitted to compare data between responding (samples 1–6 and 8–13) and non-responding (samples 14–20) patients, including patients as random effects to account for the intra-cluster correlation for clustered data. Wilcoxon rank sum test was also used for two group comparisons. SAS9.4 software (SAS Institute) was used for the analysis.
3 |. RESULTS
3.1 |. ADPC cells are more glycolytic than are CRPC cells
Cellular OCR and pH changes in the media due to cellular acid efflux (ECAR; mostly lactic acid from glycolysis) by cultured cells can be measured by Seahorse assay at a high precision.22 This assay has now been accepted as a gold standard for measuring changes in glycolysis and cellular oxygen consumption (mostly due to mitochondrial ETS activity) in cultured cells.22,23 The ECAR data from ADPC LNCaP, LNCaP-derived CRPC C4–2 and PDX CRPC PCa2b cells isolated from a patient’s bone lesion were incubated in androgen reduced media (see Section 2). The results are shown in Figure 1A. The corresponding basal OCR data are shown in Figure 1B. All Seahorse data are normalized to viable cell counts (~85%–90% viable under these conditions) as determined by a propidium iodide dye fluorescence assay performed after the completion of the Seahorse experiment.24 The data show that C4–2 and PCa2b cells are significantly less glycolytic and use more mitochondrial metabolism than do the LNCaP cells.
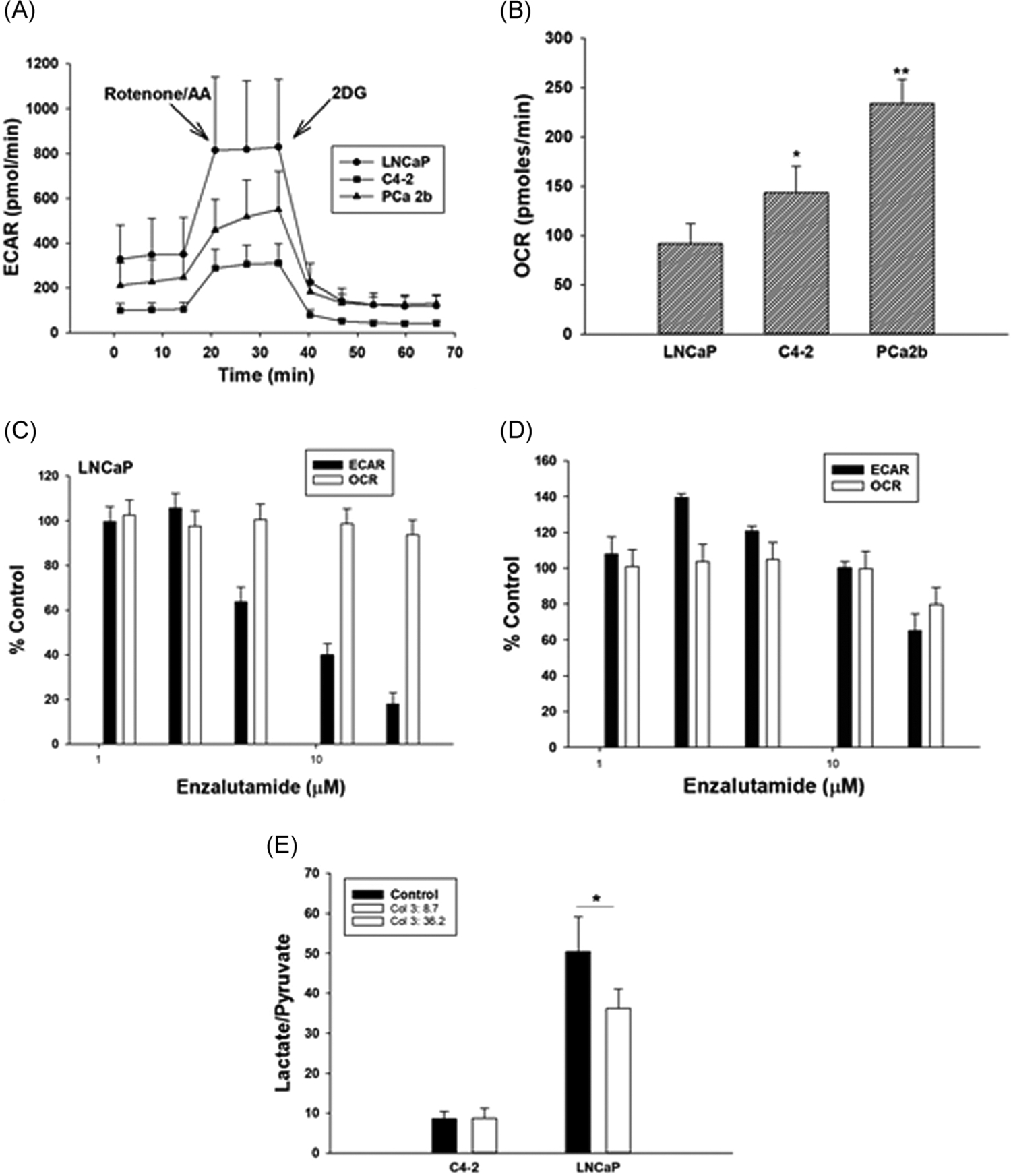
(A) Glycolysis (ECAR) and (B) oxygen consumption rate (OCR) in LNCaP, C4–2 and PCa2b cells grown in androgen reduced media for 48 h; (C) ECAR and OCR in LNCaP and (D) C4–2 cells grown in androgen depleted media for 48 h and then treated with ENZA for 24 h. All ECAR and OCR data were normalized to viable cells as determined by propidium iodide staining (see text) at the end of each assay. Each data point and error bar represent the mean and standard deviation of readings from 15 separate wells repeated three times. (E) Mass spectroscopic analysis of lactate/pyruvate ratio in C4–2 and LNCaP cells incubated in androgen depleted medium for 48 h and then treated for 24 h with ENZA at their respective IC50 doses (20 and 10 μM, respectively). Each data point and the error bar are the mean and standard deviation of 2 parallel samples run at least twice. *p < .01, **p < .001. ECAR, extracellular acidificationa rate; ENZA, enzalutamide; OCR, oxygen consumption rate
3.2 |. Anti-androgen ENZA reduces glycolysis in viable PCa cells
The ECAR and OCR in ENZA-sensitive LNCaP and ENZA-resistant C4–2 cells treated for 24 h with graded concentrations of ENZA within achievable human serum level (22 μM)25 are shown in Figure 1C,,D.D. ENZA significantly lowers the ECAR with small changes in OCR in both cell lines. These data have been further confirmed by determining the changes in total (cellular+media) lactate/pyruvate ratio using mass spectroscopy (Figure 1E). LNCaP cells produce more lactate and less pyruvate than do C4–2 cells confirming the Seahorse data. ENZA significantly lowers lactate levels in the ENZA-sensitive LNCaP, but not in the -resistant C4–2 cells at or near their respective IC50 doses.
