Abstract
Free full text

Surface gas-exchange processes of snow algae
Abstract
The red-colored chlorophyte Chlamydomonas nivalis is commonly found in summer snowfields. We used a modified Li-Cor gas-exchange system to investigate surface gas-exchange characteristics of snow colonized by this alga, finding rates of CO2 uptake up to 0.3 μmol m−2s−1 in dense algal blooms. Experiments varying the irradiance resulted in light curves that resembled those of the leaves of higher plants. Red light was more effective than white and much more effective than green or blue, because of the red astaxanthin that surrounds and masks the algal chloroplasts. Integrating daily course measurements of gas exchange showed CO2 uptake around 2,300 μmol
m−2
day−1 in heavily colonized patches, indicating that summer snowfields can be surprisingly productive.
In high-altitude and high-latitude regions where winter snow persists into the local summer, one can almost always find patches of snow colored in various ways, but particularly red or pink. In the U.S., such snow is often called “watermelon snow” because of its pink color and also because it sometimes exudes a faint odor of ripe watermelon when disturbed. The organism responsible for the pink color (and presumably the odor) is Chlamydomonas nivalis (Bauer) Wille, a unicellular alga in the Volvocales. C. nivalis is apparently global, as it has been reported from New Zealand (1), Australia (2), and Europe (3–6). In North America, it occurs in Alaska (5), the Cascade Mountains of the Pacific Northwest (7, 8), the Sierra Nevada of California (7, 9), and the Rocky Mountains of the western United States and Canada (7, 10, 11).
The life-cycle stage most abundant in snow comprises spherical cells 10–50 μm in diameter (2, 9, 12, 13) that appear bright red under the microscope, a color that comes from the carotenoid astaxanthin (14, 15). These spherical cells are called “aplanospores” and are designated “resting cells” in the algal literature, although this does not mean that they are metabolically inactive. The cells are not motile and drift passively with the meltwater as it flows along and through the snow (16), although this movement may be somewhat restricted by a mucilaginous coat that causes them to stick slightly to snow crystals (4).§ Snowfields typically show a very patchy distribution of pink color, with more intense pink often concentrated along meltwater courses.
The environment in which C. nivalis lives is a harsh one for photosynthetic organisms. At the high altitudes where they are typically found (above 2,500 m), UV-radiation levels can be quite high (17), and clear skies coupled with reflection within the snow can result in spherically integrated photosynthetically active radiation (PAR) fluence rates that often reach 4,500 μmolm−2
s−1 and occasionally reach 6,000 μmol
m−2
s−1 (18), about triple the maximum light load on a horizontal leaf at the top of a canopy. Moreover, the algae live in snow that is never much warmer than 0°C, and cold temperatures exacerbate photoinhibition (19, 20). Nonetheless, the cells are clearly photosynthetic. Thomas (9) measured photosynthesis by using 14C near Tioga Pass, California, finding rates from 6 to 34 μg C mm−3
hr−1 (≈6.5 × 10−11 μmol CO2
s−1 per cell). Thomas also reported that subsurface samples, which received less light, photosynthesized more than samples from the surface, suggesting that photoinhibition might have occurred in the surface layers. Mosser et al. (10), however, reported no photoinhibition up to 8.5 × 104 lux (≈1,600 μmol
m−2
s−1). Mosser et al. (10) also studied the effects of temperature on 14CO2 uptake, finding that most populations had temperature optima between 10°C and 20°C. (They reported their CO2-uptake rates in raw cpm.)
