Abstract
Free full text

SHED: Stem cells from human exfoliated deciduous teeth
Abstract
To isolate high-quality human postnatal stem cells from accessible resources is an important goal for stem-cell research. In this study we found that exfoliated human deciduous tooth contains multipotent stem cells [stem cells from human exfoliated deciduous teeth (SHED)]. SHED were identified to be a population of highly proliferative, clonogenic cells capable of differentiating into a variety of cell types including neural cells, adipocytes, and odontoblasts. After in vivo transplantation, SHED were found to be able to induce bone formation, generate dentin, and survive in mouse brain along with expression of neural markers. Here we show that a naturally exfoliated human organ contains a population of stem cells that are completely different from previously identified stem cells. SHED are not only derived from a very accessible tissue resource but are also capable of providing enough cells for potential clinical application. Thus, exfoliated teeth may be an unexpected unique resource for stem-cell therapies including autologous stem-cell transplantation and tissue engineering.
Postnatal stem cells have been isolated from a variety of tissues including but not limited to bone marrow, brain, skin, hair follicles, skeletal muscle, and dental pulp (1–7). Recently, the extraordinary plasticity of postnatal stem cells has been suggested, in which neural stem cells may contribute to blood and skeletal muscle (8, 9), and bone marrow stem cells may contribute to muscle, liver, and neuronal tissue (10–13). Recent emerging evidence suggests that cell-fusion events may account for some of these observations (14, 15). It is necessary to gain further insight into the characteristics of postnatal stem cells and examine their full developmental potential in vivo (16).
The transition from deciduous teeth to adult permanent teeth is a very unique and dynamic process in which the development and eruption of permanent teeth coordinate with the resorption of the roots of deciduous teeth. It may take >7 years in humans to complete the ordered replacement of 20 deciduous teeth (17). In this study we isolated a distinctive population of multipotent stem cells from the remnant pulp of exfoliated deciduous teeth. The significance of this study is that it provides evidence indicating that a naturally occurring exfoliated deciduous tooth is similar in some ways to an umbilical cord, containing stem cells that may offer a unique stem-cell resource for potential clinical applications.
Materials and Methods
Subjects and Cell Culture.
Normal exfoliated human deciduous incisors were collected from 7- to 8-year-old children under approved guidelines set by the National Institutes of Health Office of Human Subjects Research. The pulp was separated from a remnant crown and then digested in a solution of 3 mg/ml collagenase type I (Worthington Biochem, Freehold, NJ) and 4 mg/ml dispase (Roche Molecular Biochemicals) for 1 h at 37°C. Single-cell suspensions were cultured in a regular medium as reported (7). These techniques resulted in a population that we have termed stem cells from human exfoliated deciduous teeth (SHED).
Conditions for the induction of calcium accumulation were as reported (7), and recombinant human BMP-4 (R & D Systems) was used to induce osteogenic differentiation. Calcium accumulation was detected by 2% Alizarin red S (pH 4.2) staining. The calcium concentration was measured by using a commercially available kit (calcium kit 587-A, Sigma). The induction of adipogenesis was performed as reported (18). For neural differentiation, Neurobasal A (GIBCO/BRL), B27 supplement (GIBCO/BRL), 1% penicillin, 20 ng/ml epidermal growth factor (BD Bioscience), and 40 ng/ml fibroblast growth factor (FGF) (BD Bioscience) were used to culture cells attached to 0.1% gelatin-coated dishes (StemCell Technologies, Vancouver). For sphere-like cell-cluster formation, 3% rat serum and B27 were added.
Antibodies.
Rabbit antibodies included anti-HSP90 and basic FGF (bFGF) (Santa Cruz Biotechnology); anti-core-binding factor, runt domain, α subunit 1 (CBFA1) (Oncogene Research Products, Cambridge, MA); anti-endostatin, human-specific mitochondria, and glutamic acid decarboxylase (GAD) (Chemicon); and anti-alkaline phosphatase (ALP) (LF-47), bone sialoprotein (LF-120), matrix extracellular phosphaglycoprotein (MEPE) (LF-155), and dentin sialophosphoprotein (DSPP) (LF-151) (National Institute of Dental and Craniofacial Research/National Institutes of Health). Goat antibodies included anti-MAP2 and Tau (Santa Cruz Biotechnology). Mouse antibodies included anti-STRO-1 and CD146 (CC9); glial fibrillary acidic protein (GFAP), nestin, neurofilament M (NFM), neuronal nuclei (NeuN), and 2′,3′-cyclic nucleotide-3′-phosphodiesterase (CNPase) (Chemicon); and anti-βIII-tubulin (Promega). Rabbit and murine isotype-matched negative control antibodies were also used (Caltag Laboratories, Burlingame, CA).
