Abstract
Free full text

Enterolysin A, a Cell Wall-Degrading Bacteriocin from Enterococcus faecalis LMG 2333
Abstract
A novel antimicrobial protein, designated enterolysin A, was purified from an Enterococcus faecalis LMG 2333 culture. Enterolysin A inhibits growth of selected enterococci, pediococci, lactococci, and lactobacilli. Antimicrobial activity was initially detected only on solid media, but by growing the bacteria in a fermentor under optimized production conditions (MRS broth with 4% [wt/vol] glucose, pH 6.5, and a temperature between 25 and 35°C), the bacteriocin activity was increased to 5,120 bacteriocin units ml−1. Enterolysin A production was regulated by pH, and activity was first detected in the transition between the logarithmic and stationary growth phases. Killing of sensitive bacteria by enterolysin A showed a dose-response behavior, and the bacteriocin has a bacteriolytic mode of action. Enterolysin A was purified, and the primary structure was determined by combined amino acid and DNA sequencing. This bacteriocin is translated as a 343-amino-acid preprotein with an sec-dependent signal peptide of 27 amino acids, which is followed by a sequence corresponding to the N-terminal part of the purified protein. Mature enterolysin A consists of 316 amino acids and has a calculated molecular weight of 34,501, and the theoretical pI is 9.24. The N terminus of enterolysin A is homologous to the catalytic domains of different cell wall-degrading proteins with modular structures. These include lysostaphin, ALE-1, zoocin A, and LytM, which are all endopeptidases belonging to the M37 protease family. The N-terminal part of enterolysin A is linked by a threonine-proline-rich region to a putative C-terminal recognition domain, which shows significant sequence identity to two bacteriophage lysins.
Bacteriocins are antimicrobial peptides or proteins that inhibit growth of bacteria closely related to the producing organism. Antimicrobial compounds from archaea and gram-negative and gram-positive bacteria have been characterized, and especially bacteriocins of lactic acid bacteria (LAB) have received a great deal of attention due to their preservative effects in food systems. Members of most LAB genera, including lactobacilli, lactococci, pediococci, leuconostocs, carnobacteria, streptococci, and enterococci, produce bacteriocins. Almost all bacteriocins characterized so far have been purified from culture supernatants, and the amino acid sequences have been obtained and used for reverse genetic studies. However, in many strains bacteriocin production can only be detected on solid media, which has made biochemical and genetic characterization difficult (3, 12, 32). This suggests that bacteriocin production is regulated in these strains, and information about regulation of bacteriocin production is vital for obtaining more knowledge about these antimicrobial compounds. This is especially important from an applied point of view if a bacteriocin is to be produced on a large scale.
Bacteriocins from LAB are currently classified into three different major classes (33). Class I bacteriocins, the lantibiotics, are small peptides that undergo extensive posttranslational modifications. Class II bacteriocins are unmodified, heat-stable peptides. Class III bacteriocins, the least well characterized so far, are large and heat-labile antimicrobial proteins. Large bacteriocins are produced by many members of the Archaea and Bacteria (7, 11, 42, 50, 51). Among these molecules are the well-characterized colicins produced by Escherichia coli (30). These bacteriocins have a domain-type structure, in which different domains have functions for translocation, receptor binding, and lethal activity (43). However, only three class III bacteriocins from LAB have been characterized genetically to date. Helveticin J is a chromosomally encoded bacteriocin that has a narrow inhibitory spectrum and is produced by Lactobacillus helveticus 481 (26). This bacteriocin is a 37,511-Da heat-labile protein with a bactericidal mode of action. Zoocin A, produced by Streptococcus zooepidemicus 4881, has activity against other streptococci (46, 47). This compound is a protein containing 262 amino acids organized in distinct domains with different functions. Millericin B, a 259-amino-acid bacteriocin produced by Streptococcus milleri NMSCC 061, has a broader antimicrobial spectrum, which includes streptococci, listeriae, and lactococci (4, 5, 46, 47). Both zoocin A and millericin B show homology with the catalytic domains of lytic proteins from both gram-negative and gram-positive bacteria, and their mechanism of action is hydrolysis of specific peptide bonds in the stem and/or interpeptide bridges in the peptidoglycans of susceptible bacteria.
In this paper we describe a novel type III bacteriocin produced by Enterococcus faecalis LMG 2333. This bacteriocin, designated enterolysin A, is a heat-labile protein with a broad inhibitory spectrum, which breaks down the cell walls of sensitive bacteria.
MATERIALS AND METHODS
Bacterial strains, media, and growth conditions.
E. faecalis strain LMG 2333 was propagated in MRS broth (Difco Laboratories, Detroit, Mich.) at 30 or 37°C with an initial pH of 6.3. For production studies the bacteria were grown in a stirred (200 rpm) fermentor (working volume, 1,000 ml; Ingold) with MRS broth containing 4% (wt/vol) glucose. The pH of the culture broth was adjusted with hydrochloric acid or sodium hydroxide prior to sterilization, and the pH was kept constant during the fermentation by addition of 3 M sodium hydroxide. Bacterial growth was monitored by determining the optical density at 600 nm (OD600) (path length, 1 cm; UV-160 UV-visible spectrophotometer; Shimadsu, Kyoto, Japan). The indicator strains used to assay bacteriocin activity obtained in the fermentations were Lactococcus lactis IL 1403 and a streptomycin-resistant variant of this strain, L. lactis IL 1403Str. They were grown in M17 broth (Oxoid, Unipath Ltd., Basingstoke, Hampshire, England) supplemented with 0.5% (wt/vol) glucose (GM17) at 30°C. L. lactis IL 1403Str was grown in the presence of 500 μg of streptomycin ml−1. The different strains tested for sensitivity to the bacteriocin, the corresponding media, and the incubation temperatures are described in Table Table11.
TABLE 1.