Although 14CO2 methods can provide information useful to the study of the alga itself, it is also important to know how much CO2 exchange takes place between the snow and the air. Most investigators who have studied carbon exchange between snow and air have made their measurements in winter, when they generally find that CO2 flows out of the snow because of soil respiration under it (21–23); photosynthesis in snow algae could absorb CO2 from the air, or the algae could simply tap into this efflux, reducing CO2 emissions from the snow. Gas-exchange from snow-algae-covered areas might represent a small but significant global carbon sink not previously noticed, because summer snowfields cover significant areas of the Earth. Regardless of the source of CO2, algal photosynthesis could play an important role in carbon and nutrient cycling in the local ecosystem of the snowfields and their surroundings. Therefore, we decided to examine the gas-exchange characteristics of pink snow by using a modified gas-exchange apparatus that is normally used to measure leaf-level photosynthesis.
Materials and Methods
We used a Li-Cor LI-6200 photosynthesis system (Li-Cor, Lincoln, NE) as the basis of our snow-surface gas-exchange system. Because no commercially available chamber met our purposes, we constructed a shallow square Plexiglas chamber, ≈1 m square by 8 cm high, and attached the LI-6200 sensor head (containing humidity and temperature sensors and hose connections to the rest of the LI-6200 system). We installed two brushless motor fans (F62LM-012GK, Micronel, Vista, CA) to promote good gas mixing within the chamber. Recently, Hooper et al. (24) pointed out that using the LI-6200 with large chambers can lead to calculation errors caused by (i) changes in chamber water-vapor pressure and (ii) mixing in a preexisting boundary layer that might have a very different [CO2] from that of the bulk air. Because the snow is so cold, evaporation from the surface was extremely low (vapor pressure in the chamber typically changed <0.001 mbar/min), obviating the first problem, and our site was quite windy, obviating the second.
To allow us to resample the same area of snow several times, we constructed an aluminum frame that sealed onto the Plexiglas box and extended down into the snow ≈5 cm. We pressed the frame down into the snow at the beginning of a series of measurements and left it in place, clamping the box onto the frame for a measurement (which lasted ≈3 min; Fig. Fig.1)1) and propping it up on one edge between measurements. The box was sealed to the frame with a closed-cell foam gasket.
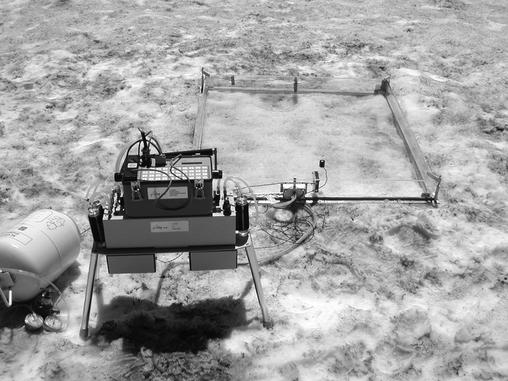
The gas-exchange apparatus in place on the snow. Patches that appear dark in this black-and-white photograph are pink watermelon snow colonized by C. nivalis.
We used natural sunlight as our sole light source, reducing the incident irradiance with multiple layers of wire and fiberglass window-screening material when we made light curves. For the colored-light experiments, we used Roscolene filters 863 medium blue, 874 medium green, and 823 medium red (Roscoe Laboratories, Stamford, CT). Fig. Fig.22 shows the spectra of these filters and also of the Plexiglas box obtained with a Hewlett–Packard Diode-Array Spectrophotometer (model 8452A, Hewlett–Packard, Waldbronn, Germany). We measured light with a quantum sensor (LI-190SA, Li-Cor) at snow level under the chamber for light curves and color experiments or on top of the chamber (adjusting light value for the Plexiglas) for daily courses.
Our field site was in the Snowy Range of the Rocky Mountains, in a persistent snowfield ≈41° 21′ N latitude, 106° 16′ W longitude, at an altitude of ≈3,250 m (10,700 feet). Like most of the snowfields in this area, this field showed both white areas and deep pink areas, indicating the absence and presence, respectively, of snow-algal communities. Snow depths ranged from >2 m to as little as 13 cm. We made measurements in the summers of 1999, 2000, and 2001, usually in early July.
