Abstract
Free full text
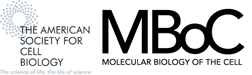
Compartmentalization of the Exocyst Complex in Lipid Rafts Controls Glut4 Vesicle Tethering
Abstract
Lipid raft microdomains act as organizing centers for signal transduction. We report here that the exocyst complex, consisting of Exo70, Sec6, and Sec8, regulates the compartmentalization of Glut4-containing vesicles at lipid raft domains in adipocytes. Exo70 is recruited by the G protein TC10 after activation by insulin and brings with it Sec6 and Sec8. Knockdowns of these proteins block insulin-stimulated glucose uptake. Moreover, their targeting to lipid rafts is required for glucose uptake and Glut4 docking at the plasma membrane. The assembly of this complex also requires the PDZ domain protein SAP97, a member of the MAGUKs family, which binds to Sec8 upon its translocation to the lipid raft. Exocyst assembly at lipid rafts sets up targeting sites for Glut4 vesicles, which transiently associate with these microdomains upon stimulation of cells with insulin. These results suggest that the TC10/exocyst complex/SAP97 axis plays an important role in the tethering of Glut4 vesicles to the plasma membrane in adipocytes.
INTRODUCTION
Insulin stimulates glucose transport in fat and muscle cells through a process of regulated vesicle recycling in which the facilitative glucose transporter Glut4 is translocated from intracellular sites to the plasma membrane (Saltiel and Kahn, 2001; Bryant et al., 2002). In unstimulated cells, Glut4 undergoes endocytosis into endosomes and subsequently sorts into specialized storage vesicles that traffic to the plasma membrane after activation of the insulin receptor. The vesicles then dock and fuse at specific sites at the membrane, resulting in extracellular exposure of the transporter. The precise signals from the insulin receptor that control these events involve the tyrosine phosphorylation of a number of intracellular substrates. These phosphorylations lead to activation of the PI3-kinase pathway (Saltiel and Kahn, 2001) and also to activation of the G protein TC10 (Chiang et al., 2001; Watson et al., 2001; Maffucci et al., 2003), which in turn binds to numerous effectors, including the exocyst protein Exo70 (Inoue et al., 2003). Exo70 exists in a multiprotein complex with the proteins Sec6 and Sec8. A dominant-negative mutant of Exo70 blocked insulin-stimulated glucose uptake, but was without effect on translocation of Glut4 to the plasma membrane. However, this Exo70 mutant prevented the appearance of Glut4 at the cell surface, leading us to propose that the exocyst complex may play a critical role in tethering Glut4 vesicles to the plasma membrane for subsequent docking and fusion (Inoue et al., 2003).
Lipid rafts are specialized compartments of the plasma membrane enriched in cholesterol and glycosphingolipids. Numerous signaling and cytoskeletal proteins are found in these subdomains, suggesting that they may act as organizing centers for signal transduction, particularly for insulin (Anderson, 1998; Schlegel et al., 1998; Watson and Pessin, 2001; Bickel, 2002; Saltiel and Pessin, 2002, 2003). Both the insulin receptor and TC10 reside in lipid rafts (Yamamoto et al., 1998; Gustavsson et al., 1999; Nystrom et al., 1999; Watson et al., 2001; Kimura et al., 2002; Vainio et al., 2002), and the mistargeting of TC10 to a nonlipid raft domain prevents its activation by insulin and blocks insulin action (Watson et al., 2001; Kanzaki and Pessin, 2002; Chunqiu Hou and Pessin, 2003; Watson et al., 2003).
MATERIALS AND METHODS
Materials
Anti-HA monoclonal antibody (mAb) and anti-insulin receptor β antibody were obtained from Santa Cruz Biotechnology (Santa Cruz, CA), and anti-GFP polyclonal antibody was obtained from Abcam (Cambridge, MA). Anti-total Akt and phospho-Akt antibodies were from Cell Signaling (Beverly, MA). Anti-Sec6, Sec8, and SAP97 monoclonal antibodies were from Stressgen (San Diego, CA). Anti-caveolin polyclonal antibody and anti-caveolin2 mAb were from BD Transduction Laboratory (San Jose, CA). Anti-Glut4 polyclonal antibody was from Alpha Diagnostic International (San Antonio, TX). Anti-Exo70 mAb kindly provided from Dr. Shu-Chan Hsu (Rutgers University; Vega and Hsu, 2001).
Cell Culture and Electroporation of 3T3L1 Adipocytes
The 3T3L1 fibroblasts were grown in DMEM with 10% fetal bovine serum (FBS) and differentiated into adipocytes as described (Baumann et al., 2000). The 3T3L1 adipocytes were transfected with stealth RNA interference (RNAi) duplexes (Invitrogen, Carlsbad, CA) by electroporation. In brief, adipocytes at day 2 of differentiation were detached from culture dishes with 0.25% trypsin, washed twice, and resuspended in phosphate-buffered saline (PBS). Approximately 5 × 106 cells (half of the cells from one p150 dish) were mixed with RNAi duplexes, which were delivered to the cells by electroporation with a Bio-Rad gene pulser II system (Richmond, CA; 0.16 kV and 960 μF). After electroporation, cells were mixed with medium for 10 min before replating.
Immunoprecipitation and Immunoblotting
Cells were lysed with HNTG buffer using 1% Triton X-100. Lysates were incubated with the indicated antibodies for 2 h at 4°C. The immune-complexes were precipitated with protein A or G agarose beads (Amersham Pharmacia, Piscataway, NJ) and washed with lysis buffer. The samples were resolved in 4-20% gradient SDS-PAGE and analyzed by immunoblotting. All immunoblots were developed by enhanced chemiluminesence (Amersham Pharmacia).
Immunofluorescence Microscopy
For immunofluorescence studies, cells were processed as described (Kimura et al., 2001, 2002; Liu et al., 2005). To make plasma membrane sheets, cells were treated for 5 min with the Triton extraction buffer (50 mM MES, pH 6.0, 150 mM NaCl, 0.25% Triton X-100, 1 mM CaCl2, 0.5 mM MgCl2) before fixation. To detect myc-Exo70, cells were stained with anti-myc mAb or polyclonal antibody (Santa Cruz Biotechnology). To detect HA-TC10, cells were stained with anti-HA mAb (Santa Cruz Biotechnology). After incubation with primary antibodies, cells were incubated with Alexa488 or Alexa594 goat anti-mouse or anti-rabbit immunoglobulin (IgG), obtained from Molecular Probes (Invitrogen, Eugene, OR). Images were captured by using an Olympus FV300 confocal laser scanning microscope (Melville, NY).
