Abstract
Free full text

A Dynamic Relationship between Intracellular and Extracellular Pools of Aβ
Abstract
The accumulation of the amyloid-β peptide (Aβ) in the brain is considered to have a primary role in Alzheimer’s disease (AD). In addition to the extracellular accumulation of Aβ in the parenchyma and cerebrovasculature, emerging evidence indicates that intraneuronal Aβ also plays a pathophysiological role in AD. It is unclear, however, if the intracellular and extracellular pools of Aβ are unrelated or connected. In these studies, we sought to establish a relationship between these two pools of Aβ. We identified an inverse relationship between intracellular and extracellular Aβ in the 3xTg-AD transgenic model of AD. Using an immunotherapy approach, we further found that extracellular Aβ was cleared before intracellular Aβ. After the antibody dissipated, however, the reappearance of extracellular plaques was preceded by the accumulation of intraneuronal Aβ. Taken together, these results provide strong experimental evidence that intraneuronal Aβ may serve as a source for some of the extracellular amyloid deposits.
Alzheimer’s disease (AD) is the most common cause of dementia. Neuropathologically, it is marked by two hallmark features: the accumulation of diffuse and neuritic plaques, mainly comprised of the amyloid-β peptide (Aβ), and neurofibrillary tangles, which consist of hyperphosphorylated tau protein.1 Besides plaques and tangles, the AD brain is characterized by profound cell loss, particularly impacting cholinergic neurons. Although the genesis of plaques is not well understood, the accumulation of Aβ is considered the earliest event in the cascade leading to AD neurodegeneration.2 Aβ is generated via the endoproteolytic cleavage of the amyloid precursor protein (APP) by the sequential activity of different secretases, presumably leading to the extracellular secretion of Aβ. It has also been established that Aβ may be generated in intracellular compartments such as the endoplasmic reticulum and the trans-Golgi.3–8
Emerging evidence suggests that intraneuronal Aβ plays a pathophysiological role in the progression of the disease.9 In this regard, several studies indicate that memory impairments in various transgenic models of AD precede the accumulation of extracellular plaques.10–12 We previously generated a transgenic model of AD (3xTg-AD) that develops an age-dependent accumulation of both plaques and tangles in disease-relevant brain regions.13 In these mice, the first pathological manifestation is the accumulation of intraneuronal Aβ, and deficits in synaptic plasticity, learning, and memory appear to be induced by the buildup of intraneuronal Aβ.12–14 Furthermore, we recently found that the 3xTg-AD mice show a loss of α7 nicotinic acetylcholine receptors restricted to brain regions that accumulate intraneuronal Aβ.15 The sum of these studies in transgenic mice clearly supports a pathophysiological role for intraneuronal Aβ. More significantly, there is also evidence from human studies supporting a role for intracellular Aβ.9. For example, in the Down’s syndrome brain, it has been shown that intraneuronal Aβ pool progressively decreases in an age-related manner as the extracellular plaque load increases.16 It is unclear, however, whether the intraneuronal and extracellular pools of Aβ are mechanistically linked or independent of each other.
We previously showed that a single intrahippocampal injection of an anti-Aβ antibody successfully removes not only extracellular Aβ deposits but also intraneuronal Aβ pathology.17 In this current study, we again used an immunotherapy approach to investigate the relationship between the intracellular and extracellular Aβ pools. We show that the extracellular pool is reduced first, within 12 hours of a single intrahippocampal injection of an anti-Aβ antibody, followed by the reduction of the intracellular pool by day 3 after injection. Notably, after the antibody dissipates, we found that the intraneuronal Aβ pathology re-emerges before the appearance of extracellular plaques. We further show that in the brains of the 3xTg-AD mice, the intraneuronal Aβ immunoreactivity decreases as the extracellular plaque load increases. These studies provide compelling experimental evidence that these two pools of Aβ are related, and that the accumulation of extracellular Aβ may be at least partially dependent on the accumulation of intraneuronal Aβ.
Materials and Methods
Mice
The generation of the 3xTg-AD mice has been described elsewhere.13 Briefly, 3xTg-AD mice were derived by co-microinjecting two independent transgenes encoding human APPSwe and the human tauP301L (both under control of the mouse Thy1.2 regulatory element) into single-cell embryos harvested from homozygous mutant PS1M146V knockin (PS1-KI) mice.13 Tissue from Tg2576 mice18 was obtained from the Institute for Brain Aging and Dementia at the University of California, Irvine.
Surgeries
Homozygous 3xTg-AD and non-Tg mice (12 to 14 months old) were anesthetized with 0.6 ml of avertin per 25 g of body weight (1.3% tribromoethanol, 0.8% amylalcohol) and placed in a stereotactic apparatus (MyNeuroLab, St. Louis, MO) with a mouse adaptor. The monoclonal anti-Aβ antibody 1560 (2 μg; Chemicon, Temecula, CA) was injected into the left hippocampus through a 33-gauge injector attached to a 5-μl Hamilton syringe (Hamilton Co., Reno, NV). The coordinates, with respect to bregma, were −2.7 mm posterior, +2.5 mm lateral, and −3.0 ventral to the skull. The rate of injection was 1 μl/minute, after which the cannula was left in place for an additional 5 minutes to allow for diffusion. Animals were kept on a warming pad until they had fully recovered from anesthesia, and were kept in individual cages to prevent damage to the scalp sutures until they were sacrificed for tissue processing. All animal procedures were performed in accordance with the National Institutes of Health Guide for the Care and Use of Laboratory Animals and approved by the University of California, Irvine, Institutional Animal Care and Use Committees. All appropriate measures were taken to minimize pain and discomfort in experimental animals.
Immunohistochemistry
Mice were sacrificed by CO2 asphyxiation and the brains were fixed for 48 hours in 4% paraformaldehyde. Free-floating sections (50 μm thick) were obtained using a vibratome slicing system (Pelco, Redding, CA) and stored in phosphate-buffered saline. The endogenous peroxidase activity was quenched for 30 minutes in 3% H2O2. Sections were then incubated in 90% formic acid (FA) for 7 minutes to expose the epitope. The appropriate primary antibody was applied overnight at 4°C. Sections were washed with Tris-buffered saline (TBS) and incubated with the appropriate secondary antibody for 1 hour at 20°C. Sections were developed with diaminobenzidine substrate using the avidin-biotin-horseradish peroxidase system (Vector Laboratories, Burlingame, CA).
Antibodies
The following antibodies were used in this study: anti-Aβ 6E10 (Signet Laboratories), anti-Aβ 1560 (Chemicon), anti-Aβ40 and anti Aβ42 (Biosource, Camarillo, CA), anti-Aβ 35-40 (MM32-13.1.1, for detecting Aβ40) anti-Aβ 35-42 (MM40-21.3.4, for detecting Aβ42), anti-IDE1 (a generous gift from Dr. D.J. Selkoe, Brigham and Women’s Hospital, Harvard University), anti-APP clones 22C11 and CT20 (Calbiochem, La Jolla, CA), anti-NEP (Abcam), and anti-β-actin (Sigma, St. Louis, MO).
