Abstract
Free full text

A Live, Attenuated Dengue Virus Type 1 Vaccine Candidate with a 30-Nucleotide Deletion in the 3′ Untranslated Region Is Highly Attenuated and Immunogenic in Monkeys
Abstract
The Δ30 deletion mutation, which was originally created in dengue virus type 4 (DEN4) by the removal of nucleotides 172 to 143 from the 3′ untranslated region (3′ UTR), was introduced into a homologous region of wild-type (wt) dengue virus type 1 (DEN1). The resulting virus, rDEN1Δ30, was attenuated in rhesus monkeys to a level similar to that of the rDEN4Δ30 vaccine candidate. rDEN1Δ30 was more attenuated in rhesus monkeys than the previously described vaccine candidate, rDEN1mutF, which also contains mutations in the 3′ UTR, and both vaccines were highly protective against challenge with wt DEN1. Both rDEN1Δ30 and rDEN1mutF were also attenuated in HuH-7-SCID mice. However, neither rDEN1Δ30 nor rDEN1mutF showed restricted replication following intrathoracic inoculation in the mosquito Toxorhynchites splendens. The ability of the Δ30 mutation to attenuate both DEN1 and DEN4 viruses suggests that a tetravalent DEN vaccine could be generated by introduction of the Δ30 mutation into wt DEN viruses belonging to each of the four serotypes.
There are four serotypes of dengue virus (dengue virus type 1 [DEN1], DEN2, DEN3, and DEN4) that annually cause an estimated 50 to 100 million cases of dengue fever and 500,000 cases of the more severe form of dengue virus infection known as dengue hemorrhagic fever/dengue shock syndrome (6). Dengue virus is widely distributed throughout the tropical and semitropical regions of the world, and the number of dengue virus infections continues to increase due to the expanding range of its Aedes aegypti mosquito vector. A vaccine is not available for the control of dengue disease despite its importance as a reemerging disease. The goal of immunization is to protect against dengue virus disease by the induction of a long-lived neutralizing antibody response against each of the four serotypes. Simultaneous protection against all four serotypes is required, since an increase in disease severity can occur in persons with preexisting antibodies to a heterotypic dengue virus. Such immunization can be achieved economically with a live, attenuated virus vaccine.
Dengue viruses are positive-sense RNA viruses belonging to the Flavivirus genus. The approximately 11,000-base genome contains a single open reading frame encoding a polyprotein which is processed by proteases of both viral and cellular origin into three structural proteins (C, prM, and E) and at least seven nonstructural (NS) proteins. Both ends of the dengue virus genome contain an untranslated region (UTR), and the overall genome organization is 5′-UTR-C-prM-E-NS1-NS2A-NS2B-NS3-NS4A-NS4B-NS5-UTR-3′. The 3′ UTR is nearly 400 bases in length and is predicted to contain several stem-loop structures conserved among dengue virus serotypes (3, 9, 14, 17). One such stem-loop structure, identified as TL2 in the proposed secondary structure of the 3′ UTR (14), was previously removed by deletion of 30 nucleotides from the DEN4 genome (3′ nucleotides 172 to 143) (12) and has subsequently been designated as the Δ30 mutation (5). The resulting virus, rDEN4Δ30, was shown to be attenuated in rhesus monkeys compared to parental viruses containing an intact TL2 sequence (5). In addition, the Δ30 mutation was shown to restrict the capacity for dissemination of DEN4 virus from the midgut to the head of mosquitoes (20). As a vaccine candidate, rDEN4Δ30 (also referred to as 2AΔ30) was administered to 20 adult human volunteers and shown to be highly immunogenic and well tolerated without causing systemic illness (5).
