Abstract
Free full text

Antiviral Protein APOBEC3G Localizes to Ribonucleoprotein Complexes Found in P Bodies and Stress Granules
Abstract
Members of the APOBEC (apolipoprotein B mRNA-editing enzyme catalytic polypeptide 1-like) family of cytidine deaminases inhibit host cell genome invasion by exogenous retroviruses and endogenous retrotransposons. Because these enzymes can edit DNA or RNA and potentially mutate cellular targets, their activities are presumably regulated; for instance, APOBEC3G (A3G) recruitment into high-molecular-weight ribonucleoprotein (RNP) complexes has been shown to suppress its enzymatic activity. We used tandem affinity purification together with mass spectrometry (MS) to identify protein components within A3G-containing RNPs. We report that numerous cellular RNA-binding proteins with diverse roles in RNA function, metabolism, and fate determination are present in A3G RNPs but that most interactions with A3G are mediated via binding to shared RNAs. Confocal microscopy demonstrated that substantial quantities of A3G localize to cytoplasmic microdomains that are known as P bodies and stress granules (SGs) and are established sites of RNA storage and metabolism. Indeed, subjecting cells to stress induces the rapid redistribution of A3G and a number of P-body proteins to SGs. Among these proteins are Argonaute 1 (Ago1) and Argonaute 2 (Ago2), factors that are important for RNA silencing and whose interactions with A3G are resistant to RNase treatment. Together, these findings reveal that A3G associates with RNPs that are found throughout the cytosol as well as in discrete microdomains. We also speculate that the interplay between A3G, RNA-silencing pathways, and cellular sites of RNA metabolism may contribute to A3G's role as an inhibitor of retroelement mobility and as a possible regulator of cellular RNA function.
The inhibition of human immunodeficiency virus type 1 (HIV-1) infection by cellular APOBEC (apolipoprotein B mRNA-editing enzyme catalytic polypeptide 1-like) proteins, in particular human APOBEC3G (A3G), is a recently discovered mode of host-mediated antiviral resistance (14, 31, 32, 66, 74, 75, 101, 124, 130, 134). In the absence of the HIV-1 Vif (virion infectivity factor) protein, A3G is incorporated into HIV-1 particles such that it mediates the deamination of cytidine (C) to uridine (U) in nascent minus-strand reverse transcripts during infection of target cells. Excessive C-to-U editing leads to the generation of hypermutated sequences that contain multiple guanosine (G)-to-adenosine (A) transitions in their plus strands and are genetically compromised (44, 74, 133). However, the relationship between APOBEC-mediated antiviral effects and editing remains incompletely understood, as HIV-1 infection can be inhibited in the absence of cytidine deamination, where reductions in reverse transcript accumulation correlate with levels of inhibition (12, 43, 92). HIV-1 is presumed to encounter the APOBEC enzymes during natural infection, as A3G as well as the related APOBEC3F (A3F) protein is expressed in the natural targets of infection, T cells and macrophages (13, 24, 25, 55, 95, 101, 114, 124), and G-to-A-hypermutated viral sequences are readily detected in infected persons (54, 61, 93, 118).
Vif is an HIV-1-encoded regulatory protein that allows the virus to evade the antiviral activities of A3G and A3F. Vif accomplishes this by binding to A3G or A3F and bridging an interaction with a cullin5-elonginB/C-Roc1 E3 ubiquitin ligase complex (79, 131). This induces the polyubiquitylation and proteasomal degradation of A3G or A3F, such that the enzymes are removed from infected cells and cannot be incorporated into viral particles (28, 76, 79, 102, 108, 124, 131, 134). There is also evidence to suggest that Vif may impede the packaging of A3G or that A3G translation may also be inhibited (56, 75, 108).
A3G and A3F belong to the mammalian APOBEC family of cytidine deaminases, of which at least nine other members appear to be expressed in humans (29, 45, 120). Two well-characterized members are APOBEC1, which edits apolipoprotein B mRNA at a single site to introduce a premature stop codon (89, 112, 129), and AID (activation-induced cytidine deaminase), which promotes antibody diversification through hypermutation and the induction of class switch recombination (48, 49, 87, 91). In each of these cases, it is evident that interactions with other cellular factors are critical for determining RNA or DNA substrate selection as well as target site specificity (9, 21, 64, 69, 72, 73, 80). It has also been inferred that APOBEC1 and AID may mutate cellular DNA and therefore contribute to cell transformation (3, 10, 46). Accordingly, it is to be expected that cells harbor mechanisms to regulate these activities and protect their genomes, perhaps by posttranslationally modifying these enzymes (e.g., by ubiquitylation), by sequestering them away from nuclear DNA as inactive complexes, and/or by confining them to the cytoplasm.