Protein Extraction and Western Blot
Mice were sacrificed by CO2 asphyxiation. Brains were homogenized in 50 mmol/L Tris, pH 8.0, containing 0.7 mg/ml pepstatin A supplemented with a complete miniprotease inhibitor tablet (Roche, Basel, Switzerland). The homogenized mixes were briefly sonicated to sheer the DNA and centrifuged at 4°C for 1 hour at 100,000 × g. The supernatant containing the cytoplasmic fraction was stored at −80°C until used. The pellet was rehomogenized in a solution of 10 mmol/L Tris, pH 7.5, 150 mmol/L NaCl (TBS), and 2% Triton containing a complete mini protease inhibitor tablet and centrifuged at 4°C for 1 hour at 100,000 × g. The supernatant, now containing the membrane fraction, was stored at −80°C until used.
Proteins were resolved by sodium dodecyl sulfate/polyacrylamide gel electrophoresis (10% Bis-Tris from Invitrogen, Carlsbad, CA) under reducing conditions and transferred to a nitrocellulose membrane. The membrane was incubated in a 5% solution of nonfat dry milk for 1 hour at 20°C. After overnight incubation at 4°C with primary antibody, the blots were washed in Tween 20-TBS (T-TBS) (0.02% Tween 20, 100 mmol/L Tris, pH 7.5, 150 nmol/L NaCl) for 20 minutes and incubated at 20°C with the appropriate secondary antibody for 1 hour. The blots were washed in T-TBS for 20 minutes, incubated for 5 minutes with Super Signal (Pierce, Rockford, IL), washed, and exposed.
Aβ Quantification and Statistical Analysis
To quantify the age-related changes in intraneuronal Aβ immunoreactivity and number of plaques, photomicrographs (six mice/group, three sections/mouse, three photographs/section) were taken with a Zeiss digital camera and imported into the Scion Image system (NIH, Bethesda, MD) and converted to black and white photos. Threshold intensity was manually set and kept constant, and the number of pixels was determined for Aβ-immunostained sections. To keep the area analyzed constant from mouse to mouse, we imaged only sections of the hippocampus underneath the rhinal fissure. A similar analysis was performed for Nissl staining. To determine the number of plaques, three images per brain section per mouse were taken using a ×20 objective and the number of plaques was manually counted. Quantification for the immunization experiments was done as following: three pictures/section were taken in the area surrounding the injection site and the number of pixels and/or number of plaques was compared to similar regions from the uninjected contralateral hippocampus. The data were subsequently analyzed by analysis of variance or t-test comparison using Graphpad Prism software.
Cortical Cultures and Enzyme-Linked Immunosorbent Assay (ELISA) Measurements
Primary cortical neurons were prepared from embryonic day 15 Tg2576 mice as previously described.19 Briefly, cortical neurons were plated at a concentration of 5 × 105 cells per ml. After 12 days in vitro, neurons were incubated in either vehicle (dimethyl sulfoxide) or 10 nmol/L γ-secretase inhibitor XVII (Calbiochem, San Diego, CA) for 0, 1, 3, 12, 24, and 48 hours. Intracellular amyloid was determined from cell lysates obtained using M-Per tissue protein extraction reagent (Perbio Science, Cramlington, UK) containing Complete Mini Protease Inhibitors (Roche Diagnostics, Pleasanton, CA). Secreted amyloid was determined from collected media. Sandwich ELISA was performed as previously described.15
Results
The first neuropathological manifestation in the brains of the 3xTg-AD mice is the buildup of intraneuronal Aβ within certain brain regions.13 To further corroborate the presence of intraneuronal Aβ in the 3xTg-AD mice, we stained serial sections from 9-month-old mice with different antibodies raised against different regions of the APP (Figure 1A). The strongest intraneuronal staining was obtained in sections stained with antibody 1560 (Figure 1B), which is raised against the first 17 amino acids of the Aβ sequence. Although this antibody recognizes any Aβ-containing fragment of APP, when serial sections were stained with 22C11, CT20, APP N-terminal and C-terminal antibodies, intraneuronal staining was markedly reduced compared to the 1560 staining (Figure 1, B–D). These data suggest that the majority of the intraneuronal immunoreactivity detected by 1560 is Aβ. In fact, serial sections stained with two different Aβ42-specific antibodies (see Materials and Methods) further confirm that Aβ42 is the primary species that accumulates in neurons of the 3xTg-AD mice (Figure 1E). Because of the presence of the M146V mutation in the PS1 gene, the 3xTg-AD mice accumulate mainly Aβ42.13
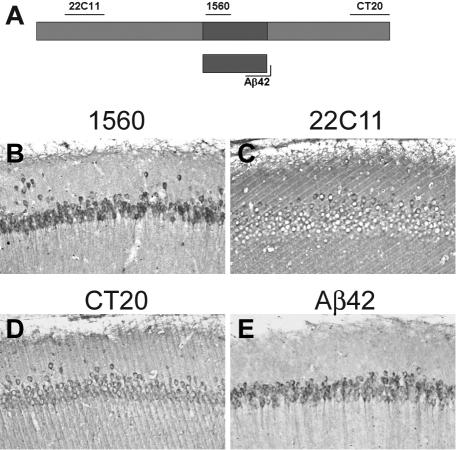
Intraneuronal Aβ accumulates in the brains of 3xTg-AD mice. A: Schematic representation of the epitopes on the APP protein recognized by different antibodies. The diagram is not to scale. B–E: Serial sections were stained with different antibodies. Although some of the intraneuronal material is detectable with 22C11 and CT20, very robust immunostaining is clearly evident with the two different Aβ antibodies.
Although intraneuronal Aβ accumulation is the first overt neuropathological manifestation in the 3xTg-AD mice, it is not yet known whether this pool remains constant with aging, particularly in the context of the age-related buildup of extracellular plaques. Our initial analysis, therefore, centered on determining whether the intraneuronal Aβ pool changes as a function of age. We addressed this matter by analyzing 12- to 18-month-old 3xTg-AD mice because there is a marked increase in the extracellular plaque load during this time period. We focused our study on the hippocampus because this is a well-defined structure that facilitates a more precise quantitative analysis and because it is one of the major brain regions impacted in both human AD and 3xTg-AD mice. Through quantitative imaging analysis, using anti-Aβ42-specific antibodies, we measured the intensity of the Aβ immunostaining in the hippocampus and found a significant reduction in intracellular Aβ immunoreactivity between 12 and 18 months (Figure 2, A–E). In contrast, the number of extracellular plaques markedly increased throughout this time period (Figure 2, A–D and F).