Based on the success of this vaccine candidate, a strategy for the development of additional vaccine candidates representing the other three DEN virus serotypes was foreseen in which wild-type (wt) dengue viruses could be similarly attenuated for vaccine use by incorporation of mutations in the 3′ UTR. As a first step, we introduced the Δ30 mutation into the homologous region of the 3′ UTR of DEN1 virus and evaluated the level of replication of the resulting virus in rhesus monkeys and mosquitoes. Although the individual nucleotides are not well conserved in the TL2 region of each of the four DEN virus serotypes, appropriate base pairing preserves the stem-loop structure for DEN1 and DEN4 (Fig. (Fig.1A).1A). The use of wt DEN1 virus as the parent for the introduction of the Δ30 mutation also permitted a comparison of the level of attenuation of rDEN1Δ30 with that of the previously described rDEN1mutF virus, which also contains mutations in the 3′ UTR (11). The mutF mutation consists of a pair of deleted nucleotides and a two-nucleotide substitution in the terminal 3′ stem-loop structure conserved among all flavivirus species (22).
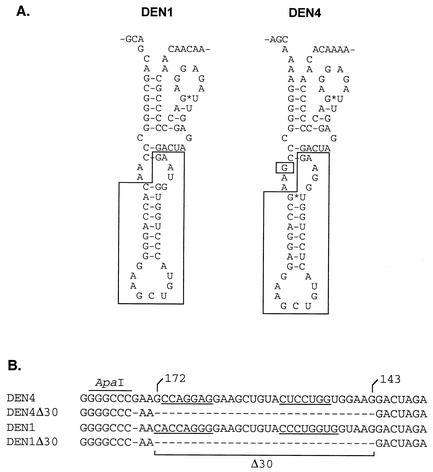
The Δ30 mutation removes 30 contiguous nucleotides from the 3′ UTR of DEN4. (A) Predicted secondary structure of the TL2 region of DEN1 and DEN4 (15). Nucleotides that are removed by the Δ30 mutation are boxed. (B) Nucleotide sequence alignment of the TL2 region of DEN4 and DEN1 and their Δ30 derivatives. Nucleotides of DEN4 are numbered starting at the 3′ terminus of the genome. Underlining indicates nucleotide pairing to form the predicted stem structure.
To introduce the Δ30 mutation into a DEN virus other than DEN4, the DEN1 Western Pacific (WP) strain was engineered to contain the mutation. The DEN1 cDNA clone, pRS424DEN1WP (16), was used as the template in PCR to generate a 292-nucleotide fragment designed to remove 30 nucleotides as shown in Fig. Fig.1B.1B. The original pRS424DEN1WP cDNA clone was digested with ApaI (cleaves just upstream of the Δ30 mutation) and SacII (cleaves downstream of the 3′ end), mixed with the PCR product, and used to transform yeast strain YPH857 as described previously (13). DNA isolated from two independent yeast colonies was used to transform Escherichia coli strain STBL2 (Invitrogen, Carlsbad, Calif.). Plasmid DNA suitable for generating RNA transcripts was prepared, and the presence of the Δ30 mutation was verified by sequence analysis.
For transcription and generation of virus, pRS424DEN1Δ30 was linearized with SacII and used as the template in a transcription reaction with SP6 RNA polymerase as previously described (13). Transcripts were electroporated into LLC-MK2 cells, the generation of virus was confirmed by observation of cytopathic effects and immunofluorescence, and the cultures were harvested on day 14. The recovered recombinant virus was terminally diluted twice and amplified in Vero cells. The genome of the resulting virus, rDEN1Δ30, was sequenced to confirm the presence of the Δ30 mutation and the absence of adventitious mutations. wt parental virus rDEN1 was similarly derived from pRS424DEN1WP cDNA and served as control virus for the animal studies. The DEN1mutF full-length cDNA clone was constructed as previously described (11), and virus was initially recovered from transfected Vero cells. Virus stocks were terminally diluted twice and amplified in Vero cells. The genome of the resulting virus, rDEN1mutF, was sequenced to confirm the presence of the mutF mutation. Incidental mutations arising from virus passage in tissue culture were identified in all viruses by sequence analysis and are listed in Table Table11.