Although substrates of viral origin, including retroviruses, the hepadnavirus hepatitis B virus, and the parvovirus adeno-associated virus, have been identified for A3G and other APOBEC3 proteins (23, 31, 45, 114), critical questions that remain are whether these cytidine deaminases act on cellular targets and, more generally, whether they possess physiological functions independent of their antiviral activities. Indeed, recent studies support the idea that one cellular function of APOBEC3 proteins may be to restrict the retrotransposition of endogenous (intracellular) retroelements such as musD, IAP, Line 1, Alu, and Ty1 (15, 16, 23, 33, 34, 86, 99, 107). However, it remains to be established whether A3G and other APOBEC3 proteins can target or edit additional cellular sequences (e.g., those unrelated to viral elements) and how these activities may be positively or negatively regulated through interactions with cellular binding partners. In this regard, recent studies with a variety of cell backgrounds revealed that A3G associates with high-molecular-weight complexes in activated CD4+ T cells and immortalized cell lines but not in quiescent CD4+ T cells, where it is found in low-molecular-weight complexes. Interestingly, the enzymatic activity of A3G that is present in high-molecular-weight complexes is inhibited but can be activated by treatment with RNase in vitro, a procedure that also results in complex disassembly (22, 25). These observations suggest that the recruitment of A3G into large ribonucleoprotein (RNP) complexes may represent one mechanism for regulating its activity in cells.
The initial objective of this study was to identify potential cellular cofactors for A3G that may regulate, or contribute to, its cellular and antiviral functions. Proteins complexed with A3G were isolated using a tandem-affinity purification (TAP) approach and identified by mass spectrometry (MS). Interestingly, most of the proteins that were identified are established RNA-binding proteins. Among these were a number of proteins whose presence in RNP complexes has been associated with various states of mRNA translational competence. Coimmunoprecipitation experiments performed in the presence or absence of RNase treatment confirmed that most interactions with A3G are RNA dependent, suggesting that they are indirect and are “bridged” by RNAs. Having identified a profile of A3G-associated proteins, we next investigated their cellular localization in relation to A3G. We found that A3G is not only dispersed throughout the cytosol but also markedly concentrated in cytoplasmic foci that were identified as mRNA-processing bodies (P bodies). We also report that heat shock induces the redistribution of A3G and certain P-body proteins towards other RNP-rich cytoplasmic microdomains termed stress granules (SGs). Significantly, the interactions between A3G and a subset of these P-body proteins that are also important for RNA silencing, namely, Argonaute 1 (Ago1) and Argonaute 2 (Ago2) (39), were found to be partially resistant to RNase treatment, perhaps reflecting the importance of direct protein-protein contacts in complex formation. Together, these results suggest that A3G is associated with dynamic cytoplasmic RNP complexes that may participate in regulating the cytoplasmic localization and posttranscriptional fate of cellular RNAs.
MATERIALS AND METHODS
Expression vectors.
The pcDNA3.1-based and pCMV4-based vectors for expressing untagged or hemagglutinin (HA)-tagged human A3G, pA3G, and pA3G-HA, the HIV-1 Vif expression vector, pcVIF, the HIV-1 Gag-Pol-packaging vector, ∂CMVp8.91, the vesicular stomatitis virus G (VSV-G) protein expression vector, pMDG VSV-G, and the green fluorescent protein (GFP)-encoding HIV-1 vector pHR′SINcPPT-SGW have been described previously (8, 44, 88, 101, 102). Plasmids expressing full-length A3G with a TAP tag at the N or C terminus (NTAP or CTAP, respectively) were constructed by inserting PCR-amplified A3G cDNAs into pNTAP and pCTAP (Interplay Mammalian TAP system; Stratagene) by use of EcoRI and XhoI to generate pNTAP-A3G and pCTAP-A3G, respectively. The NTAP-A3G insert was also cloned into the HpaI site of pCMS28, a modified MIGR1-based murine retroviral vector that confers resistance to puromycin, to create pCMS28/NTAP-A3G. The N-terminally myc-tagged pmyc-A3G vector was constructed by inserting the A3G cDNA as an EcoRI-XhoI fragment into pCMV3B (Stratagene). pYFP-A3G and pCFP-A3G express A3G fused to the C termini of yellow fluorescent protein (YFP) or cyan fluorescent protein (CFP), respectively, and were constructed using PCR with pCMV3B (Stratagene) as the starting vector. Plasmids expressing N-terminally myc-tagged proteins (YB-1, Hu antigen R [HuR], heterogeneous nuclear ribonucleoprotein [hnRNP] U, and Mov10) were constructed by cloning PCR products into pCMV3B, whereas vectors for the expression of C-terminally myc-tagged proteins (PABPC1) were based on pCMV5A (Stratagene). Expression vectors for VSV-G-tagged Ro60 and La were a kind gift of J. M. Pruijn, the pSRP68-GFP construct was a kind gift of T. Pederson, and the expression vector for LRP130 was generously provided by S. Pinol-Roma. Plasmids expressing P-body or SG markers were generously provided by the following: G. Hannon, who provided plasmids encoding myc-tag Ago1 and Ago2; J. Lykke-Andersen, who provided pcDNA3-Myc-hDcp1a; E. K. Chan, who provided the plasmid encoding GFP-GW182; D. Weil, who provided pCMV-RFP-hp54; and N. Kedersha and P. Anderson, who provided pEYFP-TIA-1 and pmRFP-Dcp1a.
Cells and cell lines.
Human 293T cells and HeLa cells were maintained under standard conditions. CEM-SS cells stably expressing NTAP-3G were generated by standard retrovirus-mediated transduction (40) using pCMS28/NTAP-A3G, or untagged A3G as a control, and selection with 0.5 μg/ml puromycin. Expression of the NTAP-A3G fusion protein was confirmed by immunoblotting. Peripheral blood mononuclear cells (PBMCs) were obtained by venipuncture, isolated using a Ficoll gradient, stimulated with 5 μg/ml phytohemagglutinin-L, and maintained in medium supplemented with interleukin-2 (105).