Transplantation.
Approximately 2.0 × 106 SHED were mixed with 40 mg of hydroxyapatite/tricalcium phosphate (HA/TCP) ceramic powder (Zimmer, Warsaw, IN) and then transplanted s.c. into immunocompromised mice (NIH-bg-nu-xid, Harlan–Sprague–Dawley) as described (19).
SHED were injected into the brain of immunocompromised mice according to specifications of an approved small-animal protocol (National Institute of Dental and Craniofacial Research 01-185). Coordinates for the target sites were determined by referencing a murine brain atlas (ref. 20; see Fig. Fig.55A). The anteroposterior, mediolateral, and dorsoventral coordinates were computed relative to Bregma. Ex vivo-expanded SHED (10,000 cells per ml) were infused to the dentate gyrus of the hippocampus (21, 22). Cells (0.5 ml per side) were infused to the coordinates (anteroposterior, mediolateral, and dorsoventral, respectively, −1.5 mm, ±0.8 mm, and −2.0 mm) by using a 1-ml Hamilton Syringe.

Transplantation of SHED into the brain. (A) Diagram indicating injection of SHED into the dentate gyrus of the hippocampus. (B) SHED were cultured in the neural-differentiation medium as described in Materials and Methods for 1 week, after which 5,000 cells in 0.5 μl of PBS were injected into the dentate gyrus of the hippocampus of immunocompromised mice. After 10 days, the brain was fixed and prepared for immunofluorescence staining with NFM and human-specific anti-mitochondrial antibody. The anti-mitochondrial antibody immunostaining showed human SHED (arrows, green) in the dentate gyrus of the hippocampus with coexpression of NFM (arrows, red). In merged images, coexpression of human mitochondria and NFM showed colocalization of antigen expression as indicated by yellow fluorescence (arrows). (Magnification, ×20.)
RT-PCR.
The PCR primers included peroxisome proliferator-activated receptor-γ2 sense (5′-CTCCTATTGACCCAGAAAGC-3′) (nucleotides 114–133) and antisense (5′-GTAGAGCTGAGTCTTCTCAG-3′) (nucleotides 441–460, GenBank accession no. XM_003059); lipoprotein lipase sense (5′-ATGGAGAGCAAAGCCCTGCTC-3′) (nucleotides 175–195) and antisense (5′-GTTAGGTCCAGCTGGATCGAG-3′) (nucleotides 718–738, GenBank accession no. XM_044682); CBFA1 sense (5′-CAGTTCCCAAGCATTTCATCC-3′) (nucleotides 880–900) and antisense (5′-TCAATATGGTCGCCAAACAG-3′) (nucleotides 1304–1323, GenBank accession no. L40992); Osterix sense (5′-GCAGCTAGAAGGGAGTGGTG-3′) (nucleotides 821–840) and antisense (5′-GCAGGCAGGTGAACTTCTTC-3′) (nucleotides 1160–1179, GenBank accession no. XM_062600); Osteocalcin sense (5′-CATGAGAGCCCTCACA-3′) (nucleotides 18–33) and antisense (5′-AGAGCGACACCCTAGAC-3′) (nucleotides 316–332, GenBank accession no. X53698); and GAPDH sense (5′-AGCCGCATCTTCTTTTGCGTC-3′) (nucleotides 12–32) and antisense (5′-TCATATTTGGCAGGTTTTTCT-3′) (nucleotides 807–827, GenBank accession no. M33197). Total RNA isolation, first-strand cDNA synthesis, and PCR processes were performed as described (23).
In Situ Hybridization.