Activities of E. faecalis LMG 2333 against gram-positive bacteria
Microorganism | Cultivation medium | Incubation temp (°C) | Sensitivity to enterolysin Aa |
---|---|---|---|
Lactobacillus sake NCDO 2714 | MRS | 30 | +++ |
L. fermentum NCDO 1750 | MRS | 30 | − |
L. brevis C136 | MRS | 30 | + |
L. curvatus NCDO | MRS | 30 | ++ |
L. plantarum C11 | MRS | 30 | − |
L. plantarum 965 | MRS | 30 | − |
L. acidophilus NCDO 860 | MRS | 30 | − |
Lactococcus cremoris CNRZ 117B | GM17 | 30 | +++ |
L. lactis IL 1403 | GM17 | 30 | ++ |
L. lactis IL 1403Str | GM17 | 30 | ++ |
L. lactis MG1614 | GM17 | 30 | +++ |
Leuconostoc lactis NCDO 533 | MRS | 30 | − |
Carnobacterium piscicola NCDO 2762 | MRS | 30 | − |
Pediococcus pentosaceus FBB 611 | MRS | 30 | ++ |
P. acidilactici PAC1.0 | MRS | 30 | ++ |
Enterococcus faecium CTC492 | MRS | 30 | ++ |
E. faecalis EF Bridge | MRS | 30 | (+) |
Listeria innocua BL86/26B | BHIb | 37 | (+) |
L. innocua LMG2785 | BHI | 37 | (+) |
L. ivanovii Li 4 | BHI | 37 | (+) |
Bacillus subtilis BD630 | BHI | 37 | (+) |
B. cereus ATCC 9139B | BHI | 37 | (+) |
Staphylococcus carnosus MC1.B | GM17 | 37 | (+) |
Propionibacterium jensenii ATCC 4868 | GM17 | 30 | (+) |
P. freudenreichii LMG 2792 | GM17 | 30 | − |
P. acidipropionici ATCC 4965 | GM17 | 30 | − |
P. thoenii LMG 2790 | GM17 | 30 | − |
Clostridium butyricum NCDO 855A | TGMc | 37 | − |
C. sporogenes NCDO 1791 | TGM | 37 | − |
C. bifermentans NCDO 1711 | TGM | 37 | − |
C. tyrobutyricum NCDO 1754 | TGM | 37 | − |
PCR and DNA sequencing.
DNA from E. faecalis LMG 2333 was isolated by using Advamax beads (Advanced Genetic Technologies Corp., Gaithersburg, Md.). Restriction enzymes and other DNA-modifying enzymes were used as recommended by the manufacturer (Promega, Madison, Wis.). The PCR was performed with Taq polymerase (Advanced Biotechnologies Ltd., London, United Kingdom) by using a model PTC-150 minicycler (MJ Research Inc., Watertown, Mass.). Amplification and sequencing of the 16S rRNA gene were performed as described by Utåker et al. (49). Primers were removed from the PCR mixtures prior to sequencing by using a QIAquick PCR purification kit (QIAGEN GmbH, Hilden, Germany). Two degenerate oligonucleotide primers were constructed on the basis of the amino acid sequence of enterolysin A and were used in PCR to generate a 117-bp DNA fragment. Further sequence data were obtained by a primer walking strategy by using DNA cut with various restriction enzymes ligated to pBluescript digested with enzymes that created compatible cohesive ends as described by Casaus et al. (9). The restriction enzymes used were DraI, PvuII, SspI, Sau3A, and ScaI. The PCR fragments of interest were purified by agarose gel electrophoresis and extracted with a Qiaex II gel extraction kit (QIAGEN GmbH). Sequencing was performed with an ABI PRISM 377 DNA sequencer (Perkin-Elmer) by using an ABI Prism dye terminator cycle sequencing Ready Reaction kit (Perkin-Elmer). Identity searches were done by performing an advanced BLAST search (1).
Electrophoretic protein pattern of strain LMG 2333.
The protein profile of strain LMG 2333 was determined by analyzing a cell extract by sodium dodecyl sulfate (SDS)-polyacrylamide gel electrophoresis (PAGE). Identification of the strain was performed by comparing the profile with protein profiles of previously described bacteria (37). The protein profile experiments and identification of strain LMG 2333 were performed by Marc Vancanneyt, University of Ghent, BCCM/LMG Culture Collection, Ghent, Belgium.
Bacteriocin activity assays.
Bacteriocin activity was detected by using the overlay assay described by Cintas et al. (12). Agar plates with indicator bacteria were incubated at appropriate temperatures (Table (Table1)1) and examined for zones of growth inhibition. Bacteriocin activity was expressed in bacteriocin units (BU) per milliliter for activity against sensitive bacteria and was determined by the microtiter plate assay (24). The cell-free culture supernatants were not heat treated as described in the original method due to the heat lability of the bacteriocin (24). Streptomycin (500 μg ml−1) was included in the medium to avoid growth of the producer, and L. lactis IL 1403Str was used as the indicator organism when bacteriocin activity was assayed in the fermentor studies.
Purification of enterolysin A.
Enterolysin A (EnlA) was purified from the supernatant of a 2-liter E. faecalis LMG 2333 culture propagated for 24 h at a constant pH of 6.5 and 30°C. Proteins were precipitated with ammonium sulfate (40%, wt/vol) and harvested by centrifugation (10,000 × g, 4°C, 45 min). The protein pellet was dissolved in 130 ml of 8 M urea in 10 mM sodium phosphate buffer (pH 7) (buffer A), which yielded crude enterolysin A. The sample was concentrated to 37.5 ml by using a Centriprep-3 concentrator filter (Amicon, Inc., Beverly, Mass.) and then applied to a cation-exchange column (25 by 25 mm) of SP-Sepharose (Amersham Pharmacia Biotech, Uppsala, Sweden) equilibrated with buffer A. After the column was washed with 30 ml of buffer A, the active fraction was eluted with 5 ml of buffer B (1 M sodium chloride in buffer A). The eluate was diluted 10-fold in buffer A and applied to a Mono-S HR 5/5 cation-exchange column connected to a fast protein liquid chromatography system (Amersham Pharmacia Biotech). A 0 to 40% buffer B gradient in 10 ml was used for elution. The pure bacteriocin eluted as a single peak after rechromatography of the active fractions. Urea, phosphate, and sodium chloride were removed from the sample by gel filtration by using a Superdex peptide HR 10/30 column from Amersham Pharmacia Biotech. Water was used as the mobile phase.