Virus infectivity.
HIV-1 GFP reporter viruses were prepared using 60-mm-diameter monolayers of 293T cells by cotransfection of ∂CMVp8.91, pMDG VSV-G, and pHR′SINcPPT-SGW (5 μg, 3 μg, and 5 μg, respectively) together with 1 μg of control vector (pcDNA3.1, pNTAP, or pCTAP) or an expression vector for A3G (pA3G, pNTAP-A3G, or pCTAP-A3G). Virus-containing supernatants were harvested at ~48 h posttransfection and filtered, and p24Gag concentrations were measured by enzyme-linked immunosorbent assay. Sixteen-millimeter-diameter HeLa cell monolayers were challenged with normalized viral stocks, and relative infectivity was determined at ~24 h by flow cytometry (FACSCalibur) by measuring the number of GFP-positive cells. Values are presented as percentages of infectivity relative to the value obtained for virus produced in the presence of pcDNA3.1.
Expression and purification of tagged A3G-containing complexes.
A3G-containing complexes were isolated using the InterPlay mammalian TAP system according to the manufacturer's instructions (Stratagene). Briefly, two 150-mm-diameter cultures of 70% confluent 293T cells were transfected with 2 μg of pNTAP-A3G or pCTAP-A3G (or pNTAP or pA3G as controls) and harvested at ~40 h; for purification from the CEM-SS line stably expressing NTAP-A3G, 2 × 108 cells were used. Following harvest, cells were washed three times in phosphate-buffered saline (PBS) and lysed in lysis buffer by three successive rounds of freezing in dry ice and thawing. Cell extracts were centrifuged for 10 min at 16,000 × g at 4°C, the supernatants passed over streptavidin resin, and eluted complexes bound to calmodulin coated-beads. Following elution from the calmodulin column, proteins were precipitated with methanol and chloroform (121) and resuspended in gel-loading buffer. The samples were resolved on 4 to 10% sodium dodecyl sulfate-polyacrylamide gel electrophoresis (SDS-PAGE) gels, which were then fixed in 50% (vol/vol) methanol, 10% (vol/vol) acetic acid, and 10% fixative enhancer (Bio-Rad) and stained using a Silver Stain Plus kit (Bio-Rad). In experiments that also contained Vif, the 293T cells were cotransfected with 4 μg of pcVIF and 2 μg of pNTAP-A3G and treated with 10 μM MG132 (a proteasome inhibitor) for 18 h prior to lysis. In some experiments, cell lysates were treated with 10 μg/ml RNase A (Sigma) for 60 min at room temperature before purification of the NTAP-A3G-containing complexes.
Mass spectrometry.
In-gel reduction, alkylation, and trypsin digestion were performed using standard procedures prior to subsequent analysis by mass spectrometry, as described previously (126). Peptides were extracted from the gel pieces by a series of acetonitrile and aqueous washes, lyophilized, and resuspended in 50 mM ammonium bicarbonate before analysis by liquid chromatography-tandem MS. Reversed-phase chromatography separations of peptides were performed using 75-μm C18 PepMap columns and an Ultimate liquid chromatography system (Dionex, United Kingdom). Peptides were then ionized by electrospray ionization using a Z-spray source fitted to a Q-Tof micro instrument (Waters Corp.), and the tandem MS analyses were conducted using collision energy profiles that were chosen based on the m/z ratio and the charge state of the peptide. The mass spectral data were processed into peak lists (containing the precursor ion m/z and charge state and the m/z and intensity of the fragment ions) and searched against the SwissProt and NCBI nonredundant databases using Mascot software (Matrix Science, United Kingdom). All results were manually verified.
Coimmunoprecipitation and immunoblotting.
Thirty-five-millimeter-diameter cultures of 293T cells were transiently transfected with 1.5 μg of the plasmid encoding the protein to be immunoprecipitated (pA3G-HA, pmyc-YB-1, or the empty vector control) and 3 μg of vector expressing the protein of interest by use of polyethylenimine (Polysciences, Inc.) at a ratio of 3 μg to 1 μg DNA. At 24 h posttransfection, cells were lysed in immunoprecipitation (IP) buffer (1% NP-40, 150 mM NaCl, 50 mM Tris-HCl [pH 7.5], 1 mM EDTA), and complete protease inhibitor cocktail (Roche) for 30 min at 4°C and then cleared by centrifugation for 15 min at 18,000 × g at 4°C. For coimmunoprecipitation assays, protein A or G agarose (Invitrogen) was preincubated with an anti-HA (mouse monoclonal; 12CA5) or anti-myc (mouse monoclonal; 9E10) antibody for 1 h at 4°C and then mixed with cell lysate for 4 h at 4°C on a rotator. Thereafter, the agarose beads were washed three times with cold IP buffer and bound complexes either eluted in gel loading buffer or treated with RNase. The latter procedure was performed by resuspending washed beads in IP buffer, dividing them into two equal volumes, adding 10 μg/ml RNase mixture (DNase free; Roche) to one, and incubating both at room temperature for 20 min. After two washes in cold IP buffer, proteins were eluted in gel-loading buffer. Samples were analyzed by SDS-PAGE using 10% gels for proteins with relative molecular masses of less than 60 kDa and 7% gels for proteins with relative molecular masses of greater than 60 kDa. Resolved proteins were detected by immunoblotting using anti-HA (mouse monoclonal HA.11; Covance Inc.), anti-myc (rabbit polyclonal ab9106; Abcam), anti-A3G (rabbit polyclonal [92]), anti-GFP (mouse monoclonal clones 7.1 and 13.1; Roche), anti-VSV-G (rabbit polyclonal, PRB-192P; Covance) or anti-LRP130 (mouse monoclonal; 9C9 [83]) as primary antibodies. Bound antibodies were visualized using appropriate horseradish peroxidase-conjugated secondary antibodies and enhanced chemiluminescence (Pierce).