3.3 |. Prolonged treatment with ENZA increases mitochondrial superoxide production
ENZA treatment for 72–96 h at respective IC50 doses did not produce enough viable PCa cells for reliable Seahorse assay results. To circumvent this problem, we have standardized a confocal microscopy method coupled with an image analysis assay to estimate mitochondrial ETS activity based on superoxide production25 (see Section 2). The representative fluorescence images of LNCaP and C4–2 cells after 96 h treatment with ENZA (at or near the IC50 doses, see above) were obtained using a fluorescence STED confocal microscope (Figure 2A). The fluorescence intensities of MSR dye are quantitated (see Methods for detail) by Image J segmentation (Figure 2B). The data are shown in Figure 2C–E. ENZA-treatment significantly increases the MSR fluorescence intensity in the ENZA-resistant CRPC C4–2 and CWR22ν1 cells as compared to the control untreated cells (Figure 2D,,E).E). In ENZA-sensitive LNCaP cells the increase observed in not statistically significant (Figure 2C). The effect of ENZA is almost completely reversed by androgen treatment suggesting the changes are mainly due to an on-target effect of ENZA interacting with AR.
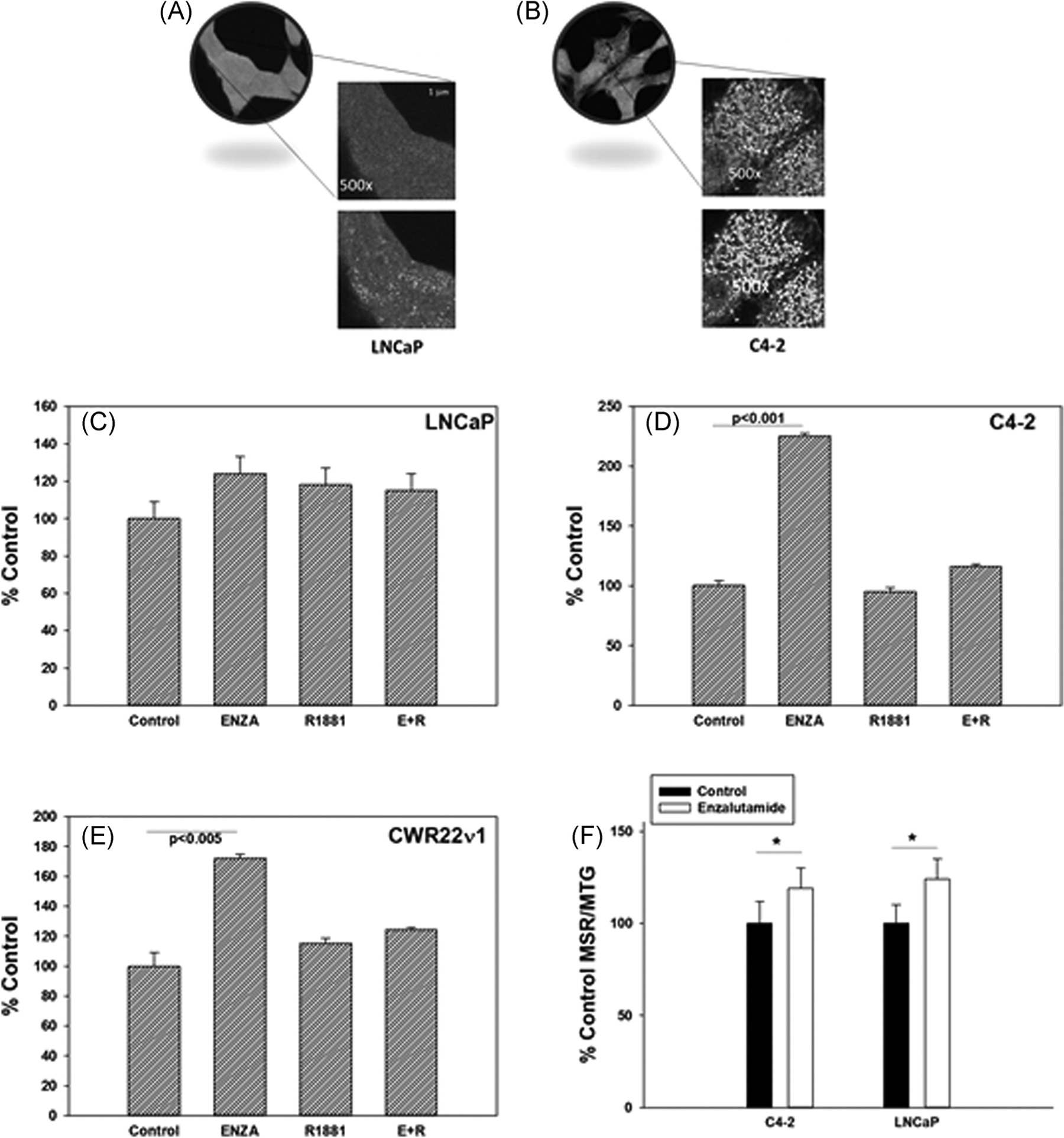
Representative confocal microscopy images of an optical section at ×100 and ×500 magnification each of (A) LNCaP and (B) C4–2 cells treated first 24 h with ENZA at their respective IC50 doses (10 and 20 μM, respectively) and then incubated with MSR dye for 4 h and examples of corresponding Image J segmentation (bottom section) for fluorescence intensity analysis. Mean pixel fluorescence intensities of oxidized MSR dye in the mitochondria were calculated from individual mitochondrion MSR fluorescence image quantitation and averaged over all mitochondria per cell as described in the text. of (C) LNCaP, (D) C4–2 and (E) CWR22ν1 cells treated with vehicle control, 10 μM ENZA (LNCaP) and 20 μM ENZA (C4–2 and CWR22ν1), androgen mimetic 2 nM R1881, and ENZA + R1881 (E + R) were calculated from individual mitochondrion MSR fluorescence image quantitation and averaged over all mitochondria per cell as described in the text. (F) MSR/MTG in C4–2 and LNCaP cells treated for 96 h with vehicle (control) and with IC50 dose of ENZA. Each data point and standard deviation are the mean of the readings from six wells run in triplicates and repeated at least three times. *p < .05, **p < .005. ENZA, enzalutamide; MSR, MitoSOX Red; MTG, MitoTracker Green