Human-specific alu and murine-specific pf1 sequences labeled with digoxigenin were used as probes for in situ hybridization as described (7). Primers included human alu sense (5′-TGGCTCACGCCTGTAATCC-3′) (nucleotides 90–108) and antisense (5′-TTTTTTGAGACGGAGTCTCGC-3′) (nucleotides 344–364, GenBank accession no. AC004024) and murine pf1 (sense, 5′-CCGGGCAGTGGTGGCGCATGCCTTTAAATCCC-3′) (nucleotides 170–201) and antisense (5′-GTTTGGTTTTTGAGCAGGGTTCTCTGTGTAGC-3′) (nucleotides 275–306, GenBank accession no. X78319).
Immunohistochemistry.
SHED were subcultured into eight-chamber slides (2 × 104 cells per well) (Nunc). The cells were fixed in 4% formaldehyde for 15 min and then blocked and incubated with primary antibodies (1:200–1:500 dilution) for 1 h, respectively. The samples were subsequently incubated with goat secondary antibodies of either IgG-rhodamine red or IgG-Cy2 (Jackson ImmunoResearch) for 45 min. For enzymatic immunohistochemical staining, the Zymed broad-spectrum immunoperoxidase AEC kit was used according to manufacturer protocol.
Western Blot.
Primary antibodies were the same as those used in immunohistochemical staining at dilutions ranging from 1:200 to 1:1,000. Western blot was performed as reported (24).
Fluorescence-Activated Cell Sorting.
SHED were collected from culture and incubated with STRO-1 (IgM) antibodies or isotype-matched negative control antibodies for 1 h on ice. Fluorescence-activated cell-sorting analysis was the same as described (23).
Results
Here we demonstrate that the remaining crown of exfoliated deciduous teeth contains a living pulp remnant comprised of a normal dental pulp including connective tissue, blood vessels, and odontoblasts (Fig. (Fig.11 A–C). To isolate stem cells, single-cell suspensions were derived from the remnant pulp and placed at low density in liquid culture. Approximately 12–20 cells from each exfoliated incisor were capable of forming adherent colonies (Fig. (Fig.11D), characteristic of other stromal stem-cell populations (7). Interestingly, when cultured either under a neuronal-differentiation condition or in 3% rat serum with B27 supplement, these cells formed sphere-like clusters (Fig. (Fig.11E) in which highly proliferative cells aggregated together in clusters that either adhered to the culture dish or floated freely in the culture medium. After separating the sphere-like clusters, the cells were able to grow as individual fibroblastic cells (Fig. (Fig.11F). When compared with adult bone marrow stromal stem cells (BMSSCs) and dental pulp stem cells (DPSCs), SHED showed a higher proliferation rate (Fig. (Fig.11G) and a higher number of population doublings (Fig. (Fig.11H).
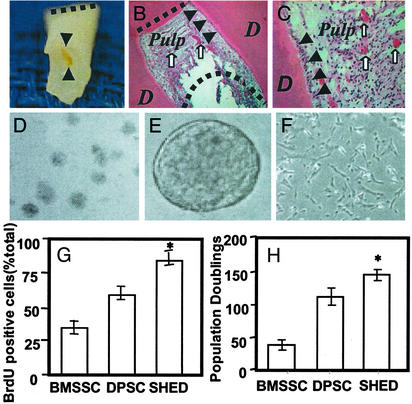
Isolation of SHED. (A) The exfoliated primary incisor contained dental pulp as shown (black triangles). The dashed line shows the occlusion edge of the incisor. (B and C) Hematoxylin/eosin staining indicated dentin (D) and pulp of exfoliated deciduous teeth. The pulp contained odontoblasts (arrows), blood vessels (open arrows), and connective tissues. The straight and curved dashed lines in B represent the occlusion and resorbed root surfaces, respectively. (D) Single colonies were formed after SHED were plated at low density and cultured for 2 weeks. (E) SHED were capable of forming sphere-like clusters when cultured with the conditions described in Materials and Methods. (F) The sphere-like clusters could be dissociated by passage through needles and subsequently grew on 0.1% gelatin-coated dishes. (G) The proliferation rates of SHED, BMSSCs, and DPSCs were assessed by BrdUrd (BrdU) incorporation for 12 h. SHED showed a significantly higher proliferation rate in comparison with BMSSCs and DPSCs (*, P < 0.05, Student's t test). (H) SHED were able to proliferate to >140 population doublings, which was significantly higher (*, P < 0.05, Student's t test) than BMSSCs and DPSCs.