SDS-PAGE.
SDS-PAGE was carried out as described by Laemmli et al. (29), except that the enterolysin samples were not heat treated prior to electrophoresis. A Mini-PROTEAN electrophoresis cell (Bio-Rad Laboratories, Hercules, Calif.) and 15% (wt/vol) polyacrylamide separating gels were used. Rainbow colored molecular mass marker (Amersham Biosciences, Freiburg, Germany) consisting of a mixture of proteins having molecular masses ranging from 2.5 to 45 kDa was electrophoresed in parallel with the bacteriocin samples in each gel. To demonstrate lytic activity, autoclaved cells of L. lactis IL 1403 (0.7%, wt/vol) were incorporated into one gel as a substrate (31, 38). Following electrophoresis, the gel was washed for 1 h in 200 ml of water and examined for clearing zones after incubation for 1 h in 10 mM sodium phosphate (pH 6.9). A parallel gel without dead cells was stained with Coomassie brilliant blue R-250 (Sigma).
Amino acid sequencing.
The N-terminal amino acid sequence of enterolysin A was determined by Edman degradation by using a 477A automatic sequencer (Applied Biosystems, Foster City, Calif.) with an on-line 120-A phenylthiohydantoin amino acid analyzer, as described previously (14).
Effect of enterolysin A on the viability of L. lactis IL 1403Str.
An overnight culture of L. lactis IL 1403Str was diluted in fresh GM17 to a concentration of 105 CFU ml−1. Crude bacteriocin was added, and the culture was incubated at 30°C. Three different bacteriocin activities were tested in addition to a control culture without bacteriocin. At appropriate time intervals, the number of viable bacteria was determined by dilution and plating on GM17 agar plates.
Assay for lytic activity.
Lytic activity was monitored by determining the rate of decrease in the turbidity of a cell suspension of L. lactis IL 1403Str as described previously (48). The bacteria were harvested in the exponential growth phase and resuspended in 10 mM phosphate buffer (pH 7) to halt further growth. The OD600 was approximately 0.34 (3 × 108 to 4 × 108 CFU ml−1). Aliquots were supplemented with crude enterolysin A, and the mixtures were incubated further at 30°C. The turbidities of the cultures were measured spectrophotometrically at 600 nm at appropriate time intervals (path length, 1 cm; UV-160 UV-visible spectrophotometer; Shimadsu). The effect of diethyl pyrocarbonate (DEPC) on the lytic activity of enterolysin A was determined under standard assay conditions. The DEPC stock solution was dissolved in ethanol (96%) to obtain a final concentration of 10% (vol/vol).
Isolation of cell walls and assay for muralytic activity.
Cell walls from L. lactis IL 1403, E. faecium CTC492, and Listeria innocua BL86/26B were prepared by using the following method. Cells from 200 ml of a culture were harvested by centrifugation at 14,000 × g for 15 min at 4°C. The cell pellet was resuspended in 10 ml of 5 mM sodium phosphate buffer (pH 7). The bacteria were disrupted by three passages through a refrigerated French pressure cell (Carver Laboratory Press; Fred S. Carver Inc., Menomonee Falls, Wis.) at 15,000 lb/in2. Whole cells were removed by centrifugation at 1,000 × g for 15 min at 4°C. The cell walls were obtained from the supernatant by centrifugation at 14,000 × g for 15 min at 4°C and washed in 10 ml of potassium buffer (pH 7). SDS was added to a final concentration of 4% (wt/vol), and the mixture was stirred for 90 min at room temperature before it was heated at 100°C for 15 min. The cell walls were harvested by centrifugation at 14,000 × g for 15 min at 20°C, resuspended in 10 ml of 0.1% (vol/vol) Triton X-100, and stirred for 30 min at room temperature before the preparation was centrifuged again at 14,000 × g for 15 min at 20°C. The final pellet was resuspended in 1 ml (total volume). For the assay for muralytic activity the cell wall suspensions were diluted in 5 mM sodium phosphate (pH 7) to an OD600 of 0.3 to 0.4. One-milliliter aliquots were supplemented with 30 BU per ml, and the OD600 was monitored during incubation at room temperature.
Nucleotide sequence accession number.
The nucleotide sequence of enterolysin A determined in this study has been deposited in the GenBank nucleotide data bank under accession number AF249740.
RESULTS
Identification of bacteriocinogenic strain LMG 2333.
Strain LMG 2333 was isolated from fish in Iceland and was chosen for further study due to its clear zones of growth inhibition in overlay assays and its activity against a broad range of bacteria. The 16S ribosomal DNA (rDNA) sequence of this strain showed 99.7% identity to the 16S rRNA gene sequence of E. faecalis (36) and 99.4% identity to the 16S rDNA gene sequence of another strain of E. faecalis (8) in a 1,480-bp stretch. On the basis of the protein profile of strain LMG 2333 we identified this isolate as E. faecalis (level of similarity, 96%), which was consistent with the 16S rDNA data (results not shown).
Optimization of bacteriocin production.