Expression Constructs
To generate a mammalian expression vector, full-length Sec8 was subcloned into the pKH3 vector. YFP-mycSAP97 construct was a gift from Dr. Benjamin Margolis (University of Michigan). The His-tagged proteins Sec8 and its Δ4aa deletion mutant were cloned into a pET15b vector.
2-Deoxyglucose Uptake Assay
The RNAi-transfected cells were reseeded on 12-well plates and cultured for 4 d. After incubation with DMEM containing 0.5% FBS for 3 h, cell were washed with Krebs-Ringer buffer (130 mM NaCl, 5 mM KCl, 1.3 mM CaCl2, 1.3 mM MgSO4, 25 mM HEPES, pH 7.4) and incubated with or without insulin for 30 min. Glucose uptake was initiated by addition of [14C]2-deoxy-d-glucose to a final assay concentration of 100 μM for 5 min and terminated by three washes with ice-cold PBS, the cells were solubilized with 0.1% SDS, and [14C] was determined by scintillation counting.
Sucrose Gradient Centrifugation
3T3L1 adipocytes were homogenized in HES buffer (20 mM HEPES, pH 7.5, 250 mM sucrose, 1 mM EDTA) with a Dounce homogenizer. After homogenization, cells were centrifuged at 800 × g and the supernatant was centrifuged at 15,000 × g to produce a total plasma membrane fraction. Total plasma membrane fraction was solubilized in 0.3 ml of 0.2% Triton X-100 in MBS, and made up to 0.4 ml with MBS. These samples were mixed with 0.4 ml of 80% sucrose (wt/vol), and overlaid with 3.5 ml of 30%, 1 ml of 5% sucrose. The gradient was centrifuged at 150,000 × g for 19 h, and 440-μl fractions were collected from the top of each gradient.
PDZ Domain Array
His-tagged Sec8 wild-type and Sec8Δ4aa mutant protein were prepared as previously described (Taylor et al., 2000). TranSignal PDZ Domain Arrays (Panomics, Redwood City, CA) were used as instructed.
RESULTS
The Exocyst Complex Is Necessary for Glucose Uptake in Adipocytes
The exocyst is a complex of eight proteins involved in targeted exocytosis in many cell types (TerBush et al., 1996; Kee et al., 1997; Lipschutz and Mostov, 2002; Yeaman, 2003; Guo and Novick, 2004; Hsu et al., 2004; Tsuboi et al., 2005). We sought to evaluate whether exocyst proteins play a crucial role in insulin-stimulated Glut4 vesicle trafficking and glucose transport. We knocked down components of the exocyst complex by electroporation of 3T3L1 adipocytes with RNAi oligomers and assayed insulin-stimulated 2-deoxyglucose uptake (Figure 1, A and B). In 3T3L1 adipocytes transfected with a scrambled oligomer, insulin produced an eightfold increase in glucose uptake. Knockdown of total Exo70 protein by 50-70% inhibited insulin-stimulated glucose transport by ~50% compared with the control scrambled RNAi oligo. This inhibitory effect of knocking down Exo70 was seen at all concentrations of insulin. Similar results were observed using two other RNAi oligos targeting two distinct sequences of Exo70 (unpublished data). Importantly, knockdown of Exo70 did not evoke any change in cell shape or proliferation, expression of insulin receptor or Glut4, or insulin-stimulated phosphorylation of Akt (Figure 1, C-E). It should also be pointed out that it is uncertain whether total protein changes reflect fractional changes in all cells or large changes in fractions of cells.
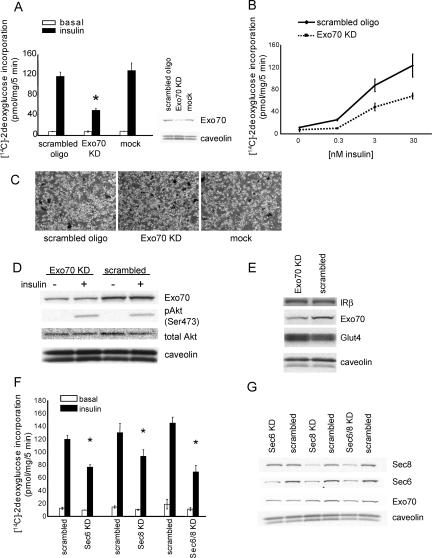
Knockdown of the exocyst complex blocks glucose uptake in 3T3L1 adipocytes. (A) Differentiated 3T3L1 adipocytes were transfected with Exo70 RNAi or scrambled oligo. Four days later, the cells were treated with or without 100 nM insulin for 30 min, and the rate of 2DG uptake was determined. Results are the mean ± SD of triplicate determinations and were reproduced five times. *Significant difference, p < 0.05. Protein expression levels were checked by Western blot for each assay. (B) The dose dependence of insulin on glucose uptake was assayed in the presence or absence of Exo70 RNAi or scrambled oligo as indicated. (C) Micrographs of 3T3L1 adipocytes 4 d after transfection of RNAi oligo as indicated. (D and E) Effect of Exo70 knockdown on insulin-stimulated Akt phosphorylation, insulin receptor and Glut4 expression. (F) Sec8, Sec6, or both were knocked down in 3T3L1 adipocytes using RNAi oligos, and 2DG uptake was assayed 4 d after transfection. Results are the mean ± SD of triplicate determinations and were reproduced three times. *Significant difference, p < 0.05. (G) The effect of Sec6 and Sec8 knockdown on each molecule and Exo70 were examined by immunoblot.
To further investigate the role of the exocyst in glucose uptake, we knocked down two other components of the complex, Sec6 and Sec8, followed by assay of insulin-stimulated 2-deoxyglucose uptake (Figure 1F). As was observed with Exo70, the knockdown of these two molecules also blocked insulin-stimulated glucose uptake. Moreover, combined electroporation with Sec6 and Sec8 RNAi oligos resulted in more effective inhibition. Interestingly, knockdown of Sec8 markedly reduced the levels of endogenous Sec6 protein. Because Sec8 appears to be in significant excess in adipocytes, these data suggest that Sec8 stabilizes the Sec6 protein in the complex (Figure 1G).
Insulin Controls the Localization of the Exocyst Complex
To evaluate the role of lipid rafts in coordinating the assembly of the exocyst complex, we performed a number of immunohistochemistry and fractionation experiments to assay the localization of exocyst proteins in these microdomains. Differentiated 3T3L1 adipocytes were transfected with cDNAs expressing HA-TC10 and its mutants, along with myc-Exo70 and caveolin-eGFP as a marker of lipid rafts. The cells were then stimulated with insulin, and plasma membrane sheets were prepared by a triton extraction method that leaves only lipid raft proteins on the cover glass (Watson et al., 2001). As previously reported (Watson et al., 2001; Chiang et al., 2002), both caveolin and TC10 constitutively reside in detergent-extracted sheets derived from untreated and insulin-stimulated cells. Exo70 protein was not detected in these sheets in untreated cells, but was found in sheets from cells treated with insulin. Moreover, coexpression with constitutively active TC10 (Q67L) produced the localization of Exo70 at lipid rafts even without insulin stimulation (Figure 2A). Although we are unable to detect endogenous Sec8, we transfected cells with Myc- or HA-tagged Sec8 and treated cells with or without insulin. Insulin stimulated the translocation of Sec8 to the plasma membrane. This effect of the hormone was mimicked by cotransfection of cells with constitutively active TC10 (Supplementary Figure 1).