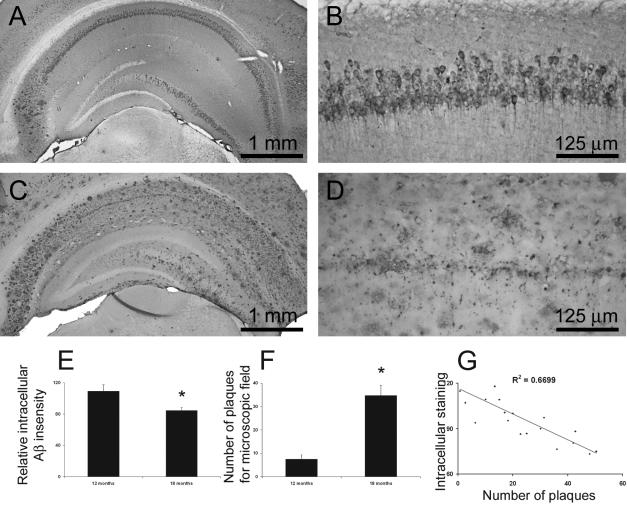
Intraneuronal Aβ immunoreactivity decreases as plaque number increases. A–G: Using Scion image software (from NIH), we measured intraneuronal Aβ immunoreactivity and extracellular Aβ load in the hippocampus of 12-to 18-month-old 3xTg-AD homozygous mice (n = 6 per group, three sections per mouse, three pictures per sections). Representative low- and high-magnification microphotographs of the hippocampus of 12-month-old (A, B) and 18-month-old (C, D) 3xTg-AD mice immunostained with an anti-Aβ42-specific antibody. E: Quantitative analysis of intraneuronal Aβ immunoreactivity shows a significant decrease in levels between 12 and 18 months of age (P < 0.05). F: In contrast, plaque numbers significantly increase during this time (P < 0.01). G: A significant inverse relationship exists between intraneuronal Aβ immunoreactivity and number of amyloid plaques in mice of different ages (n = 20).
To better quantify the relationship between the intracellular and extracellular Aβ pools, we measured intracellular Aβ staining and counted the number of amyloid plaques in 3xTg-AD mice of different ages (n = 20 mice). We found a significant inverse correlation between these two Aβ pools (Figure 2G). A similar inverse relationship between intraneuronal and extracellular Aβ has also been documented in the brains of Down’s syndrome patients.16
There are several likely factors that could account for the age-dependent decrease in intraneuronal Aβ immunoreactivity: 1) neurons are dying between 12 and 18 months of age; 2) the steady-state levels of APP/Aβ decrease between 12 and 18 months of age; 3) the expression of intracellular Aβ-degrading enzymes increases between 12 and 18 months of age; 4) the lack of intra-neuronal Aβ immunoreactivity in old mice may be due to the antibody predominantly binding extracellular plaques; and/or 5) the intraneuronal pool of Aβ contributes to the extracellular plaque load. To distinguish between these possibilities, we stained sections from 12- and 18-month-old mice with Nissl and found that the overall number of CA1 neurons is similar between these two ages (Figure 3A). The lack of terminal dUTP nick-end labeling-positive (data not shown) and fluorojade-positive neurons in the CA1 subfield in 12- and 18-month-old mice (Figure 3A) suggests that the diminished intraneuronal Aβ staining is not due to a decrease in cell number.
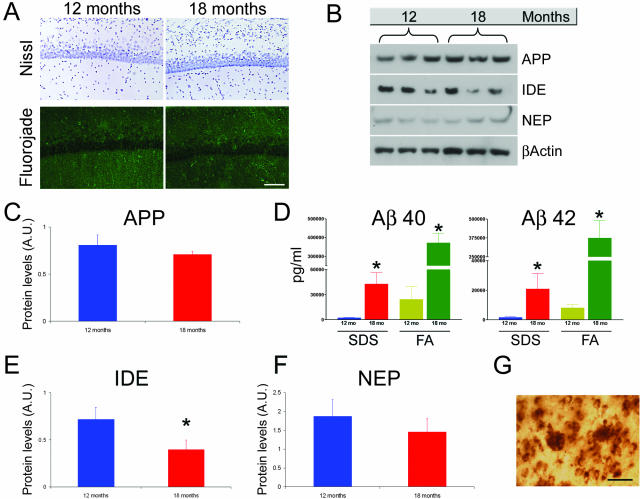
Investigation of experimental parameters that could underlie the age-related decrease in intraneuronal Aβ. A: Representative microphotographs of sections stained with the Nissl and fluorojade technique indicating that there is no evident loss of neurons between 12 and 18 months of age (n = 6 per group). B: Representative immunoblots of protein extracts from the hippocampus of 12- and 18-month-old 3xTg-AD mice analyzed for APP, IDE, and NEP. β-Actin levels were assayed to control for protein loading. Note that the representative blots are n = 3 whereas the quantifications of these blots (see below) are done one a sample of n = 6. C: Quantitative analysis of APP blots shows that full-length APP steady-state levels remain relatively constant during these time points (P = 0.158). D: ELISA measurement of Aβ40 and Aβ42 in 12- and 18-month-old 3xTg-AD mice. There is a significant age-dependent increase in both Aβ40 (P = 0.0054 and P < 0.0001 for sodium dodecyl sulfate and FA, respectively) and Aβ42 levels (P = 0.0476 and P = 0.0038 for sodium dodecyl sulfate and FA, respectively), between these time points. E: Steady-state levels of IDE show a significant age-dependent decrease (P < 0.05). F: A similar decrease was also detected for NEP, although the difference did not achieve statistical significance (P > 0.05). G: Representative microphotograph showing staining of extracellular plaques and nearby neurons that contain intraneuronal Aβ immunoreactivity. Scale bars: 125 μm (A); 50 μm (G).
We next compared the steady-state levels of full-length APP in the hippocampus of 12- and 18-month-old 3xTg-AD mice using quantitative Western blot. We found no statistically significant change in APP levels between these ages (Figure 3, B and C). We also directly measured steady-state levels of Aβ by sandwich ELISA to determine whether the age-dependent decrease in intraneuronal Aβ was due to an overall decrease in total Aβ levels. We found that both Aβ40 and Aβ42 in the soluble and insoluble fractions significantly increased between 12 and 18 months of age (Figure 3D). These results indicate that the reduced intraneuronal Aβ immunoreactivity is not due to a reduction in transgene expression nor to an overall decrease in total Aβ levels.
Aβ accumulation is the result of a balance between production and degradation and different proteases have been shown to degrade Aβ in vivo. Among these, insulin-degrading enzyme (IDE) and neprilysin (NEP) have received the major experimental confirmation.20–22 To determine whether the level of these proteases increases between 12 and 18 months, thereby accounting for the decrease in intraneuronal Aβ staining, we measured IDE and NEP steady-state levels throughout this period in the hippocampus of the 3xTg-AD mice. We found that IDE steady-state levels significantly decreased during this time, and a similar tendency was also apparent for NEP levels, although in this case the difference was not statistically significant (Figure 3, B, E, and F). The decrease in the levels of these Aβ-degrading enzymes between 12 and 18 months in the hippocampus of the 3xTg-AD argues against the possibility that the age-related reduction in intraneuronal Aβ immunoreactivity results from enhanced Aβ clearance. Moreover, these data are consistent with our previous studies showing an age-dependent decrease in IDE and NEP in brain regions susceptible to AD neuropathology.23 The age- and region-dependent decrease in these two Aβ-degrading enzymes may account for the different susceptibility of different brain regions to Aβ pathology.