To confirm that the microscopy image analysis data are not due to any selection bias, a macroscale assay to quantitate mitochondrial activities has also been standardized. The MSR/MTG ratio represent the mitochondrial ox-phos normalized per mitochondrion. The increase in this ratio for LNCaP and C4–2 cells treated with ENZA compared with the ratio in untreated control cells is small but significant (Figure 2F). This confirms the microscopic observation of relatively higher mitochondrial ETS activity in the cells that survive in the presence of ENZA. The relative increases in the MSR/MTG ratio is less in C4–2 cells than that observed in their microscopic images. This could be because the macroscale assay may include some mitochondrial staining by MTG in the dead cells that have reduced or stopped ETS activity and thus, do not cause MSR fluorescence, but still retain some membrane poteintial for MTG staining. These cells are not included in microscopic analysis. On the other hand, the increasing trend of ox-phos in LNCaP cells observed in the microscopy assay, but did not attain statistical significance did reach statistical significance in the 96-well plate-based assay due to the ability to analyze a lot more cells as compared with the limited number of fields analyzed using the high resolution microscopy.
3.4 |. Cells that switch to ox-phos are more vulnerable to mitochondria targeted agents
It is hypothesized that cells depending more on ox-phos for their energy need should be more vulnerable to mitochondrial metabolism targeted agents. To establish this, we used two agents – IACS-010759 (IACS) and CB-839 that are currently undergoing clinical trials as anticancer drugs. IACS specifically targets complex I of the mitochondrial ETS17 and CB-839 inhibits glutaminase26 that produces glutamate from glutamine, which is then converted to α-ketoglutarate α-KG and used as one of the fuels for the TCA cycle.
We have determined the effects of both agents on ECAR (see above) in LNCaP and C4–2 cells with or without ENZA pretreatment for 24 h. The results are shown in Figure 3A–F. Treatment with IACS or CB-839 decreases ox-phos with a concomitant increase in glycolysis in the surviving cells (Figure 3A,,CC,,E).E). Pretreatment with ENZA at close to the IC50 doses of the respective cell lines (10 μM for LNCaP and 20 μM for C4–2) for 24 h before IACS addition markedly blocks the observed increase in glycolysis in both cell lines (Figure 3B,,DD,,FF).
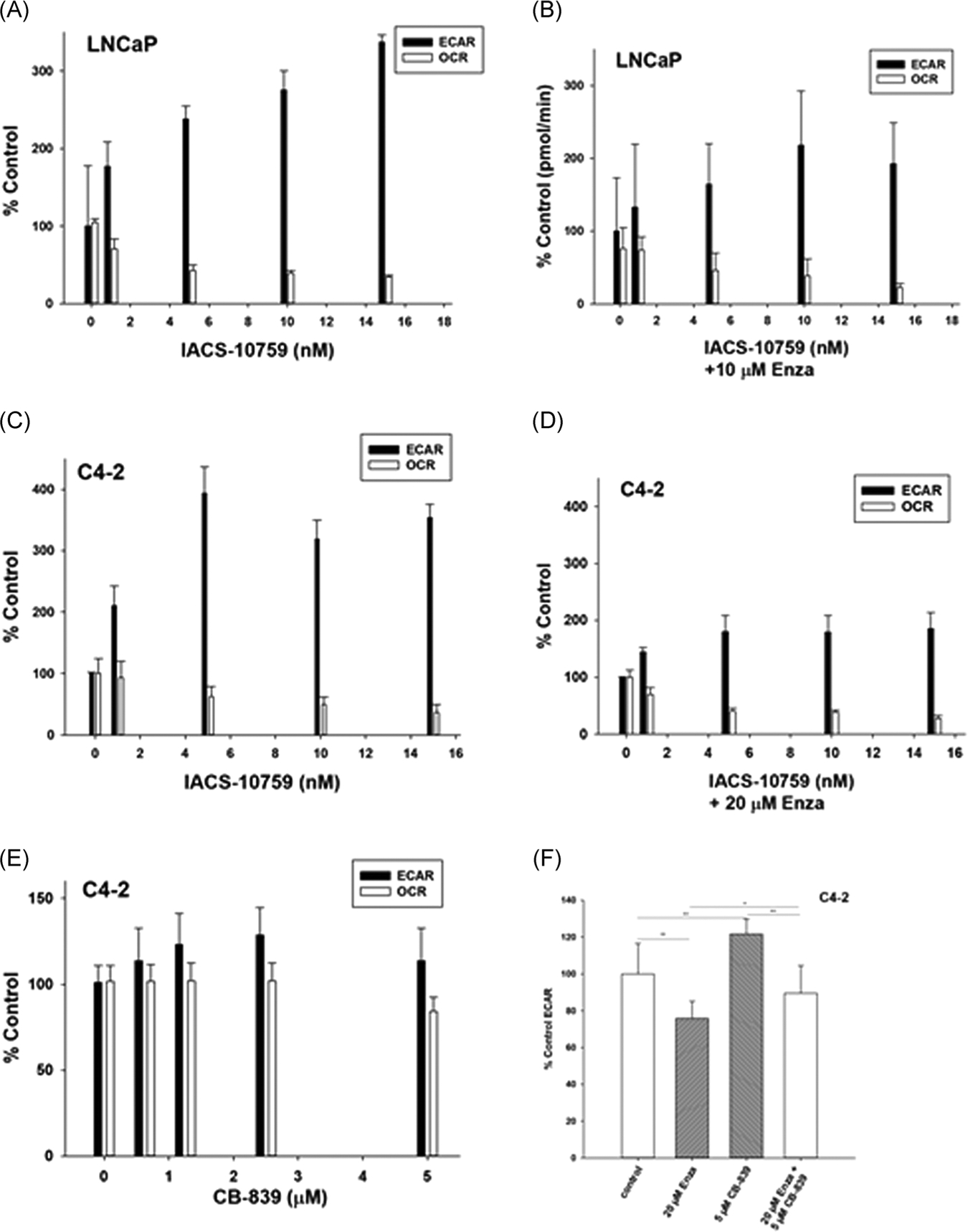