Ex vivo-expanded SHED were found to express the cell-surface molecules STRO-1 and CD146 (MUC18), two early mesenchymal stem-cell markers previously found to be present in BMSSCs and DPSCs (Fig. (Fig.22 D and E). STRO-1- and CD146-positive cells were found to be located around blood vessels of the remnant pulp by immunohistochemical staining (Fig. (Fig.22 A and B), implying that SHED may have originated from a perivascular microenvironment. A minor proportion (9%) of ex vivo-expanded SHED stained positive for the STRO-1 antibody with fluorescence-activated cell-sorting analysis (Fig. (Fig.22C). Further immunohistotypic analysis demonstrated that cultured SHED expressed stromal- and vascular-related markers ALP, MEPE, bFGF, and endostatin (Fig. (Fig.22 F–I).
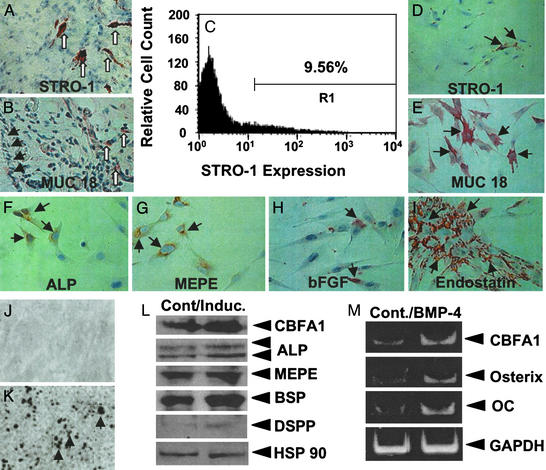
SHED possessed stem-cell characteristics. (A–E) The remnant pulp showed STRO-1 (open arrows in A) and CD146 (open arrows in B) immunopositive staining for cells in perivascular areas. Fluorescence-activated cell-sorting analysis showed that ex vivo-expanded SHED contained ≈9% STRO-1-positive cells (C). SHED expressed STRO-1 (D) and CD146 (E) (arrows). (F–I) SHED expressed the osteogenic and angiogenic markers ALP, MEPE, bFGF, and endostatin. (J and K) SHED were either cultured with regular medium (J) or l-ascorbate-2-phosphate, dexamethasone, and inorganic phosphate for 4 weeks (K). Alizarin red staining showed mineralized nodule formation in the induction (K). (L) Western blot analysis showed an up-regulated expression of CBFA1, ALP, MEPE, bone sialoprotein (BSP), and DSPP after the induction as described above. HSP90 was used to assess the amount of protein loaded per sample. (M) Human recombinant BMP-4 (300 ng/ml, 24 h) was added to induce a significant up-regulation of CBFA1, Osterix, and Osteocalcin (OC) in SHED as detected by semiquantitative PCR.
To investigate the potential of SHED to differentiate into mineralized tissue, established secondary SHED cultures were supplemented with l-ascorbate-2-phosphate, dexamethasone, and inorganic phosphate as described (7). Alizarin red-positive nodules formed in the SHED cultures after 4 weeks of induction (Fig. (Fig.22 J and K), indicating calcium accumulation in vitro. Accordingly, Western blot analysis revealed that various bone markers CBFA1, ALP, MEPE, and bone sialoprotein were up-regulated under the induction (Fig. (Fig.22L). In addition, DSPP was induced by the mineralizing induction (Fig. (Fig.22L). Furthermore, BMP-4 treatment was capable of inducing an up-regulated expression of CBFA1, Osterix, and Osteocalcin by semiquantitative RT-PCR (Fig. (Fig.22M). These data indicated that SHED possessed the ability to differentiate into functional odontoblast-like cells in vitro.
To validate the capacity of SHED to form odontoblasts, ex vivo-expanded SHED were transplanted into immunocompromised mice (7, 23). The transplants yielded human-specific alu-positive odontoblasts directly associated with a dentin-like structure (Fig. (Fig.33 A and B). Importantly, the regenerated dentin was immunoreactive to dentin-specific DSPP antibody (Fig. (Fig.33C). These findings indicated that human SHED satisfies one important stem-cell attribute: the ability to differentiate into odontoblasts in vivo. However, SHED were unable to regenerate a complete dentin–pulp-like complex as do DPSCs in vivo (Fig. (Fig.33 A and E). In addition, SHED were capable of inducing recipient murine cells to differentiate into bone-forming cells as noted by murine-specific pf1 in situ hybridization (Fig. (Fig.33L) and lacked DSPP expression (Fig. (Fig.33D). Importantly, skin fibroblasts were never capable of inducing bone formation after in vivo transplantation (data not shown). These data indicated that SHED are distinctively different from DPSCs in respect to the odontogenic differentiation and osteogenic induction.