It is also plausible that the reduced intraneuronal Aβ immunoreactivity in old 3xTg-AD mice is due to the antibody predominantly binding extracellular plaques and not neurons. However, the presence of both plaques and intraneuronal Aβ in the same brain regions argue against this possibility (Figure 3G). In fact, if the antibody were to bind predominantly to plaques and not to neurons, one would expect to find a reduction of intraneuronal Aβ immunoreactivity in neurons next to plaques. Moreover, we also stained sections from the 3xTg-AD mice with an excess of Aβ antibodies and still observed a reduction of intraneuronal Aβ staining between 12 and 18 months of age (data not shown). To determine whether the Aβ immunoreactivity changes as a results of the FA pretreatment that we used to detect extracellular plaques (see Materials and Methods), we stained serial sections from 12-month-old 3xTg-AD mice with and without FA pretreatment and found no difference in the intraneuronal Aβ immunoreactivity (data not shown). Therefore, the age-related decrease in intraneuronal Aβ immunoreactivity is likely not due to limiting concentration of the antibody.
The age-dependent decrease in intraneuronal Aβ immunoreactivity with the concomitant increase in extracellular Aβ plaques has also been demonstrated in Down’s syndrome cases,24 which agrees well with our data from the 3xTg-AD mice. Nevertheless, we sought to determine whether a similar event occurs in another transgenic model of AD. For this study we used the Tg2576 transgenic mice, which overexpress APPswe.18 Considering that these mice mainly accumulate Aβ40, we stained sections from 12- and 18-month-old mice (n = 5) with an Aβ40-specific antibody. We found that intraneuronal Aβ immunoreactivity was present in the CA1 regions of 12-month-old mice (Figure 4A). However, in the same brain region of 18-month-old mice, we found numerous extracellular Aβ plaques but no intraneuronal Aβ immunoreactivity (Figure 4B). Therefore, even in another mouse model of AD such as the Tg2576 mice, intraneuronal Aβ immunoreactivity is observed and declines with age as the extracellular plaque burden increases.
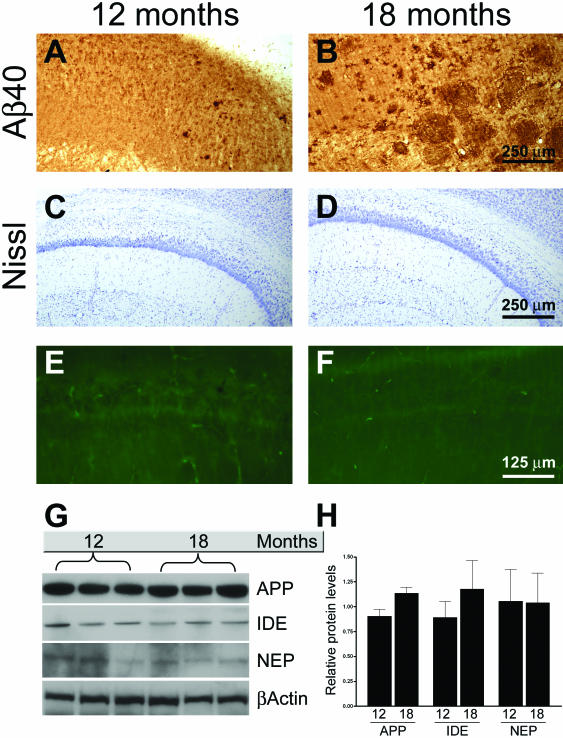
Intraneuronal Aβ immunoreactivity decreases as a function of age in Tg2576 mice. A and B: Sections from 12- and 18-month-old mice (n = 5) were stained with an Aβ40-specific antibody. The photomicrographs reveal intraneuronal Aβ immunoreactivity in 12-month-old mice, which is absent in 18-month-old mice. In contrary, the number of plaques greatly increases during this time. C–F: Representative microphotographs of hippocampal sections stained with the Nissl and fluorojade, respectively, showing that there is no evident loss of neurons between 12 and 18 months of age. G: Representative Western blots showing APP, IDE, and NEP levels as a function of age. H: Quantification of Western blots for APP, IDE, and NEP show no significant changes in the steady-state levels of these proteins between 12- and 18-month old mice. The levels of each time point were adjusted to the relative β-actin levels, which were used as a protein loading control.
To determine whether the age-related decrease in intraneuronal Aβ immunoreactivity was due to a loss of neurons between 12 and 18 months of age, we stained sections from these two time points with Nissl and fluorojade. As for the 3xTg-AD mice, the age-dependent decrease in intraneuronal Aβ immunoreactivity in the Tg2576 brain did not appear to be due to a decrease in cell numbers (Figure 4, E and F). We next addressed whether the levels of APP, IDE, and NEP changed with time in the Tg2576. We found that between 12 and 24 months of age the steady-state levels of APP, IDE, and NEP were unaltered in the hippocampus of Tg2576 mice (Figure 4, G and H).
To directly address whether a dynamic relationship exists between the intraneuronal and extracellular Aβ pools, we also investigated this question with in vitro experiments using primary neurons. The APP transgene in the 3xTg-AD mice is under the control of Thy1.2 promoter, which is expressed at low levels in embryos, rendering Aβ production below threshold for detection.19 Therefore, we used primary neurons from Tg2576 mice, in which the APP transgene is under the prion promoter and is expressed in embryos.18 Neurons were isolated and cultured in vitro for 12 days, after which the media was replaced with conditioned media from nontransgenic mice supplemented with either 10 nmol/L of the potent γ-secretase inhibitor XVII or vehicle only. The potency of this γ-secretase inhibitor has been shown previously.25–27 Although it is unlikely, we cannot exclude that the inhibitor blocks 100% of the production of Aβ. We subsequently measured intracellular and secreted Aβ levels at different time points. We found that the intracellular Aβ40 levels were significantly lower 1 hour after changing the media in both the γ-secretase inhibitor- and vehicle-treated samples (n = 4). In the γ-secretase inhibitor-treated group, as expected, intracellular Aβ40 levels did not change at the other time points, as Aβ production was blocked (Figure 5A). Conversely, in vehicle-treated neurons, after the initial decrease the level of intracellular Aβ significantly increased throughout time (Figure 5A). Although we cannot exclude that this initial decrease in intraneuronal Aβ is due to some Aβ remaining in the secretory pathway at the moment of the application of the inhibitor, the significant decrease in intracellular Aβ levels after 1 hour can be explained by the change in the balance between the intracellular and extracellular Aβ pools that occurred after replacement of the media. Moreover, we found a time-dependent increase in the levels of secreted Aβ40 for both the vehicle- and γ-secretase-treated samples, which is consistent with some of the intraneuronal Aβ pool contributing to the accumulation of secreted Aβ. Notably, the increase is more substantial in the vehicle-treated neurons as Aβ production was not blocked (Figure 5B). Because the production of Aβ is inhibited by the γ-secretase inhibitor, the secreted Aβ must come from the intracellular pool because at time 0 there was no Aβ40 in the conditioned media (Figure 5B). Similar results were obtained for Aβ42 (Figure 5C), although the intraneuronal Aβ42 levels were below detection. Nevertheless, the results are consistent; despite inhibition of Aβ formation, there was a time-dependent increase in secreted Aβ42, paralleling the results obtained with Aβ40.