Immunofluorescence.
Unmodified PBMCs, H9 T cells, or CEM-SS T cells stably expressing NTAP-A3G (2 × 104 to 4 × 104) were fixed to glass slides by centrifugation for 5 min at 800 rpm using a Shandon Cytospin 4 instrument (Thermo Electron Corporation) and were air dried for 10 min at room temperature. Adherent HeLa cell monolayers were seeded onto glass coverslips (Cover Glass, 22 mm; BDH) in 35-mm-diameter plates and were transfected with 1 μg of pA3G by use of FuGENE 6 (Roche). Cells were washed three times in PBS and fixed with 4% paraformaldehyde (Electron Microscopy Sciences) in PBS for 15 min. After being washed with PBS, cells were incubated with 50 mM ammonium chloride, permeabilized with 0.5% Triton X-100 for 5 min at room temperature, and incubated for 30 min with blocking solution (4% goat serum in PBS) at room temperature. Staining was performed by incubating the cells for 60 min with an A3G-specific antibody raised in rabbits (92) at a 1:100 dilution, washing them with 10% fetal calf serum in PBS, and incubating them for 45 min with an Alexa Fluor 594-conjugated goat anti-rabbit immunoglobulin G (Molecular Probes) at a 1:1,000 dilution. To visualize the nuclei, cells were additionally stained for 5 min with 4′,6′-diamidino-2-phenylindole (DAPI) dilactate (Molecular Probes) at a 1:25,000 dilution. Samples were mounted using Mowiol (Calbiochem), and images were collected with a confocal laser scanning microscope (DM IRE2; Leica) and processed with the LCS (version 2.02) software (Leica) and Adobe Photoshop (version 6.0). Images are Z-series compilations of 6 to 10 images in a stack.
Coimmunolocalization experiments.
Subconfluent HeLa cell monolayers were seeded onto coverslips in 35-mm-diameter plates and transfected with 0.5 to 2 μg of vectors expressing myc-tagged versions of the proteins of interest, 1 μg of pYFP-A3G or pCFP-A3G, and/or 0.5 μg of pmRFP-Dcp1a as a P-body marker or pEYFP-TIA-1 as an SG marker by use of FuGENE 6. Cells were fixed and stained 24 to 36 h after transfection. SGs were induced by incubating the cells for 30 min at 44°C prior to fixation. Cells were incubated with an anti-myc (mouse monoclonal; 9E10; 1:1,500 dilution) antibody. Alexa Fluor 488-, 546-, or 594-conjugated species-specific antibodies were used at dilutions of 1:1,000 for detection, and the coverslips were treated and analyzed by confocal microscopy (as described above). Merged images were produced using the Leica confocal software overlay function without any further image manipulation. Typically, superimposing yellow and red signals or red and green signals results in an orange signal, superimposing cyan and yellow results in a white signal, and superimposing red and cyan results in a pale pink signal. However, our experience is that the exact color of merged signals depends on the intensity of each contributing signal.
RESULTS
Purification of A3G-containing ribonucleoprotein complexes.
To purify proteins associated with A3G, we used a TAP technique (97). This method uses a double-affinity purification procedure that involves tagging the protein of interest with the streptavidin-binding peptide and the calmodulin-binding peptide. The target protein, potentially in a complex with interacting factors, can then be purified from expressing cells by two consecutive affinity purification steps using mild conditions. Because the addition of peptide sequences to the N or C termini of proteins has the potential to perturb biological function, it was important to confirm that our A3G fusion proteins retained antiviral activity. GFP-encoding HIV-1-based vector stocks were prepared in 293T cells in the presence of the A3G expression vectors pA3G, pNTAP-A3G, or pCTAP-A3G (or matching control vectors) and used in single-cycle challenges of HeLa cells (Fig. (Fig.1A).1A). Infectivities were monitored as the percentages of cells that expressed GFP, and the values were normalized using the sample containing the empty vector pcDNA3.1 as the reference. Importantly, the antiviral phenotype of each of the TAP-tagged A3G proteins was robust and therefore similar to that of the wild-type A3G protein, thus confirming the potential suitability of this approach for identifying A3G-interacting factors. The immunoblot analysis at the bottom of Fig. Fig.1A1A demonstrates that these fusion proteins are expressed in cells to levels similar to those for untagged A3G.