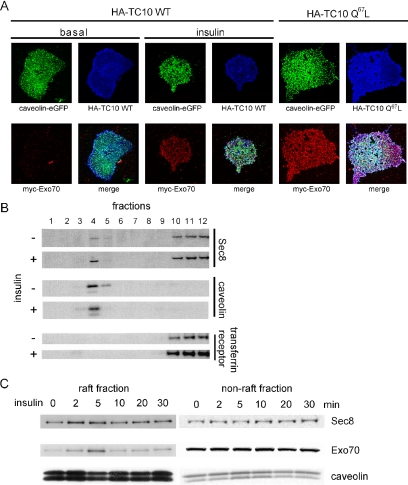
Insulin stimulates the translocation of exocyst proteins to lipid rafts. (A) HA-TC10 wild type or TC10 (Q67L) was transfected into 3T3L1 adipocytes with myc-Exo70 and caveolin-eGFP. To make plasma membrane sheets, cells were treated for 5 min with the Triton extraction buffer. The plasma membrane sheets were fixed and stained with an anti-HA mAb and anti-myc polyclonal antibody followed by Alexa633 goat anti-mouse IgG and Alexa568 goat anti-rabbit IgG. The cells transfected with HA-TC10WT were starved overnight and treated with or without insulin for 5 min (left 8 panels). The cells transfected with HA-TC10 Q67L were not starved or stimulated by insulin (right 4 panels). (B) After a 5-min insulin stimulation, 3T3L1 adipocytes were harvested in the nondetergent buffer followed by sucrose density gradient fractionation. The expression of caveolin, transferrin receptor and Sec8 were evaluated by immunoblot. (C) Cells treated with insulin for the indicated times were lysed and fractionated on sucrose gradients. Fractions 4 and 5 were pooled as the lipid raft fraction, and fraction 10-12 were pooled as the nonlipid raft fraction. These samples were resolved in 4-20% gradient SDS-PAGE and analyzed by immunoblotting with anti-Exo70, Sec8, and caveolin antibodies.
To confirm the localization of exocyst proteins in lipid rafts, we performed sucrose density gradient fractionation of purified plasma membranes prepared from untreated and insulin-treated cells, followed by immunoblot analysis, as previously described (Chamberlain and Gould, 2002; Chiang et al., 2002; Kimura et al., 2002). Adipocytes were treated with or without insulin for 5 min, followed by lysis and fractionation. Caveolin1 was exclusively detected in the lipid raft-enriched fraction 4 and 5 of the gradient and was unaffected by insulin treatment, as previously shown (Chamberlain and Gould, 2002). In contrast, the transferrin receptor was exclusively found at the bottom of the gradient in fractions 10-12, where it increased in response to insulin (Chamberlain and Gould, 2002). Interestingly, Sec8 was detected in both raft (fractions 4 and 5) and nonraft fractions (10-12) in untreated cells. However, both proteins were increased only in the lipid raft fractions in cells treated with insulin (Figure 2B).
To analyze the kinetics of this effect of insulin in more detail, we performed a time course of insulin stimulation. After hormone treatment, the plasma membranes were purified and fractionated further on sucrose gradients, and the raft and nonraft fractions were pooled before immunoblotting. Although the proteins residing in the nonraft fractions did not change as a consequence of insulin stimulation, treatment of cells with insulin produced the rapid and transient translocation of both Exo70 and Sec8 into the lipid raft fraction. This effect of insulin was maximal at 5 min and declined thereafter (Figure 2C).
Glut4 Vesicles Transit through Lipid Rafts before Fusion
Glut4-containing vesicles translocate to the plasma membrane in response to insulin stimulation (Saltiel and Kahn, 2001). However, whether these vesicles are targeted to discrete sites on the plasma membrane is not known. Because the exocyst appears to assemble in large part at lipid rafts, we sought to evaluate whether Glut4 might be targeted to these microdomains early in the translocation process. Thus, to evaluate the kinetics of Glut4 translocation to the plasma membrane, we examined the time course of Glut4 translocation using the fractionation method described above and determined the concentration of Glut4 in lipid raft and nonlipid raft fractions of the plasma membrane after insulin stimulation (Figure 3A). Glut4 was rapidly translocated to rafts in response to insulin. This effect was observed in as little as 2 min and reached maximal levels 10 min after insulin stimulation. The translocation of Glut4 into raft fractions preceded the appearance of the protein in nonlipid raft fractions, where peak levels were detected 30 min after insulin stimulation. Thus, these data suggest two possible models: one in which Glut4 is initially translocated to lipid rafts, and then transits to nonraft fractions, perhaps after fusion of the vesicles, and a second in which there are two pools of Glut4, a fast pool targeted to rafts and a slower pool target to nonraft regions.
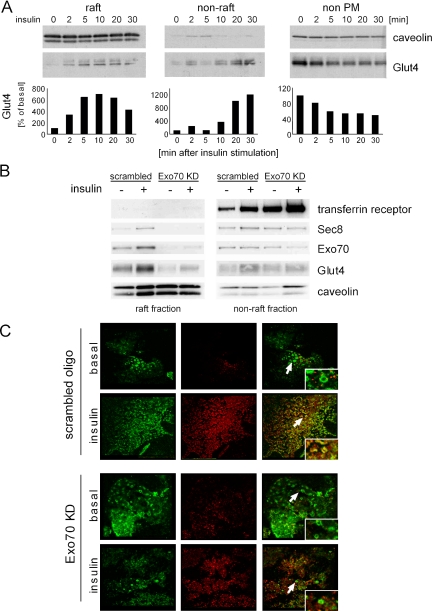
Time course of Glut4 translocation to lipid rafts. (A) Glut4 expression in lipid raft, nonlipid raft, or nonplasma membrane fractions were examined at different time points after insulin stimulation. The intensity of Glut4 levels in the raft, nonraft, and nonplasma membrane were quantified. (B) The lipid raft and nonlipid raft fractions purified by sucrose gradient from the different conditions (with or without insulin stimulation, Exo70 RNAi or scrambled oligo-transfected as indicated) were loaded on the same gel and blotted with anti-Exo70, Sec8, Glut4, transferrin receptor, and caveolin antibodies. (C) Four days after transfection of Exo70 or scrambled RNAi oligo, cells were treated with insulin for 10 min and incubated with Triton extraction buffer to make plasma membrane sheets. The plasma membrane sheets were fixed and stained with anti-Glut4 polyclonal antibody and anti-caveolin2 mAb followed by Alexa594 goat anti-rabbit IgG and Alexa488 goat anti-mouse IgG. Arrows show the rosette structures.