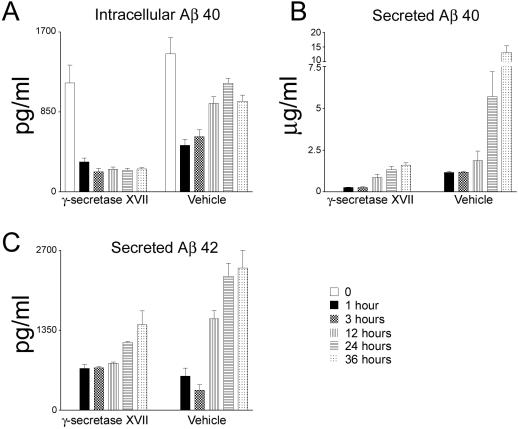
Intracellular Aβ is secreted extracellularly in primary neurons. Primary neurons from Tg2576 transgenic mice were treated with a specific γ-secretase inhibitor or with vehicle only to determine whether there was a dynamic relationship between the intracellular and extracellular Aβ pools. After 12 days in vitro, the media was replaced with conditional media from nontransgenic mice and neurons were treated with either the potent γ-secretase inhibitor XVII or with vehicle only. A: In neurons treated with the γ-secretase inhibitor, the intracellular Aβ40 significantly decreases after changing the media (compare point 0 and 1 hour, P < 0.01) and remains constant after that, whereas in the vehicle-treated neurons, after the initial decrease, the levels of intracellular Aβ40 gradually increase. The significant decrease after media replacement is consistent with the secretion of Aβ. B: Notably, we found that treatment of neurons with the γ-secretase inhibitor result in a significant increase in secreted Aβ40 levels (P < 0.05). Because Aβ production was blocked and that at time 0 there was no detectable Aβ in the media, the secreted Aβ must come from the intracellular pool, where indeed we detect a decrease in Aβ levels. Note that the increase in secreted Aβ40 is higher in the vehicle-treated neurons because Aβ production is not blocked. C: Paralleling the results obtained with Aβ40, we found a time-dependent increase in secreted Aβ42 levels (P < 0.05). The levels of intracellular Aβ42 were below detection.
To determine whether such a relationship between intracellular and extracellular Aβ is present in vivo, we used an immunotherapy approach. We previously showed that both extracellular and intracellular Aβ are cleared within 3 days after a single intrahippocampal injection of anti-Aβ antibodies.17 In this current study, we initially examined which pool was cleared first by administering Aβ antibodies into the left hippocampus of the 3xTg-AD mice and sacrificing the mice at 12, 24, 48, and 72 hours after injection. At 12 hours after antibody administration, there was a marked reduction in the number of extracellular plaques in the ipsilateral versus the uninjected contralateral hippocampus (Figure 6, A–C). Notably, the intraneuronal Aβ staining was comparable between the ipsilateral and contralateral hippocampus (Figure 6, A–C). However, by 72 hours after injection both Aβ pools were markedly reduced in the ipsilateral hippocampus (Figure 6, D–F). Therefore, these results indicate that extracellular plaques are cleared first, followed thereafter by the clearance of the intraneuronal Aβ pool. It is critical to note that the removal of Aβ is not a generalized consequence of the antibody injection as we previously showed that injection of other antibodies, such as the human tau-specific antibody HT7 (monoclonal anti-mouse) or a protozoan antibody, does not alter Aβ pathology.17 Moreover, we also showed that injection of an anti-Aβ antibody does not mask the epitope during immunohistochemistry because the reduction in Aβ deposits is also observed by thioflavine S staining.17
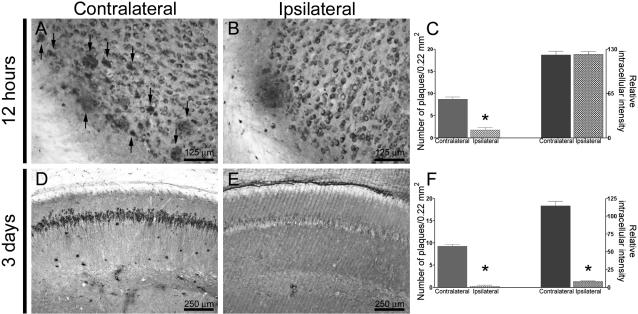
Clearance of the intracellular and extracellular Aβ pools is hierarchical. The monoclonal anti-Aβ antibody 1560 was unilaterally injected into the left hippocampus of 12- to 14-month-old 3xTg-AD mice, and the mice were subsequently sacrificed at different time points (n = 4 per time point). A–C: The ipsilateral hippocampus shows a reduction in plaque number compared to the contralateral, uninjected hippocampus 12 hours after a single intrahippocampal injection of an anti-Aβ antibody (P < 0.001), however, the intraneuronal Aβ immunoreactivity was not different between the ipsilateral and contralateral hippocampi (P = 0.887). Arrows in A point to several extracellular plaques present in the contralateral hippocampus. D–F: Areas surrounding the injection site show virtually no extracellular Aβ deposits and a marked reduction in intraneuronal Aβ immunoreactivity by 3 days after antibody administration compared to contralateral uninjected hippocampi (P < 0.001 for both number of plaques and intraneuronal Aβ immunoreactivity).
To determine whether, after the antibody diffuses, intraneuronal Aβ and extracellular plaques re-emerge concurrently or consecutively, we sacrificed 3xTg-AD mice 15, 30, and 45 days after antibody administration. At 15 days after antibody administration, we found that the intraneuronal Aβ pathology retrogressed and was comparable to the contralateral hippocampus. In contrast, at this time point no extracellular deposits were evident by immunohistochemistry, even though they were readily apparent in the contralateral hippocampus (Figure 7, A–C). By 30 days, however, the intracellular and extracellular Aβ pathologies were similar between the ipsilateral and contralateral hippocampi (Figure 7, A–C). Although we cannot exclude that glial-mediated clearance or other mechanisms may differentially influence intracellular and extracellular Aβ clearance, these data suggest that the accumulation of extracellular plaques may be, at least in part, dependent on the accumulation of intraneuronal Aβ.