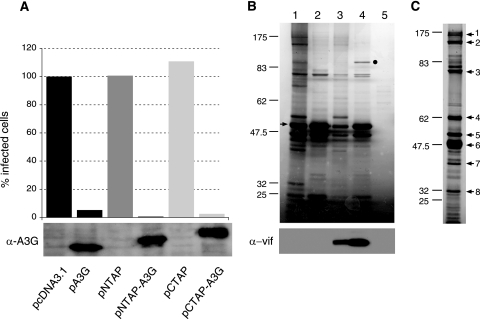
Purification of proteins associated with A3G RNP complexes. (A) Effect of A3G, NTAP-A3G, and CTAP-A3G on HIV-1 infectivity. GFP reporter viruses were produced in 293T cells in the absence of Vif and in the presence of either A3G, NTAP-A3G, CTAP-A3G, or a control vector. Normalized viral stocks were used to challenge HeLa cells, and infectivity was scored by assessing GFP expression using flow cytometry. Values are presented as percent infectivity relative to that for virus produced in the absence of A3G. Data are the means of two independent experiments. The lower panel shows an immunoblot analysis confirming comparable levels of expression of A3G and TAP-tagged A3G in virus-producing 293T cells. (B) TAP tag purification of A3G RNP complexes from 293T cells. Cultured monolayers were transfected with pNTAP-A3G alone (lanes 1 and 2), with pNTAP-A3G and pcVIF (lanes 3 and 4), or with the empty vector pNTAP (lane 5). Cell lysates were prepared and treated with RNase A for 1 h at room temperature (lanes 2 and 4) or left untreated (lanes 1 and 3). NTAP-A3G complexes were purified in two steps using streptavidin-binding peptide-coated beads and then calmodulin-binding peptide-coated beads. Final samples were resolved using 10% SDS-polyacrylamide gels, and the bands were visualized by silver staining. The band indicated by an arrow corresponds to full-length NTAP-A3G, and the band indicated by a dot was identified by MS as cullin5 (lane 4). The lower panel shows an immunoblot analysis confirming the presence of Vif in NTAP-A3G complexes. (C) TAP tag purification of A3G RNP complexes from CEM-SS cells stably expressing the NTAP-A3G protein. The methods are as described for panel B. The prominent bands were excised (arrows 1 to 8), and the proteins were then identified by mass spectrometry (refer to Table Table11).
A3G-containing complexes were first purified from 293T cells transiently transfected with either pNTAP-A3G or pCTAP-A3G. As controls, parallel purifications were performed with cells transfected with the parental pNTAP or pCTAP vectors or the untagged A3G expression vector, pA3G. As expected, immunoblotting confirmed that A3G was isolated only from the NTAP-A3G- or CTAP-A3G-containing samples (data not shown). The constellations of enriched cellular proteins were visualized by silver staining (Fig. (Fig.1B1B shows a typical data set). More than 20 bands were clearly visible in the NTAP-A3G sample, and the principal band corresponding to NTPA-A3G is indicated (Fig. (Fig.1B,1B, lane 1). Though the profile of copurifying bands from CTAP-A3G-expressing lysates was very similar, indicating that the positioning of the affinity tags on A3G did not significantly alter the composition of A3G complexes, the yields of proteins were consistently lower (data not shown); we therefore used N-terminally tagged A3G constructs thereafter.
As noted above, A3G can associate with RNA-containing complexes with high molecular weights (25). Accordingly, we wished to examine the importance of RNA for the integrity of the putative A3G-containing complexes identified here. NTAP-A3G expressing lysates were incubated with RNase A for 1 h at room temperature prior to TAP tag purification. This treatment reproducibly increased the efficiency of the purification of A3G itself (Fig. (Fig.1B,1B, compare lanes 1 and 2), suggesting that the accessibility of the TAP tag domains had been increased. Importantly, however, the majority of bands that had previously coeluted with NTAP-3G were no longer detectable following RNase treatment (Fig. (Fig.1B,1B, compare lane 2 to lane 1). We therefore concluded that RNA plays a central role in the formation of A3G-containing complexes, potentially by serving as a “scaffold” for the recruitment of multiple cellular RNA-binding proteins.
Because A3G is regulated by HIV-1 Vif through the engagement of an E3 ubiquitin ligase, we also assessed the effects of Vif expression on the formation and isolation of A3G-containing complexes. 293T cells were transfected with pNTAP-3G and analyzed as previously described, except that the cultures were also transfected with a Vif expression vector and were grown in the presence of the proteasome inhibitor MG132 for 18 h prior to harvest (Fig. (Fig.1B,1B, lanes 3 and 4; the lower panel confirms the presence of Vif). In the absence of RNase treatment, the pattern of interacting factors was much the same as those for samples lacking Vif, except that the band intensities were somewhat lower, presumably because of incomplete inhibition of the proteasome pathway (Fig. (Fig.1B,1B, lanes 1 and 3). As before, RNase treatment of the cell lysate improved the efficiency of complex purification (Fig. (Fig.1B,1B, compare lane 4 to lane 3). Interestingly, one band of ~90 kDa was very prominent in this particular sample (Fig. (Fig.1B,1B, lane 4); mass spectrometry identified this protein as cullin5, a previously defined core component of the cullin5-elonginB/C-Roc1 E3 ubiquitin ligase complex that mediates A3G ubiquitination (78, 131). This latter finding therefore served as a useful validation of our approach for identifying A3G-interacting proteins of potential biological relevance.
Identification of APOBEC3G-associated proteins.