To determine the concentration of C. nivalis cells contributing to CO2 exchange, we collected the snow under the gas-exchange chamber at the end of the day to a depth where we no longer saw any pink color, usually ≈10 cm (although there was considerable variability in the maximum depth to which we found algae). We melted the sample completely, measured its volume, filtered it through a coarse screen to remove debris such as pine and fir leaves, and took several 50-ml samples after thorough mixing. Each 50-ml sample was centrifuged at 1,300 rpm for 1.5 min, after which we carefully decanted the supernatant, leaving a volume of 5.0 ml. We resuspended the cells in this volume and counted them by using a hemacytometer, thus getting an estimate of 10 times the concentration of cells in the original snow sample.
Results
To refine our setup and determine initially whether we could observe any net CO2 exchange at all, we picked a brilliant sunny day, placed the box directly on the snow, and compared a snow patch that was obviously red with one that had no apparent red coloring (Fig. (Fig.3).3). The pink snow had a net CO2 uptake rate of ≈0.3 μmolm−2
s−1, whereas a nearby patch of snow with no pink color showed an order of magnitude less. The pink patch had a measurable rate of CO2 efflux (0.016 μmol
m−2
s−1) when we placed a black cloth over the box. These results demonstrated that the apparatus had sufficient sensitivity to detect snow-surface gas exchange and that the CO2 uptake was coming from algal photosynthesis. Because we had not yet constructed the sealing frame, the specific numbers may not be accurate, and indeed they are substantially higher than any other rates we measured.
To develop an idea of how much carbon exchange occurs in algae-colonized patches of snow over the long term, we placed the frame in the snow and measured gas-exchange rates roughly every hour, over several dawn-to-dusk periods (Figs. (Figs.44 and and5;5; other experiments showed similar patterns). We recorded light (Figs. (Figs.44B and and55B) and snow temperature (Figs. (Figs.44C and and55C) by using the PAR sensor and leaf thermocouple supplied with the gas-exchange system at the same time we measured gas exchange. CO2-exchange rates always peaked near mid-day and were lowest at dawn and sunset, and the difference between dark-measured rates and peak rates was usually on the order of 0.1–0.3 μmolm−2
s−1, or ≈1 × 10−9 μmol per cell
s−1. However, the actual values of net CO2 uptake were quite variable, often starting from negative values, sometimes as low as −0.5 μmol
m−2
s−1 and only barely reaching positive values at mid-day (compare Fig. Fig.44 with Fig. Fig.5).5).
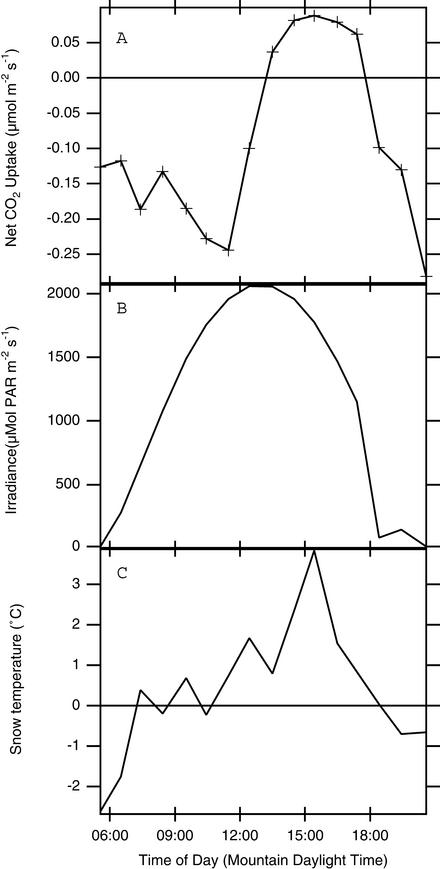
Daily course of gas exchange over a single patch of algae-colonized snow obtained on June 21, 2000. (A) Area-specific rates of photosynthesis. (B) Photosynthetically active radiation outside the chamber. (C) Snow temperature.