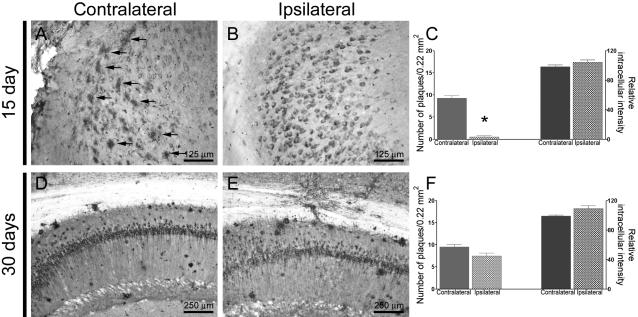
Intracellular Aβ re-emerges before extracellular Aβ. A–C: Fifteen days after antibody administration, intraneuronal Aβ immunoreactivity is detectable in areas surrounding the injection site and is comparable to the contralateral, uninjected hippocampus (P = 0.214). No extracellular Aβ deposits are apparent in the area surrounding the injection site, whereas numerous plaques are present in the contralateral site (P < 0.001). In contrast, in the contralateral, uninjected hippocampus intraneuronal and extraneuronal Aβ deposits are both readily apparent (arrows in A). D–F: Intracellular and extracellular Aβ immunoreactivity appear comparable between the ipsilateral and contralateral hippocampi (P = 0.09 and P = 0.1, for plaques and intraneuronal Aβ immunoreactivity, respectively), 30 days after a single intrahippocampal injection (n = 4 per time point). Therefore, these data suggest that the development of the extracellular pathology is in part dependent on the accumulation of intraneuronal Aβ.
Discussion
Extracellular amyloid plaques have long been considered one of the two major neuropathological hallmarks of AD.1 Despite extensive advances in our understanding of the biochemistry of APP processing/Aβ formation, there is a paucity of studies that have addressed the steps leading to extracellular Aβ deposition in the brain parenchyma. Transgenic models offer the best insight into this process because it is possible to chart the relationship between the various pools of Aβ. In this regard, immunotherapeutic approaches have proven to be a feasible means of rapidly and effectively clearing Aβ from the mammalian brain and thereby helping to elucidate the process of Aβ deposition.
The inverse relationship between plaque number and intraneuronal Aβ load and the immunotherapy results suggest that these two pools of Aβ may be dynamically related, as clearance of one pool affects the clearance of the other. Notably, our findings of an age-dependent decrease in intraneuronal Aβ pathology but an increase in extracellular Aβ in the 3xTg-AD brains are consistent with studies in human Down’s syndrome brains.16 More significantly these studies provide compelling experimental evidence that these two pools of Aβ are related and that the accumulation of extracellular Aβ may at least, in part, be dependent on the accumulation of intraneuronal Aβ.
We previously showed a strong relationship between intraneuronal Aβ and the onset of cognitive impairments in the 3xTg-AD mice.12 Although we show that intracellular Aβ levels decrease as a function of age, the cognitive impairments in the 3xTg-AD continue to worsen as a function of age (L.M. Billings et al, manuscript in preparation). In this regard, the number of thioflavine-positive plaques and the number of tangles present in the 3xTg-AD mice increases as a function of age, accounting for the worsening of the cognitive impairments. In other words, the intraneuronal Aβ accumulation causes the onset of the cognitive impairments in the 3xTg-AD mice and as the mice age, the appearance of plaques and tangles further exacerbate the cognitive impairments even if the intraneuronal Aβ immunoreactivity decreases. Behavioral deficits also occur in transgenic mice with little or no intracellular Aβ; however, these cases should not be considered contradictory with transgenics in which intraneuronal Aβ is responsible for cognitive impairments. Considering the complexity of cognition, it is likely that distinct triggers can lead to similar impairments in different transgenic mice.
The data presented here, however, should not be construed to imply that the formation of extracellular plaques can only occur after the intraneuronal accumulation of Aβ. On the contrary, plaque formation is likely to be a complex process that may occur via several independent pathways. For example, Herzig and colleagues28 recently showed that transgenic mice that overexpress APP harboring the Dutch mutation in neurons develop cerebrovascular amyloidosis and smooth muscle cell degeneration; they showed that intraneuronal Aβ is not required for the muscle cell degeneration and that extracellular neuron-derived Aβ can be imported to and accumulate in the vasculature. Therefore, at least some extracellular Aβ does not appear to originate from the intracellular pool.
The movement of Aβ from one compartment to another seems to play an important role in the pathogenic process. In this regard, different studies have shown that Aβ can be removed from the brain parenchyma into the vasculature through the blood brain barrier.28–33 These results indicate the existence of an equilibrium between the Aβ levels in the blood and the brain parenchyma.34 Here we show that a similar equilibrium exists between the intracellular and extracellular Aβ pools.
Further studies are needed to elucidate the molecular mechanism underlying this equilibrium. However, it has been shown that Aβ can be transported to the synaptic terminals and released into the extracellular space where it can accumulate.35 It is possible that the intracellular accumulation of Aβ can facilitate the terminal release of this peptide into the extracellular space. After its release, it is likely that there will be a local increase in the extracellular concentration of Aβ, which is a critical determinant of plaque formation based on kinetic studies.36 We and others have shown the presence of intraneuronal Aβ in animal models13,14,37 and more importantly in human AD and Down’s syndrome cases.24,38,39 Several lines of evidence indicate that intraneuronal Aβ accumulation is a toxic event for neurons.12,13,40–43 Understanding these processes may lead to the discovery of new therapeutic targets and help to design new drugs to ameliorate this insidious disease.
Acknowledgments
We thank Levina Tran for technical assistance and the Institute for Brain Aging and Dementia for providing tissue from Tg2576 mice.
Footnotes
Address reprint requests to Frank M. LaFerla, Department of Neurobiology and Behavior, University of California, Irvine, 1109 Gillespie Neuroscience Bldg., Irvine, CA 92697-4545. .ude.icu@alrefal :liam-E
Supported by the National Institute on Aging (AG0212982 to F.M.L.) and the Alzheimer’s Association (IIRG-02-3767 to F.M.L.).