It was conceivable that overexpression of TAP-tagged A3G in 293T cells may have led to the assembly of nonphysiological complexes. To try to address this point, we used a retrovirus vector to generate a stable cell line expressing NTAP-A3G; to avoid possible competition between endogenous A3G and the ectopic NTAP-A3G for incorporation into complexes, we used the CEM-SS cell line, as it naturally expresses very low levels of A3G (13, 101). Immunoblot analysis indicated that these CEM-SS/NTAP-A3G cells express only ~4-fold more A3G than H9 T cells, a line that naturally restricts vif-deficient HIV-1 replication (data not shown) (105). Using the same procedure as for 293T cells, NTAP-A3G-containing complexes were purified from these T cells and the isolated proteins separated by SDS-PAGE (Fig. (Fig.1C1C shows a representative gel). Most of the bands that were visible following silver staining appear to match those noted in the 293T experiments. Indeed, many were present at higher quantities relative to NTAP-A3G, particularly band 4. CEM-SS cells stably expressing untagged A3G served as a negative control for the specificity of purification (data not shown). Based on these findings, the CEM-SS/NTAP-A3G cell line became our preferred source for the isolation of A3G-associated proteins.
The eight most prominent bands, as indicated (1 to 8) in Fig. Fig.1C,1C, were excised, subjected to tryptic digestion, and analyzed by MS to identify the constituent proteins; the lower-abundance bands were not analyzed initially but will be the subject of future work. The Mascot score (94) was used to justify the accuracy of protein identification, with a score of greater than 74 indicating a significant match. Table Table11 lists the proteins present in each gel slice in descending order according to the numbers of identified peptides for each protein. Twenty-two matches corresponding to discrete proteins were identified, with A3G being a major component of bands 6 and 7 (the latter likely corresponding to a degraded A3G product). Strikingly, many of the proteins identified in this analysis are known cellular RNA-binding proteins: poly(A)-binding protein, cytoplasmic 1 (PABPC1), and various hnRNP proteins are commonly found in RNP complexes (85); HuR is an AU-rich element-binding protein that affects stability and translation regulation of mRNAs (19, 42); the 130-kDa leucine-rich PPR motif-containing protein (LRP130) has been shown to be present in RNP complexes associated with nuclear and mitochondrial RNAs (83, 84); the 60-kDa ribonucleoprotein autoantigen SS-A/Ro (Ro60) and the Sjogren syndrome antigen B (SS-B/La) proteins are core components of Ro RNP complexes (128); and Mov10 was recently described as a component of an Argonaute protein-associated RNP complex (82, 113). In addition, a number of cytoskeletal and mitochondrial proteins were also found. Because A3G's association with many factors is RNase sensitive (Fig. (Fig.1B)1B) (25), thus defining these complexes as RNP complexes, we focused our immediate attention on the RNA-binding proteins we had identified.
TABLE 1.
Mass spectrometry analysis of proteins associated with NTAP-A3G RNP complexes
Band no. | Official symbol | Common name | Accession no. | Mol mass (Da) | No. peptides matched | % Coverage | Mascot scorea |
---|---|---|---|---|---|---|---|
1 | LRPPRC | LRP130 | gi 31621305 | 157,805 | 11 | 10 | 585 |
DHX9 | RHA | Q08211 | 140,788 | 3 | 2 | 180 | |
HNRPU | hnRNP U | Q00839 | 90,423 | 2 | 4 | 136 | |
2 | MOV10 | Mov10 | Q9HCE1 | 113,599 | 28 | 38 | 1281 |
HNRPU | hnRNP U | Q00839 | 90,423 | 8 | 13 | 426 | |
3 | PABPC1 | PABPC1 | P11940 | 70,626 | 18 | 33 | 821 |
HSPA8 | HSP71 | P11142 | 70,854 | 4 | 8 | 157 | |
SYNCRIP | hnRNP Q1 | O60506 | 69,590 | 3 | 5 | 115 | |
SRP68 | SRP68 | Q9UHB9 | 70,686 | 2 | 5 | 78 | |
4 | TROVE2 | Ro60 | P10155 | 60,631 | 31 | 56 | 1362 |
MCCC2 | MCCC2 | Q9HCC0 | 61,294 | 27 | 58 | 1184 | |
PABPC1 | PABPC1 | P11940 | 70,626 | 7 | 13 | 356 | |
VIM | VIM | P08670 | 53,488 | 3 | 10 | 89 | |
PCCB | PCCB | P05166 | 58,169 | 1 | 3 | 81 | |
5 | SSB | La | P05455 | 46,808 | 24 | 47 | 912 |
APOBEC3G | A3G | Q9HC16 | 46,378 | 16 | 51 | 523 | |
K-ALPHA-1 | K-ALPHA-1 | P68363 | 50,120 | 9 | 32 | 433 | |
TUBB | TUBB | P07437 | 49,639 | 4 | 12 | 182 | |
TROVE2 | RO60 | P10155 | 60,631 | 2 | 8 | 118 | |
YBX1 | YB-1 | P67809 | 35,903 | 2 | 15 | 90 | |
6 | APOBEC3G | A3G | Q9HC16 | 46,378 | 33 | 81 | 1233 |
YBX1 | YB-1 | P67809 | 35,903 | 9 | 44 | 400 | |
7 | HNRPC | hnRNP C | P07910 | 33,667 | 15 | 33 | 741 |
APOBEC3G | A3G | Q9HC16 | 46,378 | 18 | 58 | 530 | |
HNRPA3 | hnRNP A3 | P51991 | 39,571 | 2 | 10 | 189 | |
MRPS22 | MRPS22 | P82650 | 41,254 | 3 | 10 | 125 | |
8 | SNRPA | U1A | P09012 | 31,128 | 9 | 39 | 506 |
ATP5C1 | ATP5C1 | P36542 | 32,975 | 6 | 21 | 283 | |
ELAVL1 | HuR | Q15717 | 36,069 | 4 | 13 | 230 |
RNA-dependent interactions with APOBEC3G.