ECAR and OCR in (A) LNCaP, (C) C4–2 treated with graded concentration of IACS for 24 h and (E) C4–2 treated with graded concentration of CB-839 and (B), (D) and (F) are the corresponding treatment with IACS and CB-839 after 24 h pretreatment with ENZA. All ECAR and OCR data were normalized to viable cells as determined by propidium iodide staining (see text) at the end of the assay. Each data point and error bar represent the mean and standard deviation of readings from 15 separate wells repeated at least three times. *p < .005. ECAR, extracellular acidificationa rate; ENZA, enzalutamide; OCR, oxygen consumption rate
We anticipated that a decrease in mitochondrial ox-phos, when coupled with ENZA-induced reduction in glycolysis, should have a major reduction in cellular bioenergetics. This will enhance the growth inhibitory activity of the mitochondria targeted agents in ENZA pretreated cells. We tested the growth inhibitory effects of 72 h treatment with a graded concentration of IACS or CB-839 with or without 24 h ENZA pretreatmenton LNCaP and C4–2 and a single concentration of each of the agents on PCa2b cells. Concentrations of CB-839 were kept within the range of human serum Cmax and that of IACS is kept much below the Cmax reported in preclinical in vivo studies.17 ENZA was used at or below its published human serum Cmax.24 Cell growth data in terms of cellular total DNA fluorescence.18 are shown in Figure 4. The data clearly demonstrate that the growth inhibitory effects of both IACS and CB-839 are more pronounced in ENZA pretreated cells. The effect was more apparent in C4–2 cells than in LNCaP cells probably due to relatively more mitochondrial metabolism dependency of C4–2 cells treated with ENZA than that of LNCaP cells (see Figure 2). Similar increase in growth inhibitory effect of CB-839 was also observed in two other anti-androgen resistant cell lines CWR22ν1 and PCa2b pretreated with ENZA (data not shown).
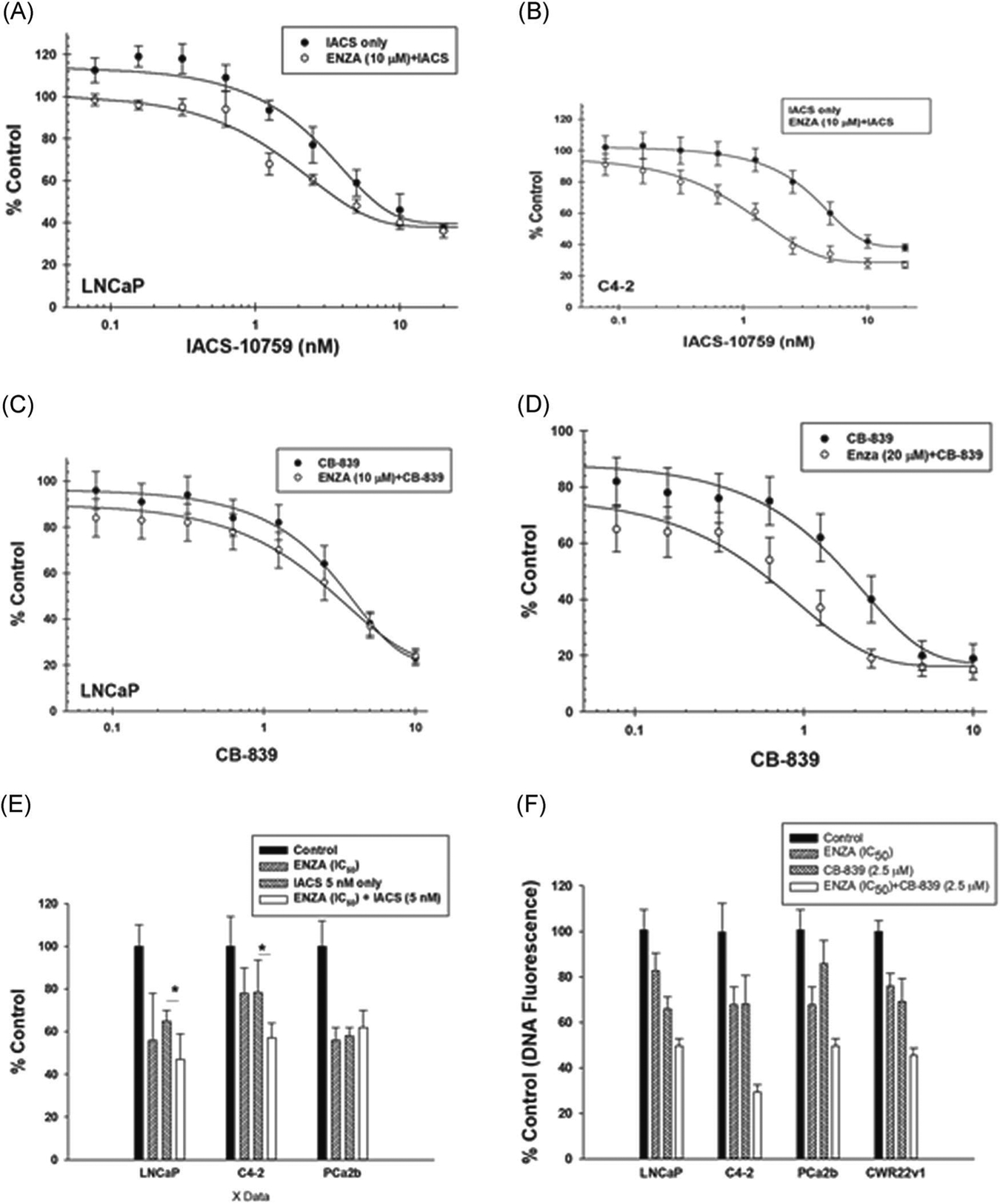
Effect of graded concentrations of IACS on the growth of (A, C) LNCaP and (B, D) C4–2 cells after 96 h treatment with IACS or CB-839 alone or after 24 h pretreatment with ENZA. (E) Effects of ENZA, IACS, and ENZA + IACS (F) and the effects of ENZA, CB-839 and ENZA + CB-839 on the growth of LNCaP, C4–2, and PDX-derived cells. Each data point and error bar represent the mean and standard deviation of readings from 18 separate wells repeated at least twice. *p < .005. ENZA, enzalutamide; PDX, patient-derived xenograft
3.5 |. Reduction of mitochondrial ROS is related to increased growth inhibition