The Exocyst Complex Directs Glut4-containing Vesicles to Lipid Rafts
Because both Glut4 and exocyst proteins translocate to lipid rafts in response to insulin, we wondered whether the assembly of the exocyst complex plays a crucial role in the trafficking of Glut4 vesicles to these microdomains in the adipocyte. We therefore used RNAi to selectively knockdown exocyst proteins and evaluated their targeting to lipid raft fractions. Exo70 knockdown reduced the insulin-stimulated translocation of both Glut4 and Sec8 into the lipid raft fraction, but did not markedly affect the levels of either protein in the nonraft fractions, nor did it affect the translocation of the transferrin receptor (Figure 3B).
We next examined the effect of Exo70 knockdown on the translocation of Glut4 to detergent-extracted plasma membrane sheets. Cells were electroporated with Exo70 RNAi or a scrambled oligo and then treated with or without insulin for 10 min. In scrambled oligo-transfected cells, endogenous Glut4 and caveolin costained in circular rosette structures in plasma membrane sheets, suggesting that a subpopulation of Glut4 vesicles translocate to lipid rafts after insulin stimulation (Figure 3C). Knockdown of Exo70 disrupted the colocalization of Glut4 and caveolin in rosettes, suggesting that the exocyst complex selectively tethers Glut4 to lipid rafts in the early phase of insulin signaling.
We previously reported that an Exo70 mutant containing only the N-terminal sequences (Exo70-N) inhibited insulin-stimulated glucose uptake (Inoue et al., 2003). To evaluate the mechanism of action of this mutant form of Exo70, we examined its localization in the plasma membrane. 3T3L1 adipocytes were electroporated with cDNAs encoding myc-Exo70-WT and myc-Exo70-N or a vector control and treated with or without insulin for 5 min. Subcellular localization of transfected and endogenous exocyst proteins was then evaluated by sucrose density gradient fractionation (Figure 4A). As shown above in untransfected cells, levels of Exo70, Sec8, and Glut4 were low in lipid raft fractions from unstimulated cells expressing Exo70-WT, but increased upon insulin stimulation. In contrast, the overexpression of myc-Exo70-N in adipocytes suppressed the insulin-dependent translocation of Exo70, Sec8, and Glut4 to lipid raft fractions. These data suggest that endogenous Exo70 and other components of the exocyst complex are competitively displaced from lipid rafts by overexpression of the dominant-negative form of the protein.
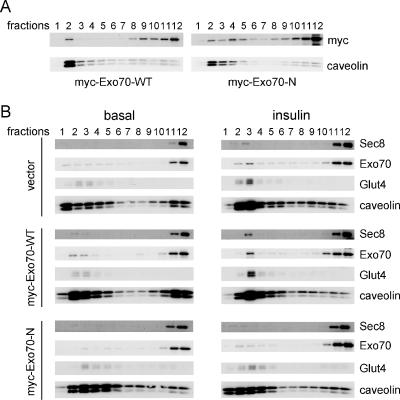
Dominant negative Exo70 inhibits Glut4 movement to lipid rafts. (A) Myc-Exo70-WT and -N were overexpressed in 3T3L1 adipocytes by electroporation and harvested 3 d later without starvation or stimulation by insulin. Sucrose gradients fractionations were performed as described above, and fractions were blotted with anti-myc mAb and anti-caveolin antibody. (B) Vector control, myc-Exo70-WT and -N overexpressed 3T3L1 adipocytes were treated with or without insulin for 5 min, and sucrose gradient fractionation were performed as in A. The fractions were loaded in SDS-PAGE and blotted with anti-Sec8 mAb, anti-Exo70 mAb, anti-Glut4 polyclonal antibody, and anti-caveolin polyclonal antibody.
SAP97 Anchors the Exocyst Complex to Lipid Rafts
To identify the molecular basis by which the exocyst proteins are specifically localized in lipid rafts, we looked for proteins that might interact with the abundant protein Sec8 upon its translocation. The recent cloning of the Sec8 gene revealed a PDZ-binding motif (ITTV) in the C-terminus of the protein, suggesting a possible interacting partner (Sans et al., 2003). We thus screened a PDZ protein array with bacterially expressed His-tagged Sec8 as bait and identified as an interacting partner SAP97, a member of the MAGUKs (membrane-associated guanylate kinase homologues) family (Funke et al., 2005). The specificity of this interaction was evaluated in Cos-1 cells cotransfected with cDNAs encoding HA-tagged Sec8 and YFP-tagged SAP97 (Figure 5A). SAP97 was coprecipitated with full-length Sec8, but not with a mutant Sec8 lacking a PDZ-binding motif in its C-terminus (Sec8-Δ4aa) (Figure 5A), verifying that SAP97 binds to Sec8 through the PDZ-binding motif in the C-terminus.
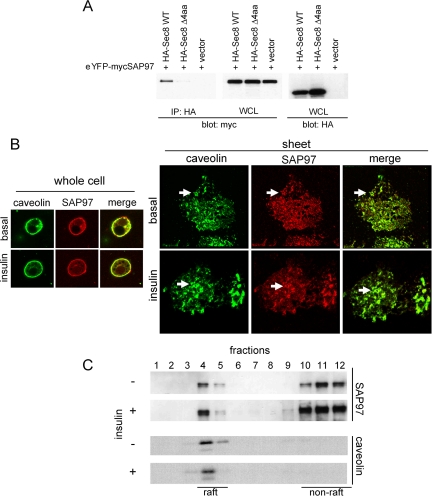
SAP97 binds to Sec8 through its PDZ-binding motif. (A) HA-Sec8 WT, HA-Sec8Δ4 amino acids and empty vector were cotransfected with eYFP-myc SAP97 in Cos-1 cells. Cells were harvested in HNTG buffer and incubated with anti-HA mAb for 2 h at 4°C. The immune complexes were precipitated with protein G beads for 1 h at 4°C, washed extensively with lysis buffer, resolved in a 4-20% gradient SDS-PAGE, and analyzed by immunoblotting with anti-YFP/eGFP polyclonal antibody. (B) Plasma membrane sheets were stained with anti-SAP97 mAb and anti-caveolin polyclonal antibody followed by Alexa594 goat anti-mouse IgG and Alexa488 goat anti-rabbit IgG. (C) Sucrose gradient samples from Figure 2B were blotted with anti-SAP97 mAb and anti-caveolin antibody.