The interactions of several proteins identified in NTAP-A3G complexes were further examined by coimmunoprecipitation using 293T cells cotransfected with an expression vector for HA-tagged A3G and one of several vectors each expressing an epitope-tagged version of the test protein of interest (Fig. (Fig.2).2). Following immunoprecipitation with an HA-specific antibody, samples were divided, and one half were treated with RNase. The maintenance or loss of the interaction with A3G was then evaluated by immunoblotting using antibodies specific for the epitopes present in the test proteins (upper panels).
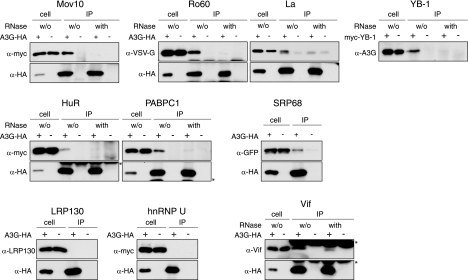
Coimmunoprecipitation of cellular proteins with A3G. Transfected 293T cells coexpressing A3G-HA (or empty vector as indicated) and tagged versions of cellular proteins (except LRP130) identified in the NTAP-A3G RNP complexes or Vif were lysed and immunoprecipitated with anti-HA or anti-myc antibodies and protein A or G linked to agarose. When indicated, the immunoprecipitates were treated with an RNase mixture (w/o, without RNase treatment). Samples were analyzed by immunoblotting using the indicated primary antibodies. In the La analyses, a background band of mobility slightly faster than that of tagged La was consistently seen for immunoprecipitated samples. For YB-1, the coimmunoprecipitation was reversed and A3G was detected on the blot. Bands indicated by asterisks correspond to immunoglobulin heavy or light chains.
Most of the proteins we tested, e.g., Mov10, Ro60, La, HuR, PABPC1, and SRP68, were specifically coimmunoprecipitated with A3G but were not recovered from the empty vector control samples (Fig. (Fig.2).2). In the case of YB-1, the experiment was performed in reverse because of the occlusion of YB-1-myc by the antibody heavy chains during immunoblotting. The cell lysates were therefore immunoprecipitated using a myc-specific antibody, and the association of A3G was then monitored by immunoblotting using an A3G-specific antibody (Fig. (Fig.2).2). Importantly, none of these particular proteins were detected in A3G-containing complexes after RNase treatment (Fig. (Fig.2),2), indicating that their presence in such complexes is dependent on RNA. The most likely explanation is that these proteins do not contact A3G directly but that they are associated with A3G-containing complexes through indirect interactions that are bridged by RNA. Similar experiments conducted with hnRNP U and LRP130 failed to confirm their presence in A3G complexes (Fig. (Fig.2).2). Whether this reflects a genuine lack of association or fortuitous copurification during TAP tag purification remains to be determined. However, it is perhaps worth noting that hnRNP U was also identified by mass spectrometry in similar affinity purification procedures of Staufen-containing RNP complexes, but its presence could not be confirmed by immunoblotting (17, 119). As a positive control for the detection of direct protein-protein interactions in these analyses, we confirmed that Vif could be coimmunoprecipitated with A3G in this experimental format and that binding was maintained following RNase treatment (Fig. (Fig.2).2). This observation was consistent with our earlier studies (Fig. (Fig.1B).1B). In sum, these coimmunoprecipitation experiments confirmed that many of the proteins identified in A3G complexes by TAP tag purification are indeed found in association with A3G, though the interactions are generally reliant upon RNA: it therefore appears reasonable to describe such complexes as A3G RNP complexes.
APOBEC3G localizes to discrete cytoplasmic foci.
Having begun to localize A3G biochemically in terms of proximity to, and interactions with, other cellular proteins, we next wished to examine localization using cell-imaging approaches, in particular confocal microscopy. Here, the literature is inconsistent, with some differing observations having been made; for instance, early results using transfected cells suggested that A3G is dispersed throughout the cytosol with no particular pattern of localization (2, 74, 76), whereas some more recent work has suggested that accumulation in cytoplasmic microdomains can occur (122). We therefore analyzed the subcellular localization of endogenous A3G in untransfected PBMCs and H9 cells by indirect immunofluorescence using an A3G-specific antibody (Fig. 3A and B, respectively). In both cell types, A3G was distributed throughout the cytoplasm, with a pronounced localization to discrete cytoplasmic foci. These varied in relative size and number from one cell to another and were not observed in parental CEM-SS cells, indicating that this staining pattern reflects the localization of the endogenous A3G protein (data not shown). Similar intense foci of A3G were also observed for the CEM-SS/NTAP-A3G line (Fig. (Fig.3C),3C), indicating that the tagging of A3G does not perturb subcellular localization, as well as for HeLa cells transfected with pA3G (Fig. (Fig.3D).3D). Finally, various fusions of A3G to fluorescent proteins, such as CFP or YFP, also localized to these cytoplasmic foci (data not shown; also see below). Importantly, appending these tags to A3G did not disrupt the ability of A3G to inhibit HIV-1 infectivity (data not shown).