To investigate photosynthetic light response under field conditions, we generated a light curve beginning with full sun and reducing the light by successively adding layers of window screen. The resulting light curve (Fig. (Fig.6)6) seems to be quite similar to that typically found in higher plants, with a linear increase at low light, saturation at high light, and no indication of photoinhibition even when PAR was at its maximum for the day, 1,872 μmol PARm−2
s−1. The maximum rate of photosynthesis we found was ≈0.1 μmol CO2
m−2
s−1.
We measured CO2 exchange in different colors of light in the field to see how gas-exchange measurements correlated with the spectral properties of individual cells we reported previously, where cells were found to screen out light of wavelengths <550 nm (18). We used broad-band filters to determine the photosynthetic response to red, blue, and green light by using window screen to adjust the total PAR inside the cuvette so it was approximately the same for each color. We superimposed these results on a light curve for white light obtained the same day (Fig. (Fig.6).6). Red light clearly drove more photosynthesis than the equivalent irradiance of white, and blue and green both drove significantly less, because astaxanthin prevented the short-wavelength light from reaching the chloroplast. The blue filter we used had some transmittance in the red (Fig. (Fig.2),2), and we expect that a purer blue would have shown even less photosynthesis because astaxanthin blocks almost all of the blue light (19).
Discussion
C. nivalis clearly takes up CO2 from the air, and some particularly rich patches of snow have area-specific rates ≈10% of the rates of many green plants. We found large patch-to-patch variations in gas-exchange rates, usually correlated with algal densities but not always (data not shown). We did the light curve (Fig. (Fig.6)6) in the year 2001, the black/white experiment (Fig. (Fig.3)3) in 1999, and daily course measurements in 2000 and 2001; 2000 was a very low snow year for the Snowy Range, and there was clearly much less snow algae present than in the year before, even in those few snowfields that persisted over the summer. The year 2001 was intermediate between 1999 and 2000. Rates at light saturation varied from 0 (with dark rates strongly negative) to 0.3 μmol CO2m−2
s−1, and algal densities only seemed to account for part of this variability. In particular, even very red patches (when they were possible to find) from the low-snow year of 2000 seemed to have much lower rates than moderately pink patches from the year before. Algal densities averaged ≈2 × 107 cells m−2 in pink patches in all years sampled, so variation in cell concentration apparently cannot account for all of the variation we saw in photosynthetic rates.
It is likely that C. nivalis gets much of its CO2 from respiration within or beneath the snow, as indicated by some of our daily courses in which practically all values of photosynthesis were negative; i.e., the patches of snow were giving off CO2 rather than taking it up (Fig. (Fig.4).4). Presumably, curves such as these occur because the algae's photosynthetic activity acts against a background of constant CO2 efflux from the snow surface. Such efflux certainly does occur: in winter and spring, CO2 effluxes from the snow ranging from 0.2 to 0.5 μmol CO2m−2
s−1 have been calculated from a location quite close to our field site (21, 22). As a rule, our more negative gas-exchange rates came from algal patches growing in relatively thin snow, which is consistent with these studies. Thus, some of the patch-to-patch variation we observed is probably caused by localized variations in under-snow respiration rates and is, perhaps, caused by different substrates (i.e., rock slabs vs. soil) and different snow depths. Perhaps stable-isotope studies could illuminate what proportion of the algae's carbon comes from the atmosphere and what comes from soil respiration.