SAP97 is reportedly expressed in lipid rafts in muscle cells (Folco et al., 2004). We explored the localization of SAP97 in 3T3L1 adipocytes by both immunostaining and sucrose density gradient fractionation. SAP97 clearly colocalized with caveolin in rosette structures in plasma membrane sheets (Figure 5B). Furthermore, we detected SAP97 in lipid raft fractions on sucrose density gradients (Figure 5C). There was little effect of insulin on the localization of SAP97.
To elucidate the functional significance of SAP97 as a binding partner of the exocyst protein Sec8, we knocked down SAP97 using RNAi oligo (Figure 6A). Knockdown of the SAP97 protein by 80% caused a marked reduction in the levels of both Sec8 and Exo70 in lipid rafts after insulin stimulation. Moreover, this reduction in SAP97 levels caused a blockade of the insulin-stimulated translocation of Sec8, Exo70, and Glut4 into lipid rafts, although the total amounts of these proteins in cell lysates were not altered (Figure 6B). Interestingly, the decreased content of the exocyst proteins in lipid rafts was accompanied by a significant increase of these proteins in nonlipid raft domains.
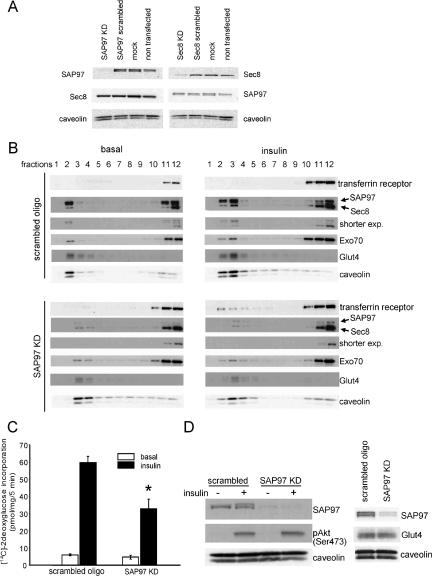
SAP97 anchors the exocyst complex to lipid rafts. (A) SAP97 RNAi, Sec8 RNAi, or scrambled oligo were transfected into 3T3L1 adipocytes. Four days later, cells were harvested without starvation or stimulation by insulin and blotted with anti-SAP97 mAb, anti-Sec8 mAb, and anti-caveolin polyclonal antibody. (B) Four days after transfection of SAP97 RNAi or scrambled RNAi, 3T3L1 adipocytes were harvested and sucrose gradient fractionation was performed. SAP97, Sec8, Exo70, Glut4, and caveolin were examined by immunoblot. (C) Differentiated 3T3L1 adipocytes were transfected with SAP97 RNAi or scrambled oligo, and 4 d later were assayed for 2DG uptake. Results are the mean ± SD of triplicate determinations and were reproduced four times. *Significant difference, p < 0.05. (D) Effect of SAP97 knockdown on insulin-stimulated Akt phosphorylation and Glut4 expression.
To evaluate the role of SAP97 in glucose transport, we evaluated insulin-stimulated glucose uptake in adipocytes electroporated with scrambled or SAP97 RNAi oligo. Consistent with the altered compartmentalization of exocyst complex and Glut4, insulin-stimulated glucose uptake in adipocytes was inhibited by more than 50% in cells treated with SAP97 RNAi oligo, without any effect on neither the expression of Glut4 or insulin-stimulated phosphorylation of Akt (Figure 6, C and D).
As a further evaluation of specificity regarding the effects of knockdown of components of the exocyst complex and related proteins, we first evaluated levels of insulin receptor in plasma membrane fractions (Supplementary Figure S2). As expected, receptor levels were diminished after treatment of cells with insulin for 10 min, due to internalization of the receptor. However, knockdown of Exo70, SAP97, Sec6, or Sec8 had no effect on insulin receptor levels in this plasma membrane fraction. We also evaluated the activation of the MAP (mitogen-activated protein) kinase pathway by insulin via assay of extracellular signal-regulated kinase (ERK) phosphorylation (Supplementary Figure S3). Knockdown of this same collection of exocyst proteins had not effect on the phosphorylation of ERK in response to insulin.
DISCUSSION
Numerous studies have demonstrated that lipid rafts act as organizing centers for insulin signaling in the adipocyte (Watson and Pessin, 2001; Bickel, 2002; Muller, 2002; Saltiel and Pessin, 2002, 2003; Cohen et al., 2003a). The activated insulin receptor specifically catalyzes the tyrosine phosphorylation of certain proteins in lipid rafts, including caveolin (Kimura et al., 2002), APS (Liu et al., 2002), and Cbl (Ribon and Saltiel, 1997). Components of insulin signaling are constitutively localized in lipid rafts, including some or all of the insulin receptor (Gustavsson et al., 1999; Kimura et al., 2002), flotillin (Baumann et al., 2000; Liu et al., 2005), and TC10 (Watson et al., 2001), which is specifically activated by insulin in these microdomains due to the recruitment of the exchange factor C3G via the tyrosine phosphorylation of c-Cbl and Cbl-b (Liu et al., 2003). Disruption of lipid rafts with cholesterol-extracting drugs such as beta-cyclodextrin or by overexpression of dominant-negative forms of caveolin block the actions of insulin, without disrupting other cellular functions (Gustavsson et al., 1999; Parpal et al., 2001; Ros-Baro et al., 2001; Watson et al., 2001; Shigematsu et al., 2003). Moreover, introduction of a modification of TC10 that prevents lipid raft association blocks the activation of the G protein by insulin, as well as the stimulation of glucose uptake in fat cells (Watson et al., 2001). Targeted disruption of the gene encoding the adipose-enriched raft protein caveolin1 also produced insulin resistance, although it remains uncertain whether this resulted from decreased insulin signaling (Cohen et al., 2003b). Taken together, these data suggest that lipid rafts serve as sites for assembly of signaling complexes that play an important role in insulin action.
Why are lipid raft microdomains crucial to the downstream actions of insulin? One possible explanation is that TC10 effectors are targeted to these regions as sites for Glut4 docking and fusion. The exocyst protein Exo70 binds specifically to activated TC10 in an insulin-dependent manner (Inoue et al., 2003). This interaction results in the assembly of components of the exocyst complex, which plays a critical role in the targeting of the Glut4 vesicle to the plasma membrane, perhaps by directing the vesicle to the precise site of fusion. To understand how the exocyst complex is stabilized in these domains, we searched for Sec8 binding partners and identified SAP97, a member of the MAGUKs family of proteins. SAP97 is expressed in lipid rafts in adipocytes, suggesting that by anchoring Sec8 it might stabilize the exocyst complex in these microdomains. In this regard, knockdown of SAP97 disrupted the exocyst complex in lipid rafts and decreased insulin-stimulated translocation of Glut4 to lipid rafts, accompanied by decreased glucose uptake.