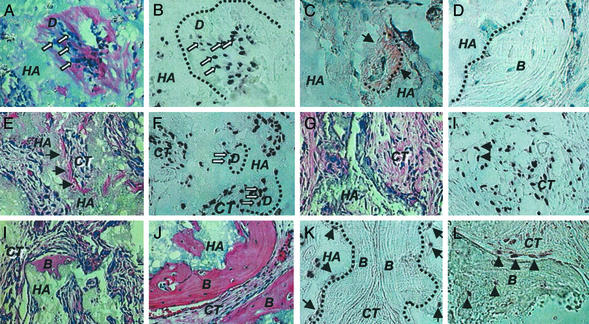
Transplanted SHED into immunocompromised mice. (A and B) After 8 weeks of transplantation, SHED were able to differentiate into odontoblasts (open arrows) that were responsible for the dentin-like structure (D) formation on the surfaces of HA (A). The same field is shown for human-specific alu in situ hybridization, indicating the human origin of odontoblasts (open arrows, B). The black dashed line represents interface between newly formed dentin (D) and HA/TCP (HA). (C) Immunohistochemical staining of anti-DSPP antibody shows a positive staining on the regenerated dentin (black arrows). (D) In contrast to DPSC transplants, newly generated bone (B) by host cells in the same SHED transplant shows no reactivity to the DSPP antibody. (E) Of 12 selected single-colony-derived SHED strains, only 3 (25%) were capable of generating dentin in vivo. Newly formed dentin (arrows) was found to be adjacent to the surfaces of HA/TCP carrier (HA) and associated with connective tissue (CT). (F) Human-specific alu in situ hybridization showed that human cells (open arrows) were associated with dentin formation (D) and were residing within the connective-tissue compartment (CT). (G) The remaining 75% (9 of 12) single-colony-derived SHED strains were unable to generate dentin in vivo. (H) In situ hybridization demonstrated that alu-positive human cells survived in the connective-tissue compartment (CT) in the transplants in which there was no odontogenesis. Human cells were also found to surround the blood vessels (arrows). (I) Seven of 12 (58.4%) single-colony-derived SHED lines induced a very limited amount of bone formation (B) on the surface of HA/TCP (HA). (J) Of 12 single-colony-derived SHED lines, 5 (41.6%) were able to induce a significant amount of bone formation (B) on the surfaces of HA/TCP (HA). (K) The alu in situ hybridization showed human cells (arrows) attached to the surfaces of HA/TCP (HA) at the initial site of bone formation (B). The black dashed lines represent the interface between newly formed bone (B) and HA/TCP (HA). (L) In situ hybridization studies showed the murine-specific pf1 DNA probe reacting with osteoblasts and osteocytes (arrows) associated with the new bone formation (B).
We next examined the characteristics of clonal cell strains, each originating from a single cell of deciduous pulp. When 12 single-colony-derived SHED clones were transplanted into immunocompromised mice, only one fourth (3 of 12) of the clones demonstrated a potential to generate ectopic dentin-like tissue on the HA/TCP carrier equivalent to that generated by multicolony-derived SHED (Fig. (Fig.33 E and G). SHED from either single or multiple colonies were found to form dentin-like tissue (Fig. (Fig.33F) and to survive in the fibrous tissue within the transplants (Fig. (Fig.33H) as demonstrated by human-specific alu in situ hybridization. These results infer that SHED may contain subpopulations of cells, either differentiating into odontoblasts or residing in the connective-tissue compartments. Surprisingly, all transplanted single-colony-derived SHED clones were capable of inducing bone formation in immunocompromised mice. Approximately 40% of the clonal cell strains (5 of 12) induced a significant amount of new bone, whereas the remaining 60% (7 of 12) induced a limited amount of bone (Fig. (Fig.33 I and J). SHED were found to be located on the surfaces of HA/TCP but did not participate in bone formation as indicated by human-specific alu in situ hybridization (Fig. (Fig.33K). In contrast, murine host cells were found to differentiate into osteoblasts and osteocytes as shown by reactivity to murine-specific pf1 in situ hybridization (Fig. (Fig.33L).