We then used high resolution microscopy to observe changes in mitochondrial ROS as measured by mean pixel MSR dye fluorescnence intensities (see Figure 2) in LNCaP, C4–2, CWR22ν1 and PCa2b cells treated with CB-839 with or without ENZA pretreatment are shown in Figure 5. Mitochondrial superoxide production remains unchanged in ENZA-sensitive LNCaP cells, but significantly increase after ENZA treatment in most androgen-resistant cell lines. As observed from the Seahorse assay (Figure 3), CB-839 causes a decrease in mitochondrial metabolism in all cell lines. Significantly more reduction of mitochondrial superoxide production was observed for CB-839 treatement of ENZA pretreated cells. This effect is not seen in cells, when CB-839 is added either before or at the same time of ENZA addition (data not shown).
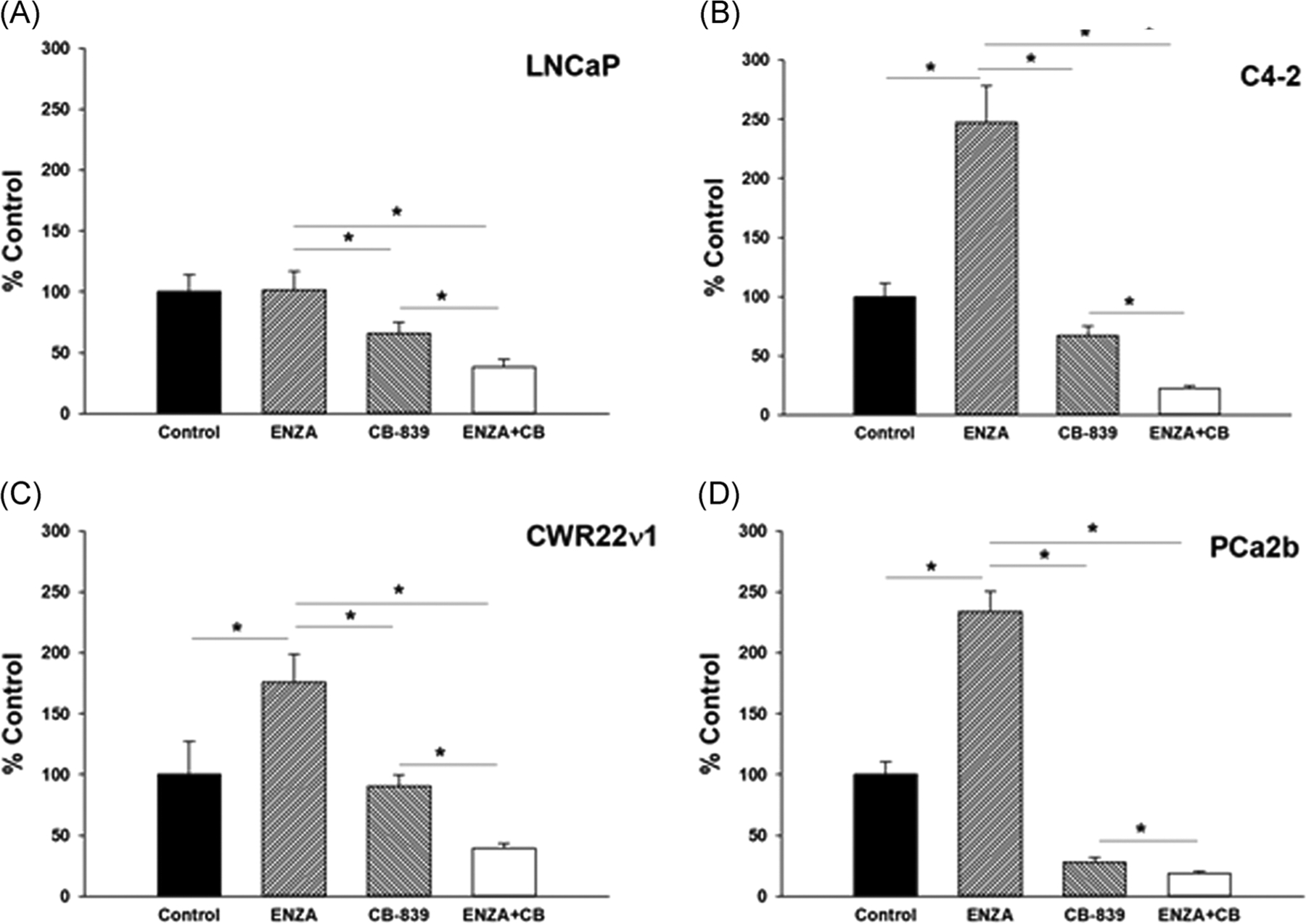
Mean pixel fluorescence intensities of oxidized MSR dye in the mitochondria were calculated from individual mitochondrion MSR fluorescence image quantitation and averaged over all mitochondria per cell as described in the text. (A) LNCaP, (B) C4–2, (C) CWR22ν1, and (D) PCa2b cells treated with vehicle control, 10 μM ENZA (LNCaP) and 20 μM ENZA (C4–2, CWR22ν1 and PCa2h) for 96 h, CB-839 for 72 h, and ENZA (96 h)+CB-839 (72 h) computed from microscopic image analysis (see text). *p < .005. CTC, circulating tumor cell; ENZA, enzalutamide; MSR, MitoSOX Red
3.6 |. Increase in ox-phos in CTCs from patients developing resistance to AR-targeted therapies
First, we have standardized a method of isolating CTCs from blood samples from consented PCa patients. PCa CTCs were identified as CD45 negative nucleated cells that are positive for AR and epithelial cell adhesion marker (EpCam). Representative images of the isolated CTCs stained for DNA (blue), AR (green) and EpCam (red) are shown in Figure 6A.
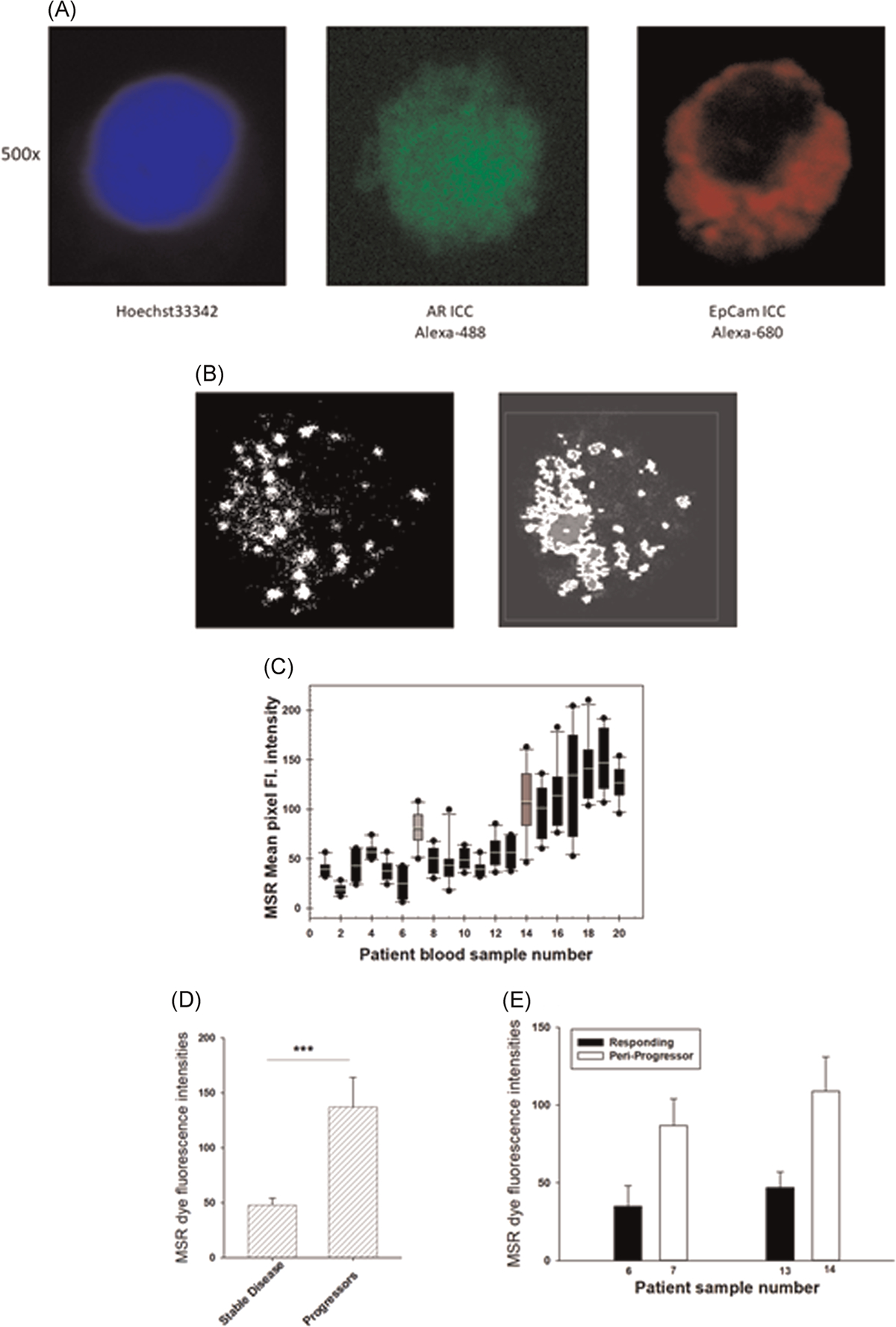
(A) CTC isolated from a PCa patient blood sample. The CTCs were identified from CD45 negative nucleated cells that are positive for androgen receptor and epithelial cell adhesion molecule as shown above the respective images; (B) a representative image of an optical section of MSR fluorescence due to oxidation by mitochondrial superoxide of a PCa patient CTC and (C) image segmentation analysis of the image in 5B for pixel fluorescence intensity analysis; (D) mean MSR dye fluorescence intensities of 10 or more CTCs obtained from each PCa patient undergoing androgen-signaling axis targeting therapies. Each sample was collected from an individual patient, except samples #6, #7 and #13, #14 (marked in gray). Samples marked with a line on top are from patients who developed resistance to anti-androgen therapy. (D) Mean CTC MSR dye fluorescence intensities in patients with stable and progressive disease, p value for linear mixed model (***p < .0001). (E) Mean CTC MSR dye fluorescence intensities for two patients while stable and near the time of progression, p value (**p < .005). CTC, circulating tumor cell; PCa, prostate cancer; MSR, MitoSOX Red