TABLE 1.
Incidental mutations observed among the recombinant DEN1 viruses
Virusa | Gene | Nucleotideb | Amino acidc |
---|---|---|---|
wt rDEN1 | prM | C816U | A240V |
wt rDEN1 | NS4B | U7165G | F2357L |
wt rDEN1 | NS4B | U7173C | V2360A |
rDEN1mutF | prM | C612U | T173M |
rDEN1Δ30 | E | A1748U | T552S |
The replication, immunogenicity, and protective efficacy of rDEN1Δ30 were compared to those of wt parental rDEN1 virus and rDEN1mutF in juvenile rhesus monkeys. Dengue virus-seronegative monkeys were injected subcutaneously with 5.0 log10 PFU of virus. Monkeys were observed daily, and blood was collected on days 0 to 10 and 28 and serum was stored at −70°C. Titers of virus in serum samples were determined by plaque assays in Vero cells as described previously (5). Plaque reduction neutralization titers against wt rDEN1 were determined for the day 28 serum samples by using methods previously described (5). All monkeys were challenged subcutaneously on day 28 with 5.0 log10 PFU of wt rDEN1, and blood was collected for 10 days. Virus titers in postchallenge sera were determined by plaque assays in Vero cells. Monkeys inoculated with wt rDEN1 or rDEN1mutF were viremic for 2 to 3 days, with mean peak titers of 2.1 and 1.4 log10 PFU/ml, respectively (Table (Table2;2; Fig. Fig.2).2). Monkeys inoculated with rDEN1Δ30 were viremic for less than 1 day, with a mean peak titer of 0.8 log10 PFU/ml, a level of replication significantly lower than that of wt rDEN1 (Tukey-Kramer test; P < 0.05), indicating that the Δ30 mutation is capable of attenuating DEN1. Although monkeys inoculated with rDEN1mutF showed a decreased level of viremia compared to those inoculated with wt rDEN1, this difference was not statistically significant. Previously published results for studies with rhesus monkeys have shown a similar level of attenuation for rDEN1mutF (11). In addition, Markoff et al. have shown that there was a difference in the mean number of days of viremia (determined by reverse transcription-PCR) between monkeys that received DEN1mutF virus and those that received wt DEN1 (11). No such difference was observed in the present study.

(A) Viremia in monkeys inoculated with DEN1 vaccine candidates. Groups of four rhesus monkeys were inoculated subcutaneously with 5.0 log10 PFU of the indicated virus in a 1-ml dose. Serum was collected daily, and virus titers were determined by plaque assays in Vero cells. Mean virus titers are shown for monkeys in each group. The lower level of virus detection was 0.7 log10 PFU/ml. (B) For comparison, viremia levels in monkeys inoculated previously with vaccine candidate rDEN4Δ30 (6) are shown. Groups of four monkeys were inoculated in a manner identical to that described above.
TABLE 2.