Initially, bacteriocin production was observed only on solid media in overlay assays and not in culture supernatants. In order to obtain amounts sufficient for biochemical work, the conditions for optimal bacteriocin production were established. In batch culture without pH control and at 37°C, E. faecalis LMG 2333 grew to an optical density of 2, and the final pH of the culture was about 4.7. By using the sensitive strain L. lactis IL 1403Str as the indicator it was possible to detect 40 BU ml−1 in the late logarithmic and stationary growth phases of such cultures. When the bacteriocin producer was propagated at a constant pH of 5.5, the same level of bacteriocin activity was obtained. However, increased bacteriocin production was obtained in cultures grown at constant pHs ranging from 6 to 8.5, and the maximum antimicrobial activity was observed at pH 6.5 (Table (Table2).2). Under all conditions tested, the antimicrobial activity peaked in the transition between the exponential and stationary growth phases (Table (Table22 and Fig. Fig.1A).1A). The influence of temperature on bacteriocin production at pH 6.5 was also studied (Table (Table2).2). Enterolysin A was produced at temperatures ranging from 20 to 45°C, and maximal production (5,120 BU ml−1) was observed at temperatures ranging from 20 to 35°C (Table (Table22).
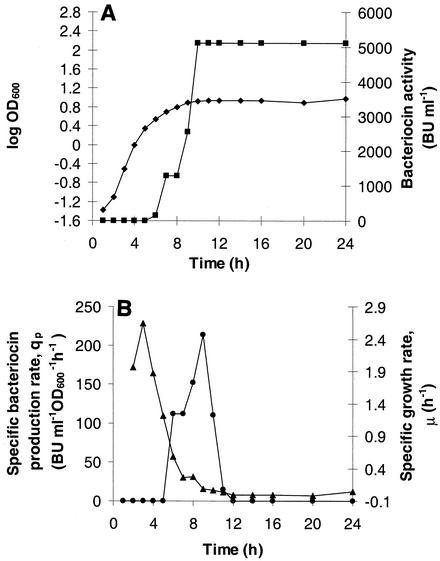
(A) Growth kinetics of E. faecalis LMG 2333 () and production of enterolysin A (
) in MRS broth with 4% (wt/vol) glucose at pH 6.5 and 35°C. The culture was grown in an Ingold fermentor with a 1-liter operating volume by using a 1% inoculum. The bacteriocin activity of cell-free culture supernatants was assayed by using L. lactis IL-1403 and the microtiter plate assay. (B) Specific bacteriocin production rate (qp) (•) and specific growth rate (μ) (
) plotted as functions of time.
TABLE 2.
Maximum bacteriocin activities and maximum bacteriocin specific activities for E. faecalis LMG 2333 cultures at different pH values and temperatures as determined with L. lactis IL 1403 as the indicator
Growth conditions | Maximum bacteriocin activity (BU ml−1) | Maximum bacteriocin sp act (BU ml−1 OD600−1)c | |
---|---|---|---|
pHa | Tempb (°C) | ||
No pH control | 40 | 27 | |
5.5 | 40 | 10 | |
6 | 640 | 94 | |
6.5 | 3,840 | 420 | |
7 | 2,560 | 257 | |
7.5 | 2,560 | 251 | |
8 | 2,560 | 226 | |
8.5 | 2,560 | 253 | |
20 | 2,560 | 307 | |
25 | 5,120 | 548 | |
30 | 5,120 | 602 | |
35 | 5,120 | 607 | |
37 | 3,840 | 420 | |
40 | 2,560 | 351 | |
45 | 320 | 48 |
Figure Figure11 shows growth kinetics and enterolysin A production in MRS medium under optimal production conditions (pH 6.5 and 35°C). Bacteriocin production was first observed in the late exponential growth phase, when the specific growth rate was about one-half the maximal value, and it lasted for about 5 h. The maximum specific production rate, 215 BU ml−1 OD600−1 h−1, was observed when the specific growth rate was reduced to 0.08 h−1 (Fig. (Fig.1B).1B). The bacteriocin activity was stable in cultures of E. faecalis LMG 2333 for most of the conditions tested; the only exceptions were 45°C and pH 8.0 and 8.5, at which the activities were reduced by about 50% at the end of the fermentations (results not shown).
Purification of enterolysin A.
The bacteriocin was purified from a 2-liter culture of E. faecalis LMG 2333 propagated in MRS broth at pH 6.5 and 30°C by using standard chromatographic methods. The bacteriocin activities at different steps in the purification process are shown in Table Table3,3, and the final yield was 2.5%. The bacteriocin eluted from the Mono-S column as a sharp symmetric peak at about 0.2 M sodium chloride (8 M urea in 10 mM phosphate buffer [pH 7]), and the activity coincided with the protein absorbance peak at 280 nm (Fig. (Fig.2).2). The gel-filtered bacteriocin was electrophoresed on a polyacrylamide gel both to test the purity of the sample and to determine the size of the bacteriocin. One band located at about 35,000 Da was observed (Fig. (Fig.3).3). Muralytic activity of enterolysin A, seen as a clear zone, could be detected directly in a polyacrylamide gel supplemented with heat-killed L. lactis IL 1403 cells at a position corresponding to a molecular weight of about 35,000 (Fig. (Fig.33).

Cation-exchange chromatography of enterolysin A. The column used was a Mono-S column from Amersham Pharmacia Biotech. The mobile phase buffer was 10 mM phosphate buffer in 8 M urea (pH 7) with an increasing gradient of sodium chloride. Enterolysin A activity () and absorbance at 280 nm (□) were determined. The dotted line indicates the concentration of sodium chloride.

SDS-PAGE of enterolysin A. Lane 1, molecular weight standard; lane 2, enterolysin A. The gel contained 15% polyacrylamide, and it was stained with Coomassie brilliant blue R-250. Lane 3 shows direct detection of enterolysin as muralytic activity in a 15% polyacrylamide gel with 0.7% (wt/vol) cell walls from L. lactis IL 1403.
TABLE 3.