The presence of Glut4 in lipid rafts has been controversial (Bickel, 2002). Biochemical isolation and immunogold electron microscopy of plasma membranes revealed the transient localization of Glut4 immunoreactivity in lipid rafts after insulin stimulation of 3T3L1 adipocytes (Scherer et al., 1994; Gustavsson et al., 1996; Karlsson et al., 2002), although others found no evidence for Glut4 in lipid rafts (Voldstedlund et al., 1993; Kandror et al., 1995). Although this discrepancy might be the result of different methodologies, it seems possible that Glut4 initially docks and fuses at lipid rafts, due to the assembly of the exocyst in this microdomain, and then transits into nonraft domains. This idea is supported by data presented in Figure 3, in which lipid raft-association of Glut4 tended to precede the appearance of nonraft transporter. On the other hand, it is also possible that there are two populations of Glut4-containing vesicles, a rapidly docking pool that is directed to lipid rafts and another slower pool targeted to nonlipid rafts. In either case, the temporal and spatial compartmentalization of the exocyst complex to lipid raft subdomains appears to be critical to the docking and fusion of Glut4 at the cell surface.
Acknowledgments
We thank Dr. Shu-Chan Hsu (Rutgers University) for the anti-Exo70 mAb, Dr. Benjamin Margolis (University of Michigan) for the YFP-SAP97 construct, and Zhao-Qin Bao for technical assistance. This work was supported by Grants DK61618 and DK60591 from the National Institutes of Health.
Notes
This article was published online ahead of print in MBC in Press (http://www.molbiolcell.org/cgi/doi/10.1091/mbc.E06-01-0030) on March 8, 2006.
The online version of this article contains supplemental material at MBC Online (http://www.molbiolcell.org).
References
- Anderson, R. G. (1998). The caveolae membrane system. Annu. Rev. Biochem. 67, 199-225. [Abstract] [Google Scholar]
- Baumann, C. A., Ribon, V., Kanzaki, M., Thurmond, D. C., Mora, S., Shigematsu, S., Bickel, P. E., Pessin, J. E., and Saltiel, A. R. (2000). CAP defines a second signalling pathway required for insulin-stimulated glucose transport [see comments]. Nature 407, 202-207. [Abstract] [Google Scholar]
- Bickel, P. E. (2002). Lipid rafts and insulin signaling. Am. J. Physiol. Endocrinol. Metab. 282, E1-E10. [Abstract] [Google Scholar]
- Bryant, N. J., Govers, R., and James, D. E. (2002). Regulated transport of the glucose transporter GLUT4. Nat. Rev. Mol. Cell. Biol. 3, 267-277. [Abstract] [Google Scholar]
- Chamberlain, L. H., and Gould, G. W. (2002). The v-and t-SNARE proteins that mediate Glut4 vesicle fusion are localised in detergent-insoluble lipid rafts present on distinct intracellular membranes. J. Biol. Chem. 9, 9. [Abstract] [Google Scholar]
- Chiang, S. H., Baumann, C. A., Kanzaki, M., Thurmond, D. C., Watson, R. T., Neudauer, C. L., Macara, I. G., Pessin, J. E., and Saltiel, A. R. (2001). Insulin-stimulated GLUT4 translocation requires the CAP-dependent activation of TC10. Nature 410, 944-948. [Abstract] [Google Scholar]
- Chiang, S. H., Hou, J. C., Hwang, J., Pessin, J. E., and Saltiel, A. R. (2002). Cloning and functional characterization of related TC10 isoforms, a subfamily of Rho proteins involved in insulin-stimulated glucose transport. J. Biol. Chem. 277, 13067-13073. [Abstract] [Google Scholar]
- Chunqiu Hou, J., and Pessin, J. E. (2003). Lipid raft targeting of the TC10 amino terminal domain is responsible for disruption of adipocyte cortical actin. Mol. Biol. Cell 14, 3578-3591. Epub 2003 Jul 3525. [Europe PMC free article] [Abstract] [Google Scholar]
- Cohen, A. W., Combs, T. P., Scherer, P. E., and Lisanti, M. P. (2003a). Role of caveolin and caveolae in insulin signaling and diabetes. Am. J. Physiol. Endocrinol. Metab. 285, E1151-E1160. [Abstract] [Google Scholar]
- Cohen, A. W., Razani, B., Wang, X. B., Combs, T. P., Williams, T. M., Scherer, P. E., and Lisanti, M. P. (2003b). Caveolin-1-deficient mice show insulin resistance and defective insulin receptor protein expression in adipose tissue. Am. J. Physiol. Cell Physiol. 285, C222-C235. Epub 2003 Mar 2026. [Abstract] [Google Scholar]
- Folco, E. J., Liu, G. X., and Koren, G. (2004). Caveolin-3 and SAP97 form a scaffolding protein complex that regulates the voltage-gated potassium channel Kv1.5. Am. J. Physiol. Heart Circ. Physiol. 287, H681-H690. [Abstract] [Google Scholar]
- Funke, L., Dakoji, S., and Bredt, D. S. (2005). Membrane-associated guanylate kinases regulate adhesion and plasticity at cell junctions. Annu. Rev. Biochem. 74, 219-245. [Abstract] [Google Scholar]
- Guo, W., and Novick, P. (2004). The exocyst meets the translocon: a regulatory circuit for secretion and protein synthesis? Trends Cell Biol. 14, 61-63. [Abstract] [Google Scholar]
- Gustavsson, J., Parpal, S., Karlsson, M., Ramsing, C., Thorn, H., Borg, M., Lindroth, M., Peterson, K. H., Magnusson, K. E., and Stralfors, P. (1999). Localization of the insulin receptor in caveolae of adipocyte plasma membrane. FASEB J. 13, 1961-1971. [Abstract] [Google Scholar]
- Gustavsson, J., Parpal, S., and Stralfors, P. (1996). Insulin-stimulated glucose uptake involves the transition of glucose transporters to a caveolae-rich fraction within the plasma membrane: implications for type II diabetes. Mol. Med. 2, 367-372. [Europe PMC free article] [Abstract] [Google Scholar]
- Hsu, S. C., TerBush, D., Abraham, M., and Guo, W. (2004). The exocyst complex in polarized exocytosis. Int. Rev. Cytol. 233, 243-265. [Abstract] [Google Scholar]