We saw no indication of photoinhibition in the field (Fig. (Fig.6)6) despite the near-freezing temperatures, high incident light levels, and reflection from the underlying snow. The algae's high content of astaxanthin, located around the chloroplast where it can absorb light that might otherwise strike the photosynthetic apparatus, probably protects the cells from excessive light. In addition, the pigments in any single cell will absorb some of the light that might otherwise strike neighboring cells, reducing light levels below what might be found in algae-free snow. Astaxanthin in C. nivalis absorbs blue light strongly, as shown by spectrophotometric measurements of monolayers of cells and of individual cells (18). The astaxanthin can also confer substantial UV protection, and the algae also contain phenolics that may help protect against UV damage; the concentration of phenolics is regulated in part by UV exposure (25). It may be that the algae close to the surface were being photoinhibited while increased contributions from the lower layers of algae, which were receiving less light, compensated for this. It is also possible that our experimental conditions were simply not severe enough to cause photoinhibition. The Plexiglas cuvette transmitted ≈92% of the visible light but virtually no UV light, so algae under the box were exposed to somewhat less severe radiation stress than they would be likely to experience outside the apparatus.
With the data presented here, it is possible to estimate what contribution photosynthesis in the snow might make to large-scale CO2 exchange. If we integrate the data from Fig. Fig.5,5, we get a daily rate of 2,256 μmolm−2
day−1, or 7.78 × 10−5 μmol per cell per day. If we knew the total quantity of algae in a given area of snow, we could multiply it by such an integrated rate to obtain daily CO2 flux. Painter et al. (26) in the Sierra Nevada Mountains of California have recently developed the use of remote sensing to estimate snow-algae densities: a trial scan of their field site found a mean concentration of 1,300 cells ml−1 over an area of 0.495 km2 of snow. Recent reanalysis of their data suggests that the number should be closer to 300 cells ml−1 (T. H. Painter, personal communication). Their assumption that all of the cells are in the top 10 cm of snow would give an average areal density of 3 × 107 cells m−2. Multiplying by the integrated photosynthesis rate of 7.78 × 10−5 μmol per cell per day gives a mean daily CO2-fixation rate of 2.3 × 103 μmol
m−2 per day, or 2.8 × 104 μg C m−2 per day. Assuming that half of the algal biomass is carbon (27) and that the snow algae are active for 90 days per year, we get an overall annual net primary production (NPP) of ≈5 g m−2. This result is small but significant, ≈2% of the area-specific productivity of arctic heathlands.¶
This approach, combining gas-exchange measurements with remote sensing of algal concentrations, is promising, but obviously more work needs to be done before we can develop large-scale estimates of snow-algae productivity with confidence. For example, the estimate of average algal concentration over a large area by Painter et al. (26) is similar to our measurements of algal concentration in patches consciously chosen for their conspicuous red color. Possibly the area of the Sierra where they worked is much richer in snow algae than the area of the Rockies where we worked, or perhaps there are differences in our methods of measurement or other calculations that could lead to this discrepancy. Also, the area covered by snow algae and the concentration within that area vary greatly during the season: the algae multiply at the same time the snow-cover decreases because of melting. Thus, periodically taking a census of the algal population would be required.
It would be illuminating to know more about the overall ecology of snow algae. We have observed that the algae tend to concentrate on the surface in areas where liquid water runs down into the snow or over the snow. This pattern could be caused by simple passive distribution with the flowing water, but the alga's mucilaginous sheath might also allow it to stay preferentially in areas where liquid water was more available. We also observed that algae seem to concentrate in areas where soil run-off flows onto the surface of the snow (rather than under the snow as happens more frequently). It could be that the nutrients that accompany soil run-off stimulate algal blooms, or perhaps the algal propagules come from the soil. The highest concentrations of snow algae of which we are aware occur on Svalbard (6, 15), below cliffs that support extensive bird-nesting areas, suggesting that nutrient availability can exert a powerful effect on algal concentrations.
Clearly, snow cannot be assumed to be a net CO2 producer, particularly in the summer months. In fact, under some circumstances it can be a significant CO2 sink.