Stepwise purification of enterolysin A from a culture supernatant of E. faecalis LMG 2333
Fraction | Vol (ml) | Total A280 | Total activity (103 BU) | Sp act (103 BU A280−1) | Increase in sp act (fold) | Yield (%) |
---|---|---|---|---|---|---|
Supernatant | 2,000 | 48,400 | 2,040 | 0.042 | 1 | 100 |
Precipitation with (NH4)2SO4 | 130 | 3,588 | 2,945 | 0.820 | 20 | 144 |
Concentration (Centriprep-3) | 37.5 | 3,050 | 2,850 | 0.934 | 22 | 140 |
Binding to cationic exchanger | ||||||
![]() ![]() ![]() ![]() | 10 | 35.4 | 382 | 10.8 | 257 | 19 |
![]() ![]() ![]() ![]() | 5 | 6.2 | 153 | 24.8 | 590 | 7.5 |
Gel filtration | 1 | 1.4 | 50.5 | 29.7 | 707 | 2.5 |
Heat stability of enterolysin A.
To test the heat stability of the protein, supernatants (pH 6.5) and crude bacteriocin were exposed to 50, 75, and 100°C for 15 min. The bacteriocin activities in the supernatants were 12.5, <1, and <1%, respectively, for the three temperatures tested. The bacteriocin was less heat labile in 8 M urea. No reduction in the activity of the crude bacteriocin was observed at 50°C, and 50 and 2% of the activity were retained at 75 and 100°C, respectively.
Amino acid and DNA sequences of enterolysin A.
The active bacteriocin fraction from the gel filtration step was subjected to Edman degradation. The following sequence was obtained for the N-terminal part of the bacteriocin: ASNEWSWPLGKPYAGRYEEGQQFGNTAFNRGGTYFHDGFDFGSAIYGNNS. Primers based on the corresponding degenerate DNA sequence were constructed, and sequencing of a number of overlapping PCR products finally resulted in determination of the complete DNA sequence encoding enterolysin A and the flanking region. The structural gene for enterolysin A, designated enlA, and flanking DNA are shown in the 1,790-nucleotide sequence shown in Fig. Fig.44 together with the translation product EnlA. With the exception of position 49 in the protein sequence, which is Gly according to the DNA sequence, the nucleotide sequence confirmed the results obtained by amino acid sequencing. The nucleotide sequence further revealed that the bacteriocin is translated with a 27-amino-acid leader peptide, which is cleaved after the Val-Asn-Ala residues (positions 25 to 27). The leader peptide has the characteristics of a typical bacterial signal sequence; it contains one positively charged amino acid in its N-terminal region, followed by a hydrophobic region high in Leu and Val and a neutral but polar C-terminal region. The signal peptide cleavage site predicted by the method of Nielsen et al. (34) corresponded to the NH2-Ala terminus of purified enterolysin A that was determined. The sequence data revealed that mature enterolysin A is a protein consisting of 316 amino acids and has a calculated isoelectric point of 9.24 and a molecular mass of 34,501 Da. The calculated molecular weight corresponds well with the size of enterolysin A determined by SDS-PAGE.
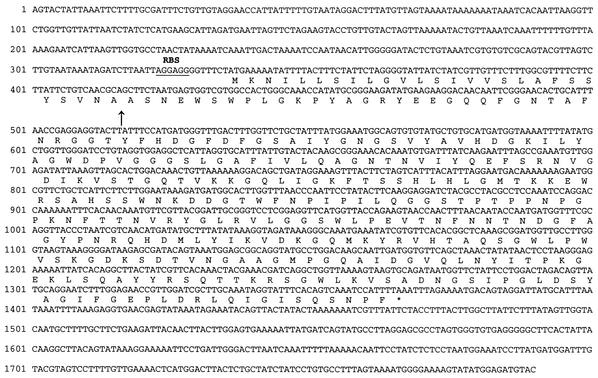
Nucleotide sequence and deduced amino acid sequence of a 1,790-nucleotide fragment from E. faecalis LMG 2333. The deduced amino acid sequence encoded by enlA is shown below the nucleotide sequence. The site where the s-dependent signal peptide is cleaved off is indicated by an arrow, and the putative ribosomal binding site (RBS) is underlined.
The amino acid sequence of enterolysin A showed similarity to the sequences of several proteins in the databases. The N-terminal region of the protein contained a domain found in the M37 family of metallopeptidases, while the C-terminal part showed similarity to different muralytic bacteriophage proteins. The N-terminal part of enterolysin A exhibited 34% identity (54% similarity) with LytM from Staphylococcus aureus in a 93-amino-acid stretch (39), 32% identity (45% similarity) with zoocin A from Streptococcus zooepidemicus 4881 in a 120-amino-acid stretch (47), 29% identity (45% similarity) with lysostaphin from Staphylococcus simulans biovar staphylolyticus in a 124-amino-acid stretch (41), and 29% identity (46% similarity) with ALE-1 from Staphylococcus capitis EPK1 in a 108-amino-acid stretch (48). The sequences homologous to the N-terminal part of enterolysin A were located at the N terminus of lysostaphin and zoocin A but in the C-terminal part of LytM and ALE-1. The homologous regions of these five proteins are aligned in Fig. Fig.5A.5A. The C-terminal part of EnlA is 67% identical (81% similar) to a lysin from Lactobacillus casei bacteriophage A2 (19) and 66% identical (80% similar) to N-acetylmuramoyl-l-alanine amidase from L. casei bacteriophage PL-1, both in the same 148-amino-acid stretch (27) (Fig. (Fig.5B5B).
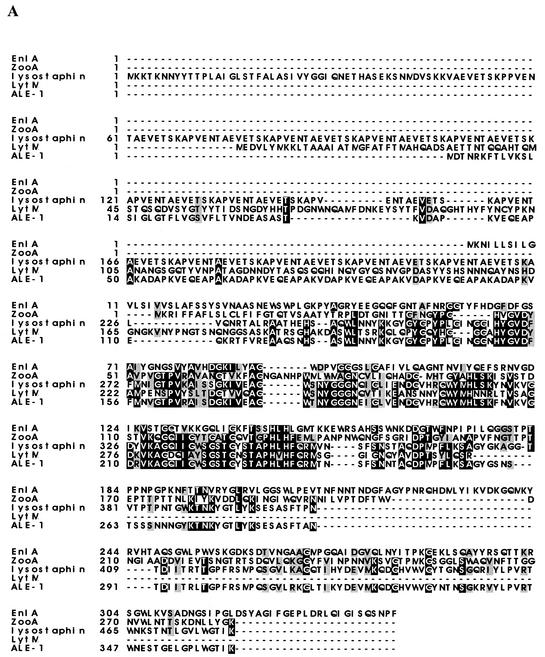

(A) Alignment of enterolysin A with ALE-1 (48), zoocin A (47), lysostaphin (23), and Lyt M (39). (B) Alignment of enterolysin A with bacteriophage A2 lysin (19) and N-acetylmuramoyl-l-alanine amidase from bacteriophage PL-1 (27). Conserved amino acids are indicated by a black background, while similar amino acids are indicated by a gray background.