The Δ30 and mutF mutations attenuate rDEN1 for rhesus monkeys and HuH-7-SCID mice
Virus | Replication in rhesus monkeysa
| Geometric mean serum-neutralizing antibody titer (reciprocal dilution)d
| Replication in HuH-7-SCID micee
| |||||||
---|---|---|---|---|---|---|---|---|---|---|
No. of monkeys | No. of monkeys with viremia
| Mean no. of viremic days per monkeyb | Mean peak, virus titerc (log10 PFU/ml ± SE) | No. of mice | Mean peak virus titerf (log10 PFU/ml ± SE) | |||||
Vaccination | Challenge | Day 0 | Day 28 | Day 56 | ||||||
wt rDEN1 | 4 | 4 | 0 | 2.8 | 2.1 ± 0.1 | <10 | 1,230 | 1,375 | 9 | 7.3 ± 0.2 |
rDEN1mutF | 4 | 4 | 0 | 2.5 | 1.4 ± 0.3 | <10 | 1,486 | 948 | 7 | 5.3 ± 0.3 |
rDEN1Δ30 | 4 | 2 | 0 | 0.5 | 0.8 ± 0.1 | <10 | 780 | 1,332 | 8 | 5.0 ± 0.3 |
The neutralizing antibody titer was lower in monkeys inoculated with rDEN1Δ30 than in animals inoculated with either wt rDEN1 or rDEN1mutF (Table (Table2).2). This lower immunogenicity of rDEN1Δ30 was consistent with its greater level of attenuation. Importantly, the immunogenicity of rDEN1Δ30 and rDEN1mutF was sufficient to protect the animals against wt rDEN1 virus challenge. wt rDEN1 virus was not detected in any serum sample collected following virus challenge, and no monkey achieved an increase greater than threefold in the level of serum-neutralizing antibody following virus challenge, indicating that monkeys were completely protected following immunization with either wt rDEN1 or vaccine candidates rDEN1Δ30 and rDEN1mutF. The level of attenuation specified by the Δ30 mutation is comparable in both the DEN1 and DEN4 genetic backgrounds (Fig. (Fig.2).2). However, because vaccine candidate rDEN1Δ30 also contains a missense mutation in the E gene region (Table (Table1),1), it is impossible at this time to formally attribute the observed attenuation phenotype solely to the Δ30 mutation. Future studies designed to genetically isolate the Δ30 mutation from background incidental mutations by its introduction into additional vaccine candidates will be necessary to further define its phenotype.
A rodent model for the evaluation of attenuated strains of DEN virus has recently been described (1, 2) and consists of SCID mice bearing intraperitoneal tumors of the human liver cell line HuH-7. Following inoculation of DEN virus directly into the tumor, virus can be detected in the serum. As previously reported, recombinant DEN4 virus replicated to greater than 6.0 log10 PFU/ml of serum in these mice, while the replication of DEN4 virus bearing the Δ30 mutation was reduced by about 10-fold (2). The replication of rDEN1Δ30 was compared to that of wt rDEN1 virus and rDEN1mutF in HuH-7-SCID mice (Table (Table2).2). Both rDEN1Δ30 and rDEN1mutF replicated to a level approximately 100-fold less than that of their wt rDEN1 parent. These results further validate the use of the HuH-7-SCID mouse model for the evaluation of attenuated strains of DEN virus, with results correlating closely with those observed with rhesus monkeys.
The DEN4 vaccine candidate bearing the Δ30 mutation was previously shown not to be transmitted to Aedes albopictus mosquitoes fed on 10 vaccinees, all of whom were infected with the vaccine strain (20). Because this lack of transmissibility was attributed to both the low level of viremia in vaccinees and to the restricted capacity of rDEN4Δ30 to disseminate from the midgut of the mosquito to its head, a phenotype previously shown to be specified by the Δ30 mutation (20), it was important to evaluate the ability of rDEN1Δ30, rDEN1mutF, and wt virus to infect and replicate in mosquitoes. This was studied by determining the mosquito 50% infectious dose (MID50) of each virus following oral feeding or intrathoracic inoculation.