Next we studied the potential of SHED to develop into other cell lineages such as neural cells and adipocytes. To elucidate the neural-differentiation potential of SHED, we examined the expression of neural markers in SHED. We found that cultured SHED expressed a variety of neural cell markers including nestin, βIII-tubulin, GAD, NeuN, GFAP, NFM, and CNPase as measured by immunocytochemical staining (Fig. (Fig.44 A–H) and Western blot analysis (Fig. (Fig.44I). After 4 weeks of neural inductive culture, expression levels of neuronal markers including βIII-tubulin, GAD, and NeuN were increased, whereas the levels of nestin, GFAP, NFM, and CNPase remained unchanged (Fig. (Fig.44I). When cultured under these conditions, SHED lost their fibroblastic morphology and developed multicytoplasmic processes correlating with either βIII-tubulin/GAD or βIII-tubulin/NFM expression (Fig. (Fig.44 J–O). The long cellular processes could be viewed best after toluidine blue staining and were immunoreactive to MAP2 and Tau antibodies (Fig. (Fig.44 P–S). After the neural inductive culture, SHED continued to express glial cell makers such as nestin, CNPase, GFAP, and NFM (Fig. (Fig.44 T–W).
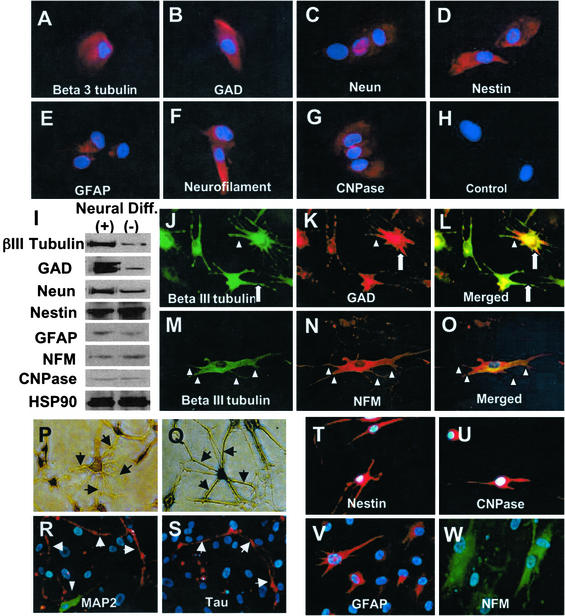
Neural differentiation of SHED. (A–H) Immunocytochemical staining depicts SHED expressing nestin, GFAP, NFM, CNPase, βIII-tubulin, GAD, and NeuN. (I) Western blot analysis confirmed that SHED expressed neural markers as described above. After 4 weeks of culture in the presence of B27 supplement, bFGF (40 ng/ml), and epidermal growth factor (20 ng/ml) (Neural Diff. +), expression levels of βIII-tubulin, GAD, and NeuN were up-regulated when compared with regular culture conditions as described in Materials and Methods (Neural Diff. −). However, expression levels of nestin, GFAP, CNPase, and NFM remained the same after the treatment. (J–O) SHED may coexpress neuronal markers including βIII-tubulin (green)/GAD (red) and βIII-tubulin (green)/NFM (red). The morphology of SHED showed elongated cell-cytoplasmic processes that sometimes coexpress neural markers (triangles) or only express individual neural marker (open arrows). (P–S) Toluidine blue (0.1%) staining depicting the altered morphology of SHED after induction with neural culture medium (P and Q, arrows). Immunopositive staining for anti-MAP2 and anti-Tau antibodies on dendrites and axon (R and S, arrows), respectively. Double-staining experiments showing βIII-tubulin-positive cells were also detected in the same field (R, triangle, green). (T–W) SHED continued to express glial cell markers including nestin (red), CNPase (red), GFAP (red), and NFM (green) by immunocytostaining.