Inhibitory spectrum of enterolysin A.
A number of bacteria were tested for sensitivity to enterolysin A, both by the overlay assay and by the microtiter plate assay (Table (Table1).1). Three of seven Lactobacillus, all four Lactococcus, two Pediococcus, and one of two Enterococcus strains tested were sensitive in both bacteriocin assays. All Listeria, Bacillus, and Staphylococcus strains tested and one Propionibacterium strain, Propionibacterium jensenii ATCC 4868, showed zones of growth inhibition in the overlay assay, but no growth inhibition was detected in the microtiter plate assay. None of the Leuconostoc, Carnobacterium, or Clostridium strains tested was inhibited by the bacteriocin in either assay. Crude bacteriocin preparations had the same activity spectrum as the supernatant (results not shown). Lactococcus cremoris CNRZ 117B was the most enterolysin A-sensitive strain in both assays. None of the six strains of gram-negative bacteria tested (Aeromonas salmonicida, Vibrio anguilarum, Pseudomonas sp., Pseudomonas fluorescens, Yersinia ruckeri, and Escherichia coli) was sensitive to enterolysin A.
Effect of enterolysin A on sensitive cells.
Cultures of L. lactis IL 1403Str were exposed to three different concentrations of enterolysin A. At different times the number of viable cells was determined by dilution and plate counting (Fig. (Fig.6A).6A). Enterolysin A has a bactericidal mode of action, and the response is dose dependent. The effect of enterolysin A on log-phase L. lactis IL 1403Str in phosphate buffer (approximately 3 × 108 to 4 × 108 CFU/ml) was also investigated to see if the bacteriocin has a lytic mode of action. A rapid decrease in the OD600 (Fig. (Fig.6B)6B) due to lysis of bacteria was observed. Within 30 min the turbidity of the cell suspension was reduced to approximately 20% of the initial turbidity for the highest bacteriocin activity tested (300 BU ml−1). Similar results were obtained for lower bacteriocin activities (30 and 3 BU ml−1), although the reduction in turbidity was slower. For the lowest bacteriocin activity (3 BU ml−1), the turbidity of the cell suspension was reduced to approximately 30% of the initial turbidity within 24 h (results not shown). Similar experiments were done with the enterolysin A-resistant strains Listeria ivanovii Li4 and Bacillus subtilis BD630, but no changes in turbidity were observed within 90 min, even with the highest bacteriocin activity tested (results not shown).
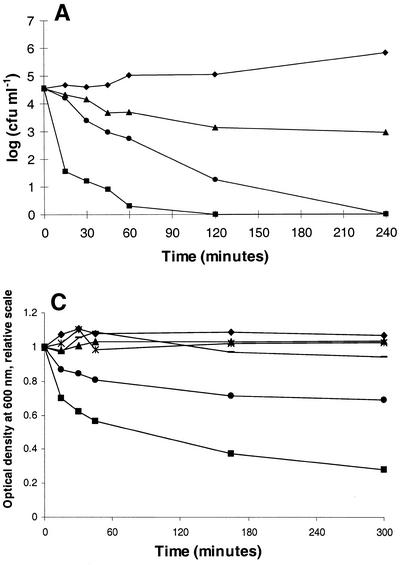
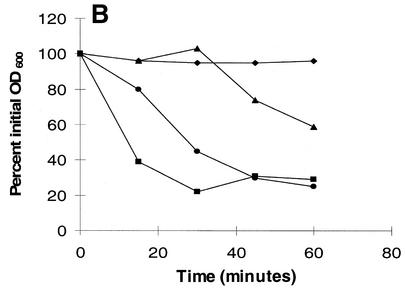
(A) Killing of L. lactis IL-1403Str in GM17 with crude enterolysin A. The number of surviving cells was determined by dilution and cell counting on GM17 agar plates. (B) Bacteriolytic activities of enterolysin A as determined by turbidimetry. Crude bacteriocin fractions were incubated with a 1-ml cell suspension of L. lactis IL 1403Str in 10 mM sodium phosphate buffer (pH 7). The cells were propagated in GM17 with 500 μg of streptomycin ml−1 and harvested in the logarithmic growth phase. The initial optical densities of the cultures were approximately 0.34, and the decreases in turbidity were measured at 600 nm with a spectrophotometer. Symbols: , 3 BU ml−1; •, 30 BU ml−1;
, 300 BU ml−1; ♦, no bacteriocin. (C) Degradation of cell walls with crude enterolysin A (30 BU ml−1). The cell wall fractions were diluted in 0.05 M potassium phosphate buffer (pH 7) to an optical density between 0.3 and 0.4, and the decreases in turbidity were measured spectrophotometrically at 600 nm after the bacteriocin was added. Symbols: ♦, L. lactis IL 1403 control cell walls;
, L. lactis IL 1403 cell walls with enterolysin A;
, E. faecium CTC492 control cell walls; •, E. faecium CTC492 cell walls with enterolysin A;
, L. innocua BL86/26B control cell walls; −, L. innocua BL86/26B cell walls with enterolysin A.
Effect of enterolysin A on cell walls.