To quantitate mitochondrial superoxide production in the CTCs, we followed ex vivo MSR dye oxidation in the CTCs following the protocol standardized for cell lines in our laboratory (see Figure 2). A representative image of an optical section of a CTC isolated from a PCa patient blood sample and its corresponding segmentation and analysis are shown in Figures 6B and and6C,6C, respectively. The background correction and threshold are adjusted to set all fluorescence pixels within the nuclei below <5 fluorescence units to correct for the mitochondria that are not in each optical section (0.5 μm) that are being analyzed. The mean pixel intensities of MSR dye fluorescence in 5–6 optical sections per cell (approximately 1500–2000 mitochondria per cell) were determined from each CTC.
Thus far, we have collected 22 blood samples from 20 patients with CRPC (age range 52–73) undergoing AR-targeted therapies either ENZA or abiraterone in combination with androgen deprivation. The patients are considered responding to therapy during sample collection, when their prostate specific antigen (PSA) levels are stable and are under 4 ng/dl for two consecutive serum PSA tests within 6 months before and after sample collection. Patients with rising PSA within that period are considered developing resistance to therapy.
From each 10 ml blood sample, we detected sufficient (10 or more) analyzable CTCs from 18 out of 20 patients. Mean MSR dye fluorescence intensities of a minimum of 10 CTCs per patient sample are shown in Figure 5D. Samples #14–20 showed significant increase in MSR fluorescence intensities representing enhanced mitochondrial ETS activity as compared to samples #1–6 and #8–13. Patients contributing samples #15–20 have progressed to therapy resistance within 3 months after the sample collection. Samples #6 and #7 (from one patient) and #13 and #14 (from another patient) were collected longitudinally within a time span of 3 months for each individual. As shown in Figure 6E,,F,F, both samples #7 and #14 showed significantly higher MSR dye intensity as compared to sample #6 and #13, respectively. At the time of collection of samples #6 and #13, both patients were responding to treatment with androgen synthesis inhibitor abiraterone acetate plus ADT as concluded from their low serum PSA level. At