References
- Selkoe DJ. Alzheimer’s disease: genes, proteins, and therapy. Physiol Rev. 2001;81:741–766. [Abstract] [Google Scholar]
- Hardy J, Selkoe DJ. The amyloid hypothesis of Alzheimer’s disease: progress and problems on the road to therapeutics. Science. 2002;297:353–356. [Abstract] [Google Scholar]
- Hartmann T, Bieger SC, Bruhl B, Tienari PJ, Ida N, Allsop D, Roberts GW, Masters CL, Dotti CG, Unsicker K, Beyreuther K. Distinct sites of intracellular production for Alzheimer’s disease A beta40/42 amyloid peptides. Nat Med. 1997;3:1016–1020. [Abstract] [Google Scholar]
- Wild-Bode C, Yamazaki T, Capell A, Leimer U, Steiner H, Ihara Y, Haass C. Intracellular generation and accumulation of amyloid beta-peptide terminating at amino acid 42. J Biol Chem. 1997;272:16085–16088. [Abstract] [Google Scholar]
- Skovronsky DM, Doms RW, Lee VM. Detection of a novel intraneuronal pool of insoluble amyloid beta protein that accumulates with time in culture. J Cell Biol. 1998;141:1031–1039. [Europe PMC free article] [Abstract] [Google Scholar]
- Xu H, Sweeney D, Wang R, Thinakaran G, Lo AC, Sisodia SS, Greengard P, Gandy S. Generation of Alzheimer beta-amyloid protein in the trans-Golgi network in the apparent absence of vesicle formation. Proc Natl Acad Sci USA. 1997;94:3748–3752. [Europe PMC free article] [Abstract] [Google Scholar]
- Cook DG, Forman MS, Sung JC, Leight S, Kolson DL, Iwatsubo T, Lee VM, Doms RW. Alzheimer’s A beta(1-42) is generated in the endoplasmic reticulum/intermediate compartment of NT2N cells. Nat Med. 1997;3:1021–1023. [Abstract] [Google Scholar]
- Wilson CA, Doms RW, Zheng H, Lee VM. Presenilins are not required for A beta 42 production in the early secretory pathway. Nat Neurosci. 2002;5:849–855. [Abstract] [Google Scholar]
- Tseng BP, Kitazawa M, LaFerla FM. Amyloid beta-peptide: the inside story. Curr Alzheimer Res. 2004;1:231–239. [Abstract] [Google Scholar]
- Kawarabayashi T, Shoji M, Younkin LH, Wen-Lang L, Dickson DW, Murakami T, Matsubara E, Abe K, Ashe KH, Younkin SG. Dimeric amyloid beta protein rapidly accumulates in lipid rafts followed by apolipoprotein E and phosphorylated tau accumulation in the Tg2576 mouse model of Alzheimer’s disease. J Neurosci. 2004;24:3801–3809. [Europe PMC free article] [Abstract] [Google Scholar]
- Westerman MA, Cooper-Blacketer D, Mariash A, Kotilinek L, Kawarabayashi T, Younkin LH, Carlson GA, Younkin SG, Ashe KH. The relationship between Abeta and memory in the Tg2576 mouse model of Alzheimer’s disease. J Neurosci. 2002;22:1858–1867. [Europe PMC free article] [Abstract] [Google Scholar]
- Billings LM, Oddo S, Green KN, McGaugh JL, Laferla FM. Intraneuronal Abeta causes the onset of early Alzheimer’s disease-related cognitive deficits in transgenic mice. Neuron. 2005;45:675–688. [Abstract] [Google Scholar]
- Oddo S, Caccamo A, Shepherd JD, Murphy MP, Golde TE, Kayed R, Metherate R, Mattson MP, Akbari Y, LaFerla FM. Triple-transgenic model of Alzheimer’s disease with plaques and tangles: intracellular Abeta and synaptic dysfunction. Neuron. 2003;39:409–421. [Abstract] [Google Scholar]
- Oddo S, Caccamo A, Kitazawa M, Tseng BP, LaFerla FM. Amyloid deposition precedes tangle formation in a triple transgenic model of Alzheimer’s disease. Neurobiol Aging. 2003;24:1063–1070. [Abstract] [Google Scholar]
- Oddo S, Caccamo A, Green KN, Liang K, Tran L, Chen Y, Leslie FM, LaFerla FM. Chronic nicotine administration exacerbates tau pathology in a transgenic model of Alzheimer’s disease. Proc Natl Acad Sci USA. 2005;102:3046–3051. [Europe PMC free article] [Abstract] [Google Scholar]
- Mori C, Spooner ET, Wisniewsk KE, Wisniewski TM, Yamaguch H, Saido TC, Tolan DR, Selkoe DJ, Lemere CA. Intraneuronal Abeta42 accumulation in Down syndrome brain. Amyloid. 2002;9:88–102. [Abstract] [Google Scholar]
- Oddo S, Billings L, Kesslak JP, Cribbs DH, LaFerla FM. Abeta immunotherapy leads to clearance of early, but not late, hyperphosphorylated tau aggregates via the proteasome. Neuron. 2004;43:321–332. [Abstract] [Google Scholar]
- Hsiao K, Chapman P, Nilsen S, Eckman C, Harigaya Y, Younkin S, Yang F, Cole G. Correlative memory deficits, Abeta elevation, and amyloid plaques in transgenic mice. Science. 1996;274:99–102. [Abstract] [Google Scholar]
- Smith IF, Hitt B, Green KN, Oddo S, LaFerla FM. Enhanced caffeine-induced Ca2+ release in the 3xTg-AD mouse model of Alzheimer’s disease. J Neurochem. 2005;94:1711–1718. [Abstract] [Google Scholar]
- Leissring MA, Farris W, Chang AY, Walsh DM, Wu X, Sun X, Frosch MP, Selkoe DJ. Enhanced proteolysis of beta-amyloid in APP transgenic mice prevents plaque formation, secondary pathology, and premature death. Neuron. 2003;40:1087–1093. [Abstract] [Google Scholar]
- Selkoe DJ. Clearing the brain’s amyloid cobwebs. Neuron. 2001;32:177–180. [Abstract] [Google Scholar]
- Iwata N, Tsubuki S, Takaki Y, Shirotani K, Lu B, Gerard NP, Gerard C, Hama E, Lee HJ, Saido TC. Metabolic regulation of brain Abeta by neprilysin. Science. 2001;292:1550–1552. [Abstract] [Google Scholar]
- Caccamo A, Oddo S, Sugarman MC, Akbari Y, LaFerla FM. Age- and region-dependent alterations in Abeta-degrading enzymes: implications for Abeta-induced disorders. Neurobiol Aging. 2005;26:645–654. [Abstract] [Google Scholar]
- Gyure KA, Durham R, Stewart WF, Smialek JE, Troncoso JC. Intra-neuronal abeta-amyloid precedes development of amyloid plaques in Down syndrome. Arch Pathol Lab Med. 2001;125:489–492. [Abstract] [Google Scholar]
- Lee HJ, Jung KM, Huang YZ, Bennett LB, Lee JS, Mei L, Kim TW. Presenilin-dependent gamma-secretase-like intramembrane cleavage of ErbB4. J Biol Chem. 2002;277:6318–6323. [Abstract] [Google Scholar]
- Tian G, Sobotka-Briner CD, Zysk J, Liu X, Birr C, Sylvester MA, Edwards PD, Scott CD, Greenberg BD. Linear non-competitive inhibition of solubilized human gamma-secretase by pepstatin A methylester, L685458, sulfonamides, and benzodiazepines. J Biol Chem. 2002;277:31499–31505. [Abstract] [Google Scholar]
- Ni CY, Murphy MP, Golde TE, Carpenter G. Gamma-secretase cleavage and nuclear localization of ErbB-4 receptor tyrosine kinase. Science. 2001;294:2179–2181. [Abstract] [Google Scholar]
- Herzig MC, Winkler DT, Burgermeister P, Pfeifer M, Kohler E, Schmidt SD, Danner S, Abramowski D, Sturchler-Pierrat C, Burki K, van Duinen SG, Maat-Schieman ML, Staufenbiel M, Mathews PM, Jucker M. Abeta is targeted to the vasculature in a mouse model of hereditary cerebral hemorrhage with amyloidosis. Nat Neurosci. 2004;7:954–960. [Abstract] [Google Scholar]