Since enterolysin A shows homology to cell wall-degrading proteins that have been shown to lyse bacteria, it was of interest to study its effect directly on isolated cell walls. Cell walls were isolated from two sensitive strains, L. lactis IL 1403 and E. faecium CTC492, and one insensitive strain, L. innocua BL86/26B, and treated with enterolysin A. The optical densities of the cell wall fractions isolated from the two sensitive strains were reduced upon addition of the bacteriocin, showing that the cell wall is indeed the target of the antimicrobial activity (Fig. (Fig.6C).6C). Negative controls, cell walls without added bacteriocin, and the cell walls isolated from the insensitive Listeria strain showed no reduction in turbidity. Together with the zymogram data (Fig. (Fig.3B),3B), these results clearly show that the activity of enterolysin A is muralytic.
DISCUSSION
One aim of the present study was to optimize bacteriocin production in E. faecalis LMG 2333, so that factors crucial for production could be identified and sufficient amounts of the antimicrobial compound could be purified for further characterization. Initially, bacteriocin production by strain LMG 2333 could be detected only on agar plates in overlay assays, and no antimicrobial activity was found in liquid cultures. Production of antimicrobial activity only on solid media has been reported for several LAB (15, 25). Cintas et al. (12) reported that only 12 of 55 isolates of LAB that exhibited antagonistic activities in overlay assays showed detectable bacteriocin activities in liquid cultures. Similar frequencies were reported by Geis et al. (20) for lactococci and by Schillinger and Lücke (44) for lactobacilli.
Previous studies of bacteriocin production in various LAB have shown that bacteriocin production can be influenced by pH, temperature, inoculum size, and other environmental factors (6, 16-18, 32, 35). By growing the cells at a constant pH it was possible to obtain good bacteriocin production in liquid media. The production of bacteriocin by E. faecalis LMG 2333 was highly dependent on the pH of the growth medium; the optimum pH was pH 6.5, and there was very low production at pHs below pH 6. Enterolysin A production does not occur parallel with growth, a production pattern that has been observed for several LAB bacteriocins (16, 17, 35). The bacteriocin seems to exhibit transition state-dependent production, and production is turned on in the late logarithmic or early stationary growth phase. The specific growth rate approaches the minimum value when the specific production rate is maximal. In batch cultures grown without pH control, as in overnight liquid cultures, the pH is below 5.5 when the culture reaches the late exponential growth phase (results not shown). This probably explains why bacteriocin production is low in these cultures, since production is also low in cultures grown at a constant pH of 5.5.
All bacteria utilize cell wall-active enzymes as part of their growth and repair mechanisms. When these enzymes are secreted, they may function catabolically to release nutrients from closely related bacteria in the environment when nutrients become limiting (22, 41, 46). The function of stationary-phase bacteriocin production might also be to kill bacteria in the same environment and thereby reduce the number of bacteria that compete for limiting nutrients.
Enterolysin A is the first bacteriocin from an Enterococcus belonging to class III, the large and heat-labile bacteriocins. To our knowledge, only three class III bacteriocins from LAB have been characterized previously at the genetic level; these are helveticin J from L. helveticus 481 (26), zoocin A from S. zooepidemicus 4881 (47), and millericin B from S. milleri NMSCC 061 (4, 5). Whereas helveticin J exhibits no sequence similarity to enterolysin A, zoocin A and millericin B do. The latter two bacteriocins exert their bactericidal activities by hydrolyzing peptide bonds in the peptidoglycan of susceptible cells. Since EnlA degrades cell walls of sensitive bacteria and exhibits sequence similarity to these bacteriocins, it is likely that EnlA has a similar mode of action.
The sequence analysis of enterolysin A suggested that this bacteriocin consists of two separate domains, an N-terminal catalytic domain and a C-terminal substrate recognition domain. EnlA exhibits identity with cell wall-degrading enzymes produced by different gram-positive bacteria. Three of these enzymes, lysostaphin, ALE-1, and LytM, have been shown to be metalloproteases belonging to the M37 family of proteases (39, 41, 48). In a BlastP search in which the EnlA sequence was used as the query, an M37 domain was identified starting at position 79 and ending at amino acid 155 of the protein (1). Members of this family of proteins have a range of hydrolyzing specificities, and Zn2+ is bound in the catalytic sites of these enzymes. The amino acids involved in binding of the metal ion have not yet been identified with certainty, but Sugai et al. (48) suggested that the His residues at positions 231 and 233 in ALE-1 may serve as the Zn2+ ligands based on similarities to carbonic anhydrase and β-lytic protease from Achromobacter lyticus. These His residues are conserved in all five proteins in the alignment, including enterolysin A, and are found within the M37 domain. In addition, the Zn2+ metalloprotease LasA from Pseudomonas aeruginosa, which can hydrolyze staphylococcal cell walls, has these conserved histidines (His-120 and His-122) (28). Staphylolytic activity of LasA was eliminated when Gustin et al. (21) replaced His-120 with Ala, thereby clearly demonstrating the importance of this His for the enzymatic activity of LasA. DEPC, which chemically modifies His residues, has previously been shown to inhibit the activities of lysostaphin, ALE-1, and LytM (10). Based on sequence similarity and conserved His residues, we hypothesized that DEPC should greatly affect EnlA activity. At a DEPC concentration of 10 mM, EnlA lost the ability to lyse L. cremoris CNRZ 117B (results not shown). This implies that His residues are involved in forming the catalytic site in enterolysin A. The involvement of metal ions in the catalytic site, bound to His residues, seems likely to be due to the conservation of the His motif, but this needs to be investigated further.