the time of collection of sample #7, the patient had a stable PSA measurement, but the patient showed progressive disease within 3 months after collection of the sample #7 and could not be followed further for serum PSA or radiographic progression. At the time of collection of sample #14, the patient’s serum PSA increased and subsequent follow up showed steadily increasing PSA followed by radiographic progression of the disease.
4 |. DISCUSSION
While glycolysis is ~4 fold less in CRPC C4–2 cells relative to its parental ADPC LNCaP cells (Figure 1A), oxygen consumption in C4–2 cells is only about 1.5-fold more than that in LNCaP cells (Figure 1B). As ox-phos generates 18 times more ATP than does glycolysis, a relatively smaller increase in oxygen consupmtion can compensate for a larger decrease in glycolysis. It is noted that ENZA-treatment causes a marked decrease in glycolysis (Figure 1C) in ENZA-sensitive LNCaP cells relative to that in ENZA-resistant C4–2 cells (Figure 1D). The Seahorse data are supported by metabolite estimation data related to ENZA-treatment of LNCaP and C4–2 cells (Figure 1E). As C4–2 is much less glycolytic than is LNCaP (Figure 1A,,E),E), it is difficult to ascertain if the lack of ENZA-effect in C4–2 cells is related to its ENZA resistance or the lack of sensitivity of the assay in cells at a very low glycolytic state.
Mitochondrial ETS activity relates to ox-phos in most cells not treated with an uncoupling agent such as oligomycin. The ETS activity could be measured at a much higher accuracy in single cells using high resolution fluorescence microscopy of MSR dye fluorescence coupled with computer-aided image analysis as compared to Seahorse assay. A significant increase in MSR dye fluorescence in ENZA-treated cells shows that the relatively higher ETS activity is indeed related to ENZA-resistance in two -resistant cell lines C4–2 and CWR22ν1 as compared to -sensitive LNCaP cells. The single cell data are confirmed in a 96-well plate-based measurement of changes in MSR intensity per mitochondria (MSR fluorescence normalized to MTG fluorescence of total mitochondria per cell) in LNCaP and C4–2 cells. Unlike the LNCaP cells, the MSR fluorescence in the -resistant C4–2 cells significantly increases with ENZA treatment. This effect is more pronounced for IACS than for CB-839 probably because IACS directly inhibits ox-phos by blocking the mitochondrial ETS activity, while CB-839 only indirectly affects ox-phos by blocking the supply of α-KG, which is one of several metabolites that feed the TCA cycle to generate electrons for the ETS.