Neural developmental potential was studied further in vivo by injecting SHED into the dentate gyrus of the hippocampus of immunocompromised mice (Fig. (Fig.55A). Histological examination showed that SHED survived for >10 days inside the mouse brain microenvironment as noted by human-specific antimitochondrial antibody staining and continued to express neural markers such as NFM (Fig. (Fig.55B).
After 5 weeks of culture with an adipogenic inductive mixture, ≈5% of cultured SHED were found to possess the potential to develop into Oil red O-positive lipid-laden fat cells (Fig. (Fig.66A). This correlated with an up-regulation in the expression of two adipocyte-specific transcripts, peroxisome proliferator-activated receptor-γ2 and lipoprotein lipase, as detected by semiquantitative RT-PCR (Fig. (Fig.66B).
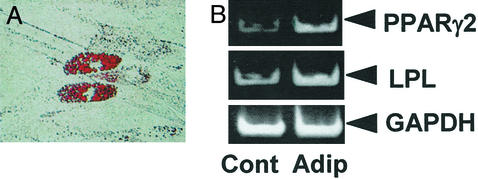
Adipogenic differentiation of SHED. (A) Cultured SHED formed Oil red O-positive lipid clusters after 5 weeks of induction in the presence of 0.5 mM isobutylmethylxanthine, 0.5 μM hydrocortisone, and 60 μM indomethacin. (B) A significant up-regulation of peroxisome proliferator-activated receptor-γ2 (PPARγ2) and lipoprotein lipase (LPL) was observed in the group induced with the adipogenic mixture (Adip) as compared with the control group (Cont) by RT-PCR.
Discussion
Here we provide evidence that remnant dental pulp derived from exfoliated deciduous teeth contains a multipotent stem-cell population. These stem cells can be isolated and expanded ex vivo, thereby providing a unique and accessible population of stem cells from an unexpected tissue resource. Previous experiments have shown that dental pulp tissue of adult teeth contains a population of DPSCs that are capable of differentiating into odontoblasts and adipocytes as well as expressing nestin and GFAP and form a dentin/pulp-like complex after in vivo transplantation (23). Deciduous teeth are significantly different from permanent teeth with regards to their developmental processes, tissue structure, and function. Therefore, it is not a surprise to find that SHED are distinct from DPSCs with respect to their higher proliferation rate, increased cell-population doublings, sphere-like cell-cluster formation, osteoinductive capacity in vivo, and failure to reconstitute a dentin–pulp-like complex. SHED apparently represent a population of multipotent stem cells that are perhaps more immature than previously examined postnatal stromal stem-cell populations.
The mechanisms controlling the growth and replacement of teeth are largely unknown (17), in particular with respect to how craniofacial components including bone and soft tissues surrounding teeth participate in the process of tooth development. SHED demonstrated a strong capacity to induce recipient cell-mediated bone formation in vivo. According to our investigations, SHED could not differentiate directly into osteoblasts but did induce new bone formation by forming an osteoinductive template to recruit murine host osteogenic cells. These data imply that deciduous teeth may not only provide guidance for the eruption of permanent teeth, as generally assumed, but may also be involved in inducing bone formation during the eruption of permanent teeth.
It is notable that SHED expressed neuronal and glial cell markers, which may be related to the neural crest-cell origin of the dental pulp (25). Neural crest cells play a pivotal role in embryonic development, giving rise to a variety of cell types such as neural cells, pigment cells, smooth muscle, craniofacial cartilage, and bone (26). Previous studies demonstrated that BMSSCs were also capable of differentiating into neural-like cells after in vivo transplantation (27). Dental pulp cells are known to produce neurotrophic factors and even rescue motoneurons after spinal cord injury (28). Moreover, neural progenitors were identified recently in mammalian dermal skin layers (6). These evidences support the notion that stem cells of nonneural tissue may be capable of differentiating into neural cells.
Our study provides evidence that SHED represent a population of postnatal stem cells capable of extensive proliferation and multipotential differentiation. Deciduous teeth therefore may be an ideal resource of stem cells to repair damaged tooth structures, induce bone regeneration, and possibly to treat neural tissue injury or degenerative diseases. However, the biological significance of the existence of SHED remains to be determined. This study provides a description of a stem-cell population residing in exfoliated human deciduous teeth and establishes the foundation for further studies to determine the efficacy of using SHED in cellular-based therapies.