Maintenance, infection, and detection of viral antigen in A. aegypti, A. albopictus, and Toxorhynchites splendens were conducted as previously described (20). Briefly, A. aegypti and A. albopictus were fed a blood meal containing serial dilutions of virus suspension, maintained for 21 days, and frozen. Head and midgut preparations were processed by immunofluorescence assays (IFA) using mouse monoclonal antibody 4G2 derived from hybridoma HB112 (American Type Culture Collection, Manassas, Va.), which is specific for flavivirus group-specific antigens. T. splendens mosquitoes were inoculated intrathoracically with a 0.2-μl dose containing serial 10-fold dilutions of virus in PBS, and head preparations were processed for IFA as described above. The wt rDEN1 and mutant viruses showed low infectivity (MID50s of >4.0 log10 PFU/ml and >3.0 log10 PFU/ml, respectively) by oral feeding of A. aegypti and A. albopictus, precluding meaningful comparison of the infectivity of these viruses by this route (data not shown). In previously published studies (18), DEN1 viruses showed very low infectivity for A. aegypti fed an infectious blood meal and a relatively higher level of infectivity for A. albopictus which was nonetheless low compared to those of other DEN serotypes. In contrast, each of the recombinant viruses was highly infectious for T. splendens following intrathoracic inoculation, with MID50 values approaching 1 for each of the viruses tested (Table (Table3).3). The MID50 titers for the three viruses after infection of T. splendens were not significantly different (logistic likelihood ratio test; df = 2, P = 0.12, where df is degrees of freedom). This finding is consistent with previous observations that DEN1 strains are highly infectious by intrathoracic inoculation of T. splendens (18, 19) and that the Δ30 mutation does not restrict infection of DEN4 virus given intrathoracically to T. splendens (20). Although the mutF mutation has previously been associated with reduced replication in C6/36 cells (11), it had no detectable effect on the infectivity of DEN1 by intrathoracic inoculation.
TABLE 3.
The Δ30 and mutF mutations do not decrease infectivity of rDEN1 virus inoculated intrathoracically into T. splendens
Virus | Dosea (log10 PFU) | No. tested | No. (%) infectedb |
---|---|---|---|
wt rDEN1 | 3.5 | 7 | 7 (100) |
2.5 | 8 | 6 (75) | |
1.5 | 7 | 5 (71) | |
0.5 | 5 | 3 (60) | |
rDEN1mutF | 2.3 | 8 | 7 (88) |
1.3 | 5 | 4 (80) | |
0.3 | 8 | 7 (88) | |
rDEN1Δ30 | 2.7 | 8 | 8 (100) |
1.7 | 7 | 7 (100) | |
0.7 | 6 | 5 (83) |
The demonstration that the Δ30 mutation attenuates both DEN1 and DEN4 viruses for rhesus monkeys to a comparable degree, yet preserves immunogenicity, has important implications for development of a tetravalent vaccine. It seems likely that the Δ30 mutation will have a corresponding effect on DEN2 and DEN3 wt viruses, and such recombinant viruses are currently being generated. The strategy of introducing the Δ30 mutation into wt DEN viruses of each serotype to generate a suitably attenuated tetravalent vaccine formulation is a novel and attractive approach for several reasons. First, the mutation responsible for attenuation is a 30-nucleotide deletion in the 3′ UTR, thus assuring that all of the structural and NS proteins expressed by each of the four components of the tetravalent vaccine candidate are authentic wt proteins. Such wt proteins should elicit antibody and cellular immune responses that are broadly based rather than based solely on the M and E proteins that are present in chimeric dengue virus vaccine candidates (8, 10). The novelty of this approach derives from the fact that other live, attenuated dengue virus vaccines have attenuating mutations in their structural or NS proteins (4, 15); therefore, the immune response induced by these viruses will be to a mutant protein rather than to a wt protein. Second, deletion mutations are genetically more stable than point mutations and reversion of the attenuation phenotype is unlikely. In humans, DEN4Δ30 present in serum of vaccinees retained its Δ30 mutation, confirming its genetic stability in vivo (5). The attenuating mutations in other existing dengue live, attenuated vaccine candidates are based on less-stable point mutations (4, 15). Third, since the Δ30 mutation is common to all of the four viruses of the tetravalent vaccine, recombination between the any of the four vaccine serotypes would not lead to loss of the attenuating mutation or reversion to a wt phenotype. Recombination between components of the trivalent polio vaccine has been observed (7), and naturally occurring recombinant dengue viruses have been described (21), indicating the ability of this flavivirus to exchange genetic elements between two different viruses. Fourth, viruses with wt structural proteins appear more infectious than viruses with altered structural proteins (10). This could potentially decrease the quantity of each of the four virus components in the final vaccine, contributing to a lower cost of manufacture. This is a very important consideration for any dengue virus vaccine, since the virus is widely distributed throughout many developing countries in the tropical and semitropical regions of the world.