Thus, we have shown that CRPC cells in general, are less glycolytic and use more mitochondrial ETS than their androgen dependent counterparts. All PCa cells surviving in the presence of anti-androgens further increase their dependence on mitochondrial ETS activity. Mitochondrial metabolism targeted inhibitors currently under preclinical and clinical development decreases ox-phos. Thus, we hypothesize that CRPC cells surviving in the presence of anti-androgens will be more vulnerable to mitochondrial metabolism targeting agent.
As anticipated, the mitochondria-targeted metabolic inhibitors strongly suppress mitochondrial ETS activity. ENZA pretreatment further decreases mitochondrial activity (Figure 5) and increases growth inhibitory effect of CB-839 (Figure 4E,,F).F). Interestingly, this effect is not observed, when ENZA was added either with or after CB-839 treatment (data not shown). We hypothesize that the ENZA induced increase in mitochondrial ETS activity in androgen-resistant cells happens due to changes in cellular metabolism either at or upstream of the ETS (site of action of IACS) or glutaminase activity (target of CB-839). Therefore, ENZA cannot further increase the mitochondrial ROS in any of the cells that are either pretreated or co-treated with the metabolic inhibitors. In fact, ENZA pretreatment further decreases mitochondrial ETS activity in CB-839 treated cells. This suggests that ENZA-treated cells become more dependent on mitochondrial metabolism and reduce other cellular metabolic activities upstream to mitochondrial metabolism such as glycolytic and/ or lipid biosynthetic pathways that may indirectly contribute to mitochondrial ETS activity.
High resolution microscopic image analysis of CTCs showed a significantly more mitochondrial ETS activities during or just before the development of resistance to anti-androgen therapy in all patients studied thus far.
5 |. CONCLUSION
ENZA treatment makes PCa cells more vulnerable to metabolic inhibitors that are under clinical development. The high resolution microscopy method may be developed further for clinical application to identify the disease state in patients, when they will best respond to mitochondrial metabolism and ETS inhibitors.
ACKNOWLEDGMENTS
We thank MD Anderson Genitourinary Oncology clinical research group and all patients who participated in this study and their families for their help and support. We also thank Joe Marszalek, Inst Head, CCCT Transl Biology, MDACC for the supply of IACS-010759. The study was supported by the National Institute of Health: R01 CA185251–02, DoD CDMRP: W81XWH-20–1-0306 and W81XWH-15–1-0509, MD Anderson Cancer Center moonshot program, and the Department of Genitourinary oncology, MDACC.
Funding information
Congressionally Directed Medical Research Programs, Grant/Award Numbers: W81XWH- 15–1-0509, W81XWH2010306; National Cancer Institute, Grant/Award Number: 5 R01 CA185251–02
Footnotes
CONFLICT OF INTERESTS
All Authors declare no potential conflict of interest with this publication.
DATA AVAILABILITY STATEMENT
The data that support the findings of this study are available from the corresponding author upon reasonable request.
REFERENCES
Citations & impact
Impact metrics
Citations of article over time
Alternative metrics

Discover the attention surrounding your research
https://www.altmetric.com/details/108196119
Smart citations by scite.ai
Explore citation contexts and check if this article has been
supported or disputed.
https://scite.ai/reports/10.1002/pros.24146
Article citations
Identification of prostate cancer bone metastasis related genes and potential therapy targets by bioinformatics and in vitro experiments.
J Cell Mol Med, 28(15):e18511, 01 Aug 2024
Cited by: 1 article | PMID: 39098992 | PMCID: PMC11298316
MED12 and CDK8/19 Modulate Androgen Receptor Activity and Enzalutamide Response in Prostate Cancer.
Endocrinology, 165(10):bqae114, 01 Aug 2024
Cited by: 0 articles | PMID: 39253786 | PMCID: PMC11398899
The polyunsaturated fatty acid docosahexaenoic affects mitochondrial function in prostate cancer cells.
Cancer Metab, 12(1):24, 07 Aug 2024
Cited by: 0 articles | PMID: 39113152 | PMCID: PMC11308158
Tracking down metabolic vulnerabilities in CDK12-mutant prostate cancer.
Clin Transl Discov, 4(4):e345, 19 Jul 2024
Cited by: 0 articles | PMID: 39183936
Enzalutamide Sensitizes Castration-Resistant Prostate Cancer to Copper-Mediated Cell Death.
Adv Sci (Weinh), 11(30):e2401396, 10 Jun 2024
Cited by: 1 article | PMID: 38859590 | PMCID: PMC11321675
Go to all (16) article citations
Similar Articles
To arrive at the top five similar articles we use a word-weighted algorithm to compare words from the Title and Abstract of each citation.
Enhanced radiosensitization of enzalutamide via schedule dependent administration to androgen-sensitive prostate cancer cells.
Prostate, 78(1):64-75, 14 Nov 2017
Cited by: 33 articles | PMID: 29134684
Influence of abiraterone and enzalutamide on body composition in patients with metastatic castration resistant prostate cancer.
Cancer Treat Res Commun, 25:100256, 01 Dec 2020
Cited by: 10 articles | PMID: 33307509
Isolation and characterization of castration-resistant prostate cancer LNCaP95 clones.
Hum Cell, 34(1):211-218, 20 Sep 2020
Cited by: 9 articles | PMID: 32954481 | PMCID: PMC8693726
Persistent androgen receptor addiction in castration-resistant prostate cancer.
J Hematol Oncol, 8:128, 13 Nov 2015
Cited by: 46 articles | PMID: 26566796 | PMCID: PMC4644296
Review Free full text in Europe PMC
Funding
Funders who supported this work.
Congressionally Directed Medical Research Programs (2)
Grant ID: W81XWH2010306
Grant ID: W81XWH‐15‐1‐0509
NCI NIH HHS (1)
Grant ID: R01 CA185251