- Inoue, M., Chang, L., Hwang, J., Chiang, S. H., and Saltiel, A. R. (2003). The exocyst complex is required for targeting of Glut4 to the plasma membrane by insulin. Nature 422, 629-633. [Abstract] [Google Scholar]
- Kandror, K. V., Stephens, J. M., and Pilch, P. F. (1995). Expression and compartmentalization of caveolin in adipose cells: coordinate regulation with and structural segregation from GLUT4. J. Cell Biol. 129, 999-1006. [Europe PMC free article] [Abstract] [Google Scholar]
- Kanzaki, M., and Pessin, J. E. (2002). Caveolin-associated filamentous actin (Cav-actin) defines a novel F-actin structure in adipocytes. J. Biol. Chem. 277, 25867-25869. Epub 22002 May 25830. [Abstract] [Google Scholar]
- Karlsson, M., Thorn, H., Parpal, S., Stralfors, P., and Gustavsson, J. (2002). Insulin induces translocation of glucose transporter GLUT4 to plasma membrane caveolae in adipocytes. FASEB J. 16, 249-251. Epub 2001 Dec 2014. [Abstract] [Google Scholar]
- Kee, Y., Yoo, J. S., Hazuka, C. D., Peterson, K. E., Hsu, S. C., and Scheller, R. H. (1997). Subunit structure of the mammalian exocyst complex. Proc. Natl. Acad. Sci. USA 94, 14438-14443. [Europe PMC free article] [Abstract] [Google Scholar]
- Kimura, A., Baumann, C. A., Chiang, S. H., and Saltiel, A. R. (2001). The sorbin homology domain: a motif for the targeting of proteins to lipid rafts. Proc. Natl. Acad. Sci. USA 98, 9098-9103. [Europe PMC free article] [Abstract] [Google Scholar]
- Kimura, A., Mora, S., Shigematsu, S., Pessin, J. E., and Saltiel, A. R. (2002). The insulin receptor catalyzes the tyrosine phosphorylation of caveolin-1. J. Biol. Chem. 277, 30153-30158. Epub 32002 May 30129. [Abstract] [Google Scholar]
- Lipschutz, J. H., and Mostov, K. E. (2002). Exocytosis: the many masters of the exocyst. Curr. Biol. 12, R212-R214. [Abstract] [Google Scholar]
- Liu, J., DeYoung, S. M., Hwang, J. B., O'Leary, E. E., and Saltiel, A. R. (2003). The roles of Cbl-b and c-Cbl in insulin-stimulated glucose transport. J. Biol. Chem. 278, 36754-36762. Epub 32003 Jul 36752. [Abstract] [Google Scholar]
- Liu, J., Deyoung, S. M., Zhang, M., Dold, L. H., and Saltiel, A. R. (2005). The stomatin/prohibitin/flotillin/HflK/C domain of flotillin-1 contains distinct sequences that direct plasma membrane localization and protein interactions in 3T3-L1 adipocytes. J. Biol. Chem. 280, 16125-16134. Epub 12005 Feb 16114. [Abstract] [Google Scholar]
- Liu, J., Kimura, A., Baumann, C. A., and Saltiel, A. R. (2002). APS facilitates c-Cbl tyrosine phosphorylation and GLUT4 translocation in response to insulin in 3T3-L1 adipocytes. Mol. Cell. Biol. 22, 3599-3609. [Europe PMC free article] [Abstract] [Google Scholar]
- Maffucci, T., Brancaccio, A., Piccolo, E., Stein, R. C., and Falasca, M. (2003). Insulin induces phosphatidylinositol-3-phosphate formation through TC10 activation. EMBO J. 22, 4178-4189. [Europe PMC free article] [Abstract] [Google Scholar]
- Muller, G. (2002). Dynamics of plasma membrane microdomains and crosstalk to the insulin signalling cascade. FEBS Lett. 531, 81-87. [Abstract] [Google Scholar]
- Nystrom, F. H., Chen, H., Cong, L. N., Li, Y., and Quon, M. J. (1999). Caveolin-1 interacts with the insulin receptor and can differentially modulate insulin signaling in transfected Cos-7 cells and rat adipose cells. Mol. Endocrinol. 13, 2013-2024. [Abstract] [Google Scholar]
- Parpal, S., Karlsson, M., Thorn, H., and Stralfors, P. (2001). Cholesterol depletion disrupts caveolae and insulin receptor signaling for metabolic control via insulin receptor substrate-1, but not for mitogen-activated protein kinase control. J. Biol. Chem. 276, 9670-9678. [Abstract] [Google Scholar]
- Ribon, V., and Saltiel, A. R. (1997). Insulin stimulates tyrosine phosphorylation of the proto-oncogene product of c-Cbl in 3T3-L1 adipocytes. Biochem. J. 324, 839-845. [Europe PMC free article] [Abstract] [Google Scholar]
- Ros-Baro, A., Lopez-Iglesias, C., Peiro, S., Bellido, D., Palacin, M., Zorzano, A., and Camps, M. (2001). Lipid rafts are required for GLUT4 internalization in adipose cells. Proc. Natl. Acad. Sci. USA 98, 12050-12055. Epub 12001 Oct 12052. [Europe PMC free article] [Abstract] [Google Scholar]
- Saltiel, A. R., and Kahn, C. R. (2001). Insulin signalling and the regulation of glucose and lipid metabolism. Nature 414, 799-806. [Abstract] [Google Scholar]
- Saltiel, A. R., and Pessin, J. E. (2002). Insulin signaling pathways in time and space. Trends Cell Biol. 12, 65-71. [Abstract] [Google Scholar]
- Saltiel, A. R., and Pessin, J. E. (2003). Insulin signaling in microdomains of the plasma membrane. Traffic 4, 711-716. [Abstract] [Google Scholar]
- Sans, N., Prybylowski, K., Petralia, R. S., Chang, K., Wang, Y. X., Racca, C., Vicini, S., and Wenthold, R. J. (2003). NMDA receptor trafficking through an interaction between PDZ proteins and the exocyst complex. Nat. Cell Biol. 5, 520-530. [Abstract] [Google Scholar]
- Scherer, P. E., Lisanti, M. P., Baldini, G., Sargiacomo, M., Mastick, C. C., and Lodish, H. F. (1994). Induction of caveolin during adipogenesis and association of GLUT4 with caveolin-rich vesicles. J. Cell Biol. 127, 1233-1243. [Europe PMC free article] [Abstract] [Google Scholar]
- Schlegel, A., Volonte, D., Engelman, J. A., Galbiati, F., Mehta, P., Zhang, X. L., Scherer, P. E., and Lisanti, M. P. (1998). Crowded little caves: structure and function of caveolae. Cell Signal. 10, 457-463. [Abstract] [Google Scholar]