- DeMattos RB, Bales KR, Cummins DJ, Paul SM, Holtzman DM. Brain to plasma amyloid-beta efflux: a measure of brain amyloid burden in a mouse model of Alzheimer’s disease. Science. 2002;295:2264–2267. [Abstract] [Google Scholar]
- DeMattos RB, Bales KR, Cummins DJ, Dodart JC, Paul SM, Holtzman DM. Peripheral anti-A beta antibody alters CNS and plasma A beta clearance and decreases brain A beta burden in a mouse model of Alzheimer’s disease. Proc Natl Acad Sci USA. 2001;98:8850–8855. [Europe PMC free article] [Abstract] [Google Scholar]
- Lee VM. Abeta immunization: moving Abeta peptide from brain to blood. Proc Natl Acad Sci USA. 2001;98:8931–8932. [Europe PMC free article] [Abstract] [Google Scholar]
- Lemere CA, Spooner ET, LaFrancois J, Malester B, Mori C, Leverone JF, Matsuoka Y, Taylor JW, DeMattos RB, Holtzman DM, Clements JD, Selkoe DJ, Duff KE. Evidence for peripheral clearance of cerebral Abeta protein following chronic, active Abeta immunization in PSAPP mice. Neurobiol Dis. 2003;14:10–18. [Abstract] [Google Scholar]
- Matsuoka Y, Saito M, LaFrancois J, Gaynor K, Olm V, Wang L, Casey E, Lu Y, Shiratori C, Lemere C, Duff K. Novel therapeutic approach for the treatment of Alzheimer’s disease by peripheral administration of agents with an affinity to beta-amyloid. J Neurosci. 2003;23:29–33. [Europe PMC free article] [Abstract] [Google Scholar]
- Zlokovic BV. Clearing amyloid through the blood-brain barrier. J Neurochem. 2004;89:807–811. [Abstract] [Google Scholar]
- Lazarov O, Lee M, Peterson DA, Sisodia SS. Evidence that synaptically released beta-amyloid accumulates as extracellular deposits in the hippocampus of transgenic mice. J Neurosci. 2002;22:9785–9793. [Europe PMC free article] [Abstract] [Google Scholar]
- Jarrett JT, Lansbury PT., Jr Seeding “one-dimensional crystallization” of amyloid: a pathogenic mechanism in Alzheimer’s disease and scrapie? Cell. 1993;73:1055–1058. [Abstract] [Google Scholar]
- Echeverria V, Ducatenzeiler A, Alhonen L, Janne J, Grant SM, Wandosell F, Muro A, Baralle F, Li H, Duff K, Szyf M, Cuello AC. Rat transgenic models with a phenotype of intracellular Abeta accumulation in hippocampus and cortex. J Alzheimers Dis. 2004;6:209–219. [Abstract] [Google Scholar]
- Gouras GK, Tsai J, Naslund J, Vincent B, Edgar M, Checler F, Greenfield JP, Haroutunian V, Buxbaum JD, Xu H, Greengard P, Relkin NR. Intraneuronal Abeta42 accumulation in human brain. Am J Pathol. 2000;156:15–20. [Europe PMC free article] [Abstract] [Google Scholar]
- LaFerla FM, Troncoso JC, Strickland DK, Kawas CH, Jay G. Neuronal cell death in Alzheimer’s disease correlates with apoE uptake and intracellular Abeta stabilization. J Clin Invest. 1997;100:310–320. [Europe PMC free article] [Abstract] [Google Scholar]
- LaFerla FM, Tinkle BT, Bieberich CJ, Haudenschild CC, Jay G. The Alzheimer’s A beta peptide induces neurodegeneration and apoptotic cell death in transgenic mice. Nat Genet. 1995;9:21–30. [Abstract] [Google Scholar]
- Zhang Y, McLaughlin R, Goodyer C, LeBlanc A. Selective cytotoxicity of intracellular amyloid beta peptide1-42 through p53 and Bax in cultured primary human neurons. J Cell Biol. 2002;156:519–529. [Europe PMC free article] [Abstract] [Google Scholar]
- D’Andrea MR, Nagele RG, Wang HY, Peterson PA, Lee DH. Evidence that neurones accumulating amyloid can undergo lysis to form amyloid plaques in Alzheimer’s disease. Histopathology. 2001;38:120–134. [Abstract] [Google Scholar]
- Magrane J, Smith RC, Walsh K, Querfurth HW. Heat shock protein 70 participates in the neuroprotective response to intracellularly expressed beta-amyloid in neurons. J Neurosci. 2004;24:1700–1706. [Europe PMC free article] [Abstract] [Google Scholar]
Articles from The American Journal of Pathology are provided here courtesy of American Society for Investigative Pathology
Full text links
Read article at publisher's site: https://doi.org/10.2353/ajpath.2006.050593
Read article for free, from open access legal sources, via Unpaywall:
http://ajp.amjpathol.org/article/S0002944010620822/pdf
Citations & impact
Impact metrics
Citations of article over time
Article citations
MAD-microbial (origin of) Alzheimer's disease hypothesis: from infection and the antimicrobial response to disruption of key copper-based systems.
Front Neurosci, 18:1467333, 02 Oct 2024
Cited by: 0 articles | PMID: 39416952 | PMCID: PMC11480022
Review Free full text in Europe PMC
Regional AT-8 reactive tau species correlate with intracellular Aβ levels in cases of low AD neuropathologic change.
Acta Neuropathol, 147(1):40, 14 Feb 2024
Cited by: 0 articles | PMID: 38353753 | PMCID: PMC10866780
Modulation of hippocampal protein expression by a brain penetrant biologic TNF-α inhibitor in the 3xTg Alzheimer's disease mice.
J Transl Med, 22(1):291, 18 Mar 2024
Cited by: 0 articles | PMID: 38500108 | PMCID: PMC10946165
Mitochondria-sequestered Aβ renders synaptic mitochondria vulnerable in the elderly with a risk of Alzheimer disease.
JCI Insight, 8(22):e174290, 22 Nov 2023
Cited by: 1 article | PMID: 37991017 | PMCID: PMC10721326
SARS-CoV-2 amyloid, is COVID-19-exacerbated dementia an amyloid disorder in the making?
Front Dement, 2:1233340, 06 Jul 2023
Cited by: 3 articles | PMID: 39081980 | PMCID: PMC11285677
Go to all (166) article citations
Data
Similar Articles
To arrive at the top five similar articles we use a word-weighted algorithm to compare words from the Title and Abstract of each citation.
Intracellular amyloid-β accumulation in calcium-binding protein-deficient neurons leads to amyloid-β plaque formation in animal model of Alzheimer's disease.
J Alzheimers Dis, 29(3):615-628, 01 Jan 2012
Cited by: 35 articles | PMID: 22269161
Intraneuronal APP, not free Aβ peptides in 3xTg-AD mice: implications for tau versus Aβ-mediated Alzheimer neurodegeneration.
J Neurosci, 31(21):7691-7699, 01 May 2011
Cited by: 76 articles | PMID: 21613482 | PMCID: PMC3118598
AβPP accumulation and/or intraneuronal amyloid-β accumulation? The 3xTg-AD mouse model revisited.
J Alzheimers Dis, 28(4):897-904, 01 Jan 2012
Cited by: 18 articles | PMID: 22112547
Intracellular and extracellular Abeta, a tale of two neuropathologies.
Brain Pathol, 15(1):66-71, 01 Jan 2005
Cited by: 40 articles | PMID: 15779238 | PMCID: PMC8095923
Review Free full text in Europe PMC