Acknowledgments
We thank Kathleen Painter, Caroline Boice, Diane Azu, and Elizabeth Krause for being able and enthusiastic field assistants; we also thank Patrick Morgan for his field help. We thank Jan O'Dell and Frances Titus for providing essential logistic help. We thank Mike Sanders (supervisor, Medicine Bow-Routt National Forest) for permission to work in Medicine Bow National Forest. Li-Cor, Inc., provided generous advice and quick turnaround on equipment repairs, and we particularly thank Tanvir Demetriades-Shah and John Wells. This work was supported in part by National Science Foundation Grant LEXEN IBN-97-12268 (to T.C.V.) and also by faculty-development grants from St. Mary's College of Maryland (to H.L.G. and W.E.W.).
Abbreviation
PAR | photosynthetically active radiation |
Footnotes
This paper was submitted directly (Track II) to the PNAS office.
§Hoham, R. W., Sixtieth Annual Western Snow Conference, April 14–16, 1992, Jackson, WY, pp. 78–83.
¶Shaver, G. R. (2001) NPP Tundra: Toolik Lake, Alaska, 1982 (Oak Ridge National Laboratory Distributed Active Archive Center), Vol. 2002, www.daac.ornl.gov/.
References
Articles from Proceedings of the National Academy of Sciences of the United States of America are provided here courtesy of National Academy of Sciences
Full text links
Read article at publisher's site: https://doi.org/10.1073/pnas.0235560100
Read article for free, from open access legal sources, via Unpaywall:
https://europepmc.org/articles/pmc141035?pdf=render
Citations & impact
Impact metrics
Citations of article over time
Alternative metrics

Discover the attention surrounding your research
https://www.altmetric.com/details/102522384
Article citations
Greenland Ice Sheet Surfaces Colonized by Microbial Communities Emit Volatile Organic Compounds.
Front Microbiol, 13:886293, 07 Jun 2022
Cited by: 1 article | PMID: 35747370 | PMCID: PMC9211068
The colors of life: an interdisciplinary artist-in-residence project to research fungal pigments as a gateway to empathy and understanding of microbial life.
Fungal Biol Biotechnol, 9(1):1, 10 Jan 2022
Cited by: 3 articles | PMID: 35012670 | PMCID: PMC8744264
Extremophilic Microorganisms in Central Europe.
Microorganisms, 9(11):2326, 10 Nov 2021
Cited by: 6 articles | PMID: 34835450 | PMCID: PMC8620676
Review Free full text in Europe PMC
Investigating the Growth of Algae Under Low Atmospheric Pressures for Potential Food and Oxygen Production on Mars.
Front Microbiol, 12:733244, 12 Nov 2021
Cited by: 7 articles | PMID: 34867849 | PMCID: PMC8633435
Revealing the Characteristics of the Antarctic Snow Alga Chlorominima collina gen. et sp. nov. Through Taxonomy, Physiology, and Transcriptomics.
Front Plant Sci, 12:662298, 07 Jun 2021
Cited by: 6 articles | PMID: 34163502 | PMCID: PMC8215615
Go to all (21) article citations
Other citations
Similar Articles
To arrive at the top five similar articles we use a word-weighted algorithm to compare words from the Title and Abstract of each citation.
The light environment and cellular optics of the snow alga Chlamydomonas nivalis (Bauer) Wille.
Photochem Photobiol, 73(6):611-620, 01 Jun 2001
Cited by: 26 articles | PMID: 11421066
Ecophysiology, secondary pigments and ultrastructure of Chlainomonas sp. (Chlorophyta) from the European Alps compared with Chlamydomonas nivalis forming red snow.
FEMS Microbiol Ecol, 92(4):fiw030, 15 Feb 2016
Cited by: 24 articles | PMID: 26884467 | PMCID: PMC4815433
Detection and quantification of snow algae with an airborne imaging spectrometer.
Appl Environ Microbiol, 67(11):5267-5272, 01 Nov 2001
Cited by: 23 articles | PMID: 11679355 | PMCID: PMC93300
Energy transfer reactions involving carotenoids: quenching of chlorophyll fluorescence.
J Photochem Photobiol B, 36(1):3-15, 01 Oct 1996
Cited by: 44 articles | PMID: 8988608
Review