- Shigematsu, S., Watson, R. T., Khan, A. H., and Pessin, J. E. (2003). The adipocyte plasma membrane caveolin functional/structural organization is necessary for the efficient endocytosis of GLUT4. J. Biol. Chem. 278, 10683-10690. Epub 12002 Dec 10620. [Abstract] [Google Scholar]
- Taylor, G. S., Maehama, T., and Dixon, J. E. (2000). Inaugural article: myotubularin, a protein tyrosine phosphatase mutated in myotubular myopathy, dephosphorylates the lipid second messenger, phosphatidylinositol 3-phosphate. Proc. Natl. Acad. Sci. USA 97, 8910-8915. [Europe PMC free article] [Abstract] [Google Scholar]
- TerBush, D. R., Maurice, T., Roth, D., and Novick, P. (1996). The Exocyst is a multiprotein complex required for exocytosis in Saccharomyces cerevisiae. EMBO J. 15, 6483-6494. [Europe PMC free article] [Abstract] [Google Scholar]
- Tsuboi, T., Ravier, M. A., Xie, H., Ewart, M. A., Gould, G. W., Baldwin, S. A., and Rutter, G. A. (2005). Mammalian exocyst complex is required for the docking step of insulin vesicle exocytosis. J. Biol. Chem. 280, 25565-25570. [Abstract] [Google Scholar]
- Vainio, S., Heino, S., Mansson, J. E., Fredman, P., Kuismanen, E., Vaarala, O., and Ikonen, E. (2002). Dynamic association of human insulin receptor with lipid rafts in cells lacking caveolae. EMBO Rep. 3, 95-100. Epub 2001 Dec 2019. [Europe PMC free article] [Abstract] [Google Scholar]
- Vega, I. E., and Hsu, S. C. (2001). The exocyst complex associates with microtubules to mediate vesicle targeting and neurite outgrowth. J. Neurosci. 21, 3839-3848. [Europe PMC free article] [Abstract] [Google Scholar]
- Voldstedlund, M., Tranum-Jensen, J., and Vinten, J. (1993). Quantitation of Na+/K(+)-ATPase and glucose transporter isoforms in rat adipocyte plasma membrane by immunogold labeling. J. Membr. Biol. 136, 63-73. [Abstract] [Google Scholar]
- Watson, R. T., Furukawa, M., Chiang, S. H., Boeglin, D., Kanzaki, M., Saltiel, A. R., and Pessin, J. E. (2003). The exocytotic trafficking of TC10 occurs through both classical and nonclassical secretory transport pathways in 3T3L1 adipocytes. Mol. Cell. Biol. 23, 961-974. [Europe PMC free article] [Abstract] [Google Scholar]
- Watson, R. T., and Pessin, J. E. (2001). Subcellular compartmentalization and trafficking of the insulin-responsive glucose transporter, GLUT4. Exp. Cell Res. 271, 75-83. [Abstract] [Google Scholar]
- Watson, R. T., Shigematsu, S., Chiang, S. H., Mora, S., Kanzaki, M., Macara, I. G., Saltiel, A. R., and Pessin, J. E. (2001). Lipid raft microdomain compartmentalization of TC10 is required for insulin signaling and GLUT4 translocation. J. Cell Biol. 154, 829-840. [Europe PMC free article] [Abstract] [Google Scholar]
- Yamamoto, M., Toya, Y., Schwencke, C., Lisanti, M. P., Myers, M. G., Jr., and Ishikawa, Y. (1998). Caveolin is an activator of insulin receptor signaling. J. Biol. Chem. 273, 26962-26968. [Abstract] [Google Scholar]
- Yeaman, C. (2003). Ultracentrifugation-based approaches to study regulation of Sec6/8 (exocyst) complex function during development of epithelial cell polarity. Methods 30, 198-206. [Abstract] [Google Scholar]
Articles from Molecular Biology of the Cell are provided here courtesy of American Society for Cell Biology
Full text links
Read article at publisher's site: https://doi.org/10.1091/mbc.e06-01-0030
Read article for free, from open access legal sources, via Unpaywall:
https://europepmc.org/articles/pmc1446102?pdf=render
Citations & impact
Impact metrics
Citations of article over time
Article citations
DLG1 functions upstream of SDCCAG3 and IFT20 to control ciliary targeting of polycystin-2.
EMBO Rep, 25(7):3040-3063, 07 Jun 2024
Cited by: 0 articles | PMID: 38849673 | PMCID: PMC11239879
An isoform-specific function of Cdc42 in regulating mammalian Exo70 during axon formation.
Life Sci Alliance, 6(3):e202201722, 21 Dec 2022
Cited by: 3 articles | PMID: 36543541 | PMCID: PMC9772827
GPR125 (ADGRA3) is an autocleavable adhesion GPCR that traffics with Dlg1 to the basolateral membrane and regulates epithelial apicobasal polarity.
J Biol Chem, 298(10):102475, 09 Sep 2022
Cited by: 3 articles | PMID: 36089063 | PMCID: PMC9539791
Genetic differentiation in East African ethnicities and its relationship with endurance running success.
PLoS One, 17(5):e0265625, 19 May 2022
Cited by: 4 articles | PMID: 35588128 | PMCID: PMC9119534
Coupled sterol synthesis and transport machineries at ER-endocytic contact sites.
J Cell Biol, 220(10):e202010016, 20 Jul 2021
Cited by: 13 articles | PMID: 34283201 | PMCID: PMC8294947
Go to all (80) article citations
Data
Data behind the article
This data has been text mined from the article, or deposited into data resources.
BioStudies: supplemental material and supporting data
Similar Articles
To arrive at the top five similar articles we use a word-weighted algorithm to compare words from the Title and Abstract of each citation.
The exocyst complex is required for targeting of Glut4 to the plasma membrane by insulin.
Nature, 422(6932):629-633, 01 Apr 2003
Cited by: 226 articles | PMID: 12687004
Phosphorylation of the exocyst protein Exo84 by TBK1 promotes insulin-stimulated GLUT4 trafficking.
Sci Signal, 10(471):eaah5085, 21 Mar 2017
Cited by: 20 articles | PMID: 28325821
Insulin-stimulated GLUT4 translocation requires the CAP-dependent activation of TC10.
Nature, 410(6831):944-948, 01 Apr 2001
Cited by: 343 articles | PMID: 11309621
Insulin signaling: GLUT4 vesicles exit via the exocyst.
Curr Biol, 13(14):R574-6, 01 Jul 2003
Cited by: 34 articles | PMID: 12867054
Review
Funding
Funders who supported this work.
NIDDK NIH HHS (4)
Grant ID: R01 DK060591
Grant ID: R01 DK061618
Grant ID: DK60591
Grant ID: DK61618