Acknowledgments
The authors thank the following individuals for expert technical assistance: Christopher T. Hanson and Cai-Yen Firestone (NIAID, Bethesda, Md.), Stephanie Polo (FDA, Bethesda, Md.), Lara Gilmore (WRAIR, Silver Spring, Md.), and Marisa St. Claire and Tammy Tobery (BIOQUAL, Rockville, Md.).
REFERENCES
Articles from Journal of Virology are provided here courtesy of American Society for Microbiology (ASM)
Full text links
Read article at publisher's site: https://doi.org/10.1128/jvi.77.2.1653-1657.2003
Read article for free, from open access legal sources, via Unpaywall:
https://europepmc.org/articles/pmc140839?pdf=render
Citations & impact
Impact metrics
Citations of article over time
Alternative metrics
Smart citations by scite.ai
Explore citation contexts and check if this article has been
supported or disputed.
https://scite.ai/reports/10.1128/jvi.77.2.1653-1657.2003
Article citations
A safer cell-based yellow fever live attenuated vaccine protects mice against YFV infection.
iScience, 27(10):110972, 17 Sep 2024
Cited by: 0 articles | PMID: 39398246 | PMCID: PMC11470684
The N and C-terminal deleted variant of the dengue virus NS1 protein is a potential candidate for dengue vaccine development.
Sci Rep, 14(1):18883, 14 Aug 2024
Cited by: 0 articles | PMID: 39143088 | PMCID: PMC11324946
Subgenomic flavivirus RNA as key target for live-attenuated vaccine development.
J Virol, 98(7):e0010023, 29 May 2024
Cited by: 1 article | PMID: 38808973
Review
Neutralizing antibodies from prior exposure to dengue virus negatively correlate with viremia on re-infection.
Commun Med (Lond), 3(1):148, 19 Oct 2023
Cited by: 0 articles | PMID: 37857747 | PMCID: PMC10587183
NS1 Protein N-Linked Glycosylation Site Affects the Virulence and Pathogenesis of Dengue Virus.
Vaccines (Basel), 11(5):959, 08 May 2023
Cited by: 2 articles | PMID: 37243063 | PMCID: PMC10221952
Go to all (89) article citations
Data
Data behind the article
This data has been text mined from the article, or deposited into data resources.
BioStudies: supplemental material and supporting data
Nucleotide Sequences (3)
- (1 citation) ENA - AY145123
- (1 citation) ENA - AY145121
- (1 citation) ENA - AY145122
Similar Articles
To arrive at the top five similar articles we use a word-weighted algorithm to compare words from the Title and Abstract of each citation.
Recombinant, live-attenuated tetravalent dengue virus vaccine formulations induce a balanced, broad, and protective neutralizing antibody response against each of the four serotypes in rhesus monkeys.
J Virol, 79(9):5516-5528, 01 May 2005
Cited by: 97 articles | PMID: 15827166 | PMCID: PMC1082773
Introduction of mutations into the non-structural genes or 3' untranslated region of an attenuated dengue virus type 4 vaccine candidate further decreases replication in rhesus monkeys while retaining protective immunity.
Vaccine, 22(25-26):3440-3448, 01 Sep 2004
Cited by: 31 articles | PMID: 15308370
Vaccine candidates for dengue virus type 1 (DEN1) generated by replacement of the structural genes of rDEN4 and rDEN4Delta30 with those of DEN1.
Virol J, 4:23, 28 Feb 2007
Cited by: 21 articles | PMID: 17328799 | PMCID: PMC1819370
Development of a live attenuated dengue virus vaccine using reverse genetics.
Viral Immunol, 19(1):10-32, 01 Jan 2006
Cited by: 62 articles | PMID: 16553547
Review