Acknowledgments
This work was supported by the Division of Intramural Research, National Institute of Dental and Craniofacial Research of the National Institutes of Health, and Department of Health and Human Service.
Abbreviations
SHED | stem cells from human exfoliated deciduous teeth |
FGF | fibroblast growth factor |
bFGF | basic FGF |
CBFA1 | core-binding factor, runt domain, α subunit 1 |
GAD | glutamic acid decarboxylase |
MEPE | matrix extracellular phosphoglycoprotein |
DSPP | dentin sialophosphoprotein |
GFAP | glial fibrillary acidic protein |
NFM | neurofilament M |
NeuN | neuronal nuclei |
CNPase | 2′,3′-cyclic nucleotide-3′-phosphodiesterase |
HA/TCP | hydroxyapatite/tricalcium phosphate |
BMSSC | bone marrow stromal stem cell |
DPSC | dental pulp stem cell |
ALP | alkaline phosphatase |
References
Articles from Proceedings of the National Academy of Sciences of the United States of America are provided here courtesy of National Academy of Sciences
Full text links
Read article at publisher's site: https://doi.org/10.1073/pnas.0937635100
Read article for free, from open access legal sources, via Unpaywall:
http://www.pnas.org/content/100/10/5807.full.pdf
Citations & impact
Impact metrics
Citations of article over time
Alternative metrics

Discover the attention surrounding your research
https://www.altmetric.com/details/101865842
Article citations
Dental stem cell dynamics in periodontal ligament regeneration: from mechanism to application.
Stem Cell Res Ther, 15(1):389, 31 Oct 2024
Cited by: 0 articles | PMID: 39482701 | PMCID: PMC11526537
Review Free full text in Europe PMC
The Differentiation Potential of Apical Papilla Cells in Relation to Tenascin-C and Syndecan-1 Expression and Their Potential Role in Regeneration.
Int J Dent, 2024:7295498, 20 Sep 2024
Cited by: 0 articles | PMID: 39345930 | PMCID: PMC11436271
Distinct muscle regenerative capacity of human induced pluripotent stem cell-derived mesenchymal stromal cells in Ullrich congenital muscular dystrophy model mice.
Stem Cell Res Ther, 15(1):340, 07 Oct 2024
Cited by: 0 articles | PMID: 39370505 | PMCID: PMC11457425
Specific cell subclusters of dental pulp stem cells respond to distinct pathogens through the ROS pathway.
Front Cell Infect Microbiol, 14:1452124, 12 Sep 2024
Cited by: 0 articles | PMID: 39328360 | PMCID: PMC11424553
Mesenchymal Stem Cell Therapy: Therapeutic Opportunities and Challenges for Diabetic Kidney Disease.
Int J Mol Sci, 25(19):10540, 30 Sep 2024
Cited by: 0 articles | PMID: 39408867 | PMCID: PMC11477055
Review Free full text in Europe PMC
Go to all (1,476) article citations
Other citations
Data
Data behind the article
This data has been text mined from the article, or deposited into data resources.
BioStudies: supplemental material and supporting data
Nucleotide Sequences (5)
- (1 citation) ENA - X53698
- (1 citation) ENA - AC004024
- (1 citation) ENA - L40992
- (1 citation) ENA - M33197
- (1 citation) ENA - X78319
Similar Articles
To arrive at the top five similar articles we use a word-weighted algorithm to compare words from the Title and Abstract of each citation.
[Isolation and identification of stem cells derived from human exfoliated deciduous teeth].
Nan Fang Yi Ke Da Xue Xue Bao, 29(3):479-482, 01 Mar 2009
Cited by: 0 articles | PMID: 19304530
Stem Cells from Human Exfoliated Deciduous Teeth: A Growing Literature.
Cells Tissues Organs, 202(5-6):269-280, 20 Aug 2016
Cited by: 35 articles | PMID: 27544531
Review
Induced in vitro differentiation of neural-like cells from human exfoliated deciduous teeth-derived stem cells.
Int J Dev Biol, 55(2):189-195, 01 Jan 2011
Cited by: 57 articles | PMID: 21671222
Comparison of mesenchymal-like stem/progenitor cells derived from supernumerary teeth with stem cells from human exfoliated deciduous teeth.
Regen Med, 6(6):689-699, 01 Nov 2011
Cited by: 17 articles | PMID: 22050521