Lysostaphin, ALE-1, LytM, and zoocin A are highly specific proteins which lyse only bacteria that belong to the same genus or species as the producer (40, 45, 46, 48). Enterolysin A, however, like millericin B, has a different and broader inhibitory spectrum. The target of the catalytic activities of lysostaphin, ALE-1, LytM, and zoocin A is the pentaglycine interpeptide bridge in the peptidoglycan of susceptible cells, and the catalytic activities in these proteins have been shown to be located in the domain that exhibits homology with the N-terminal part of enterolysin A (40, 41, 46, 48). Wang et al. (52) showed that there is considerable homology between the noncatalytic C-terminal regions of an S. aureus peptidoglycan hydrolase, LytA (N-acetylmuramyl-l-alanine amidase), and lysostaphin. Both proteins bind staphylococcal cell walls, but since their catalytic activities are different, it is likely that these molecules have a modular type of construction and that their C-terminal consensus regions represent the cell wall binding domains. Using hybrid proteins, Baba and Schneewind (2) showed that the C-terminal domain of lysostaphin indeed is responsible for target cell specificity.
The specificity of enterolysin A indicates that the target of its putative recognition domain is different from the other targets described previously. This is consistent with the fact that the putative, noncatalytic C-terminal part of enterolysin A shows no sequence homology to the proteins active against staphylococci and streptococci,. However, the C-terminal domain of EnlA exhibits high levels of sequence identity to a lysin from bacteriophage A2 and an N-acetomuramoyl-l-alanine amidase from bacteriphage PL-1, both of which are bacteriophages of L. casei. The regions of these lysins that are homologous to the C-terminal part of EnlA are thought to be responsible for binding of these enzymes to their cell wall substrates (27).
Another finding that may support the idea that enterolysin A is organized into an N-terminal catalytic domain and a C-terminal cell wall recognition domain is the presence of a short threonine-proline (TP)-rich putative linker sequence (residues 180 to 194; TPTPPNPGPKNFTT) located on the C-terminal side of the putative N-terminal catalytic domain. Similar linker sequences have been identified in many proteins known to exhibit domain structure organization, including lysostaphin and zoocin A (13, 41, 47). Based on the similarities mentioned above, we suggest that enterolysin A is a domain-organized protein, with an N-terminal domain possessing endopeptidase activity, a TP-rich linker region, and a C-terminal recognition domain.
No common denominator has been identified in the interpeptide bridges in the peptidoglycan chains of bacteria sensitive to enterolysin A. However, all the sensitive bacteria have the following stem peptide sequence in their peptidoglycan: l-Ala-d-Glu-l-Lys-d-Ala. This suggests that it is most likely that enterolysin A exerts its activity by hydrolyzing a peptide bond in the stem peptide, suggesting that its activity is similar to that of millericin B (4, 5).
Acknowledgments
T. Nilsen was supported by grant P93154 from The Nordic Industry Fund. H. Holo was supported by grants from the Norwegian Dairy Association, Oslo, Norway.
We thank SINTEF, Applied Chemistry, Department of Biotechnology, Trondheim, Norway, for lending us fermentors and Katinka Quenild Lystad for technical assistance in preparing the fermentors. We are also grateful to Anne May Lønneborg for running the DNA sequencing gels and K. Sletten for performing the amino acid sequencing analysis.
REFERENCES
Articles from Applied and Environmental Microbiology are provided here courtesy of American Society for Microbiology (ASM)
Full text links
Read article at publisher's site: https://doi.org/10.1128/aem.69.5.2975-2984.2003
Read article for free, from open access legal sources, via Unpaywall:
https://aem.asm.org/content/69/5/2975.full.pdf
Citations & impact
Impact metrics
Citations of article over time
Alternative metrics
Article citations
Whole genome sequencing of <i>Lacticaseibacillus casei</i> KACC92338 strain with strong antioxidant activity, reveals genes and gene clusters of probiotic and antimicrobial potential.
Front Microbiol, 15:1458221, 26 Sep 2024
Cited by: 0 articles | PMID: 39391606 | PMCID: PMC11464305
The Enterococcus secretome inhibits the growth of vancomycin-resistant Enterococcus faecalis V853 with their antiproliferative properties and nanoencapsulation effects.
Int Microbiol, 22 May 2024
Cited by: 0 articles | PMID: 38775969
Two Enterococcus faecium Isolates Demonstrated Modulating Effects on the Dysbiosis of Mice Gut Microbiota Induced by Antibiotic Treatment.
Int J Mol Sci, 25(10):5405, 15 May 2024
Cited by: 0 articles | PMID: 38791443 | PMCID: PMC11121104
Comparative Probiogenomics Analysis of Limosilactobacillus fermentum 3872.
Probiotics Antimicrob Proteins, 08 May 2024
Cited by: 0 articles | PMID: 38717735
Molecular Assessments of Antimicrobial Protein Enterocins and Quorum Sensing Genes and Their Role in Virulence of the Genus Enterococcus.
Probiotics Antimicrob Proteins, 04 May 2024
Cited by: 0 articles | PMID: 38703322
Go to all (148) article citations
Data
Data behind the article
This data has been text mined from the article, or deposited into data resources.
BioStudies: supplemental material and supporting data
Nucleotide Sequences
- (1 citation) ENA - AF249740
Similar Articles
To arrive at the top five similar articles we use a word-weighted algorithm to compare words from the Title and Abstract of each citation.
Production of enterolysin A by rumen Enterococcus faecalis strain and occurrence of enlA homologues among ruminal Gram-positive cocci.
J Appl Microbiol, 102(2):563-569, 01 Feb 2007
Cited by: 18 articles | PMID: 17241363
Determination of the mode of action of enterolysin A, produced by Enterococcus faecalis B9510.
J Appl Microbiol, 115(2):484-494, 28 May 2013
Cited by: 21 articles | PMID: 23639072
Isolation and characterization of enterocin SE-K4 produced by thermophilic enterococci, Enterococcus faecalis K-4.
Biosci Biotechnol Biochem, 65(2):247-253, 01 Feb 2001
Cited by: 37 articles | PMID: 11302155
Purification and characterization of enterocin 4, a bacteriocin produced by Enterococcus faecalis INIA 4.
Appl Environ Microbiol, 62(11):4220-4223, 01 Nov 1996
Cited by: 27 articles | PMID: 8900014 | PMCID: PMC168244