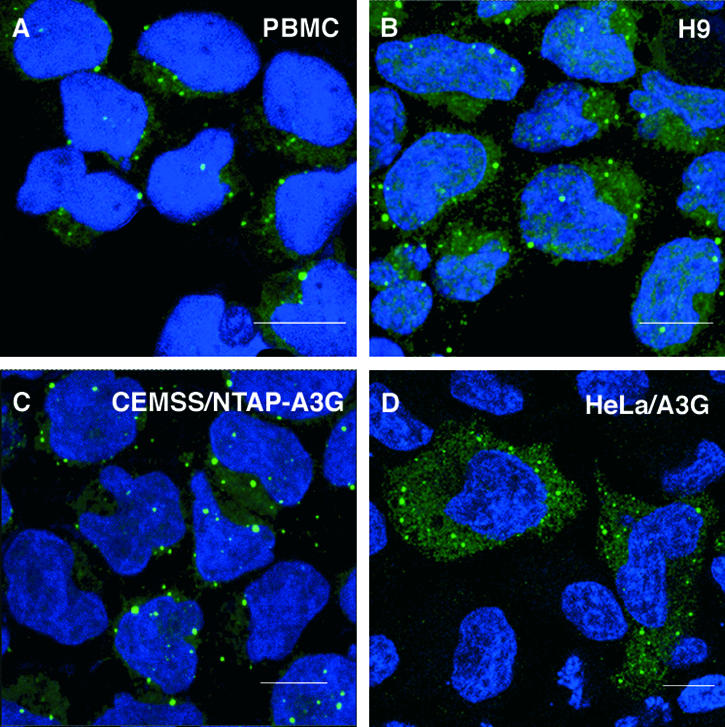
A3G is localized in the cytoplasm and is enriched in discrete cytoplasmic foci. PBMCs (A), H9 cells (B), CEM-SS cells stably expressing the NTAP-A3G protein (C), or HeLa cells transfected with pA3G (D) were fixed and probed with rabbit anti-A3G antibodies. The cells were then stained with a rabbit-specific secondary antibody directly conjugated to Alexa Fluor 594 dye. Cell nuclei were stained with DAPI dilactate, and the samples examined by confocal microscopy. Scale bars = 10 μm.
APOBEC3G localizes to P bodies.
Cytoplasmic RNP complexes can cluster or accumulate in discrete microdomains within the cytoplasm. In particular, P bodies and SGs are two types of nonmembraned RNP granules where translationally silenced mRNA is stored and/or degraded in mammalian cells (5, 7, 18, 27, 30, 38, 57, 58, 103, 111). P bodies are sites of accumulation for components of the mRNA-decapping and 5′-to-3′ mRNA decay machinery (30, 50, 71, 103, 117), the nonsense-mediated decay pathway (41, 104, 115), and RNA-induced silencing complexes (RISC) (68, 82, 96, 100), whereas SGs are sites where mRNAs coding for “housekeeping” proteins are transiently stored in a translationally silenced state during cellular stress, thereby facilitating the preferential translation of proteins important for the stress response (4, 6, 57). Although the precise roles of P bodies versus SGs have yet to be fully defined—and they may well overlap to some extent—mRNA localization to P bodies is generally associated with the terminal stages of RNA degradation pathways. Provocatively, Mov10, one of the proteins identified in A3G RNP complexes (Table (Table11 and Fig. Fig.2),2), is an established constituent of P bodies (82, 113), whereas certain other A3G-associated proteins such as PABPC1 and HuR can each accumulate in SGs (42, 59). Taken together with A3G's pronounced localization to cytoplasmic foci, these findings prompted us to investigate the possible targeting of A3G to P bodies and/or SGs.
We first examined A3G localization to P bodies (Fig. (Fig.4).4). Here, proliferating HeLa cell monolayers were cotransfected with expression vectors for tagged versions of A3G and various P-body components that carried either YFP, CFP, red fluorescent protein (RFP), or a myc epitope tag as required. The P-body proteins analyzed included Mov10, a putative RNA helicase (belonging to the dead-like helicase superfamily) that can associate with the Ago1 and Ago2 proteins (82, 113), both of which also accumulate in P bodies and are components of the RISC complex (39, 67, 81, 106); Ago1 and Ago2 themselves; the dead box helicase rck/p54, which facilitates P-body formation and acts as a translational repressor (26, 27); Dcp1a, the mRNA-decapping enzyme involved in the 5′-to-3′ degradation of mRNA (30, 71); and GW182, a protein that has been ascribed roles in RNA silencing and P-body integrity (53, 68). After 24 to 36 h, the cells were fixed, stained as necessary, and examined using confocal microscopy. Figure 4A to F show representative fields for each sample, with the single-channel analyses and merged images displayed. The results were essentially indistinguishable among these six samples, with A3G and all P-body markers displaying diffuse cytosolic staining and prominent localization to cytoplasmic microdomains. Most importantly, the majority of the A3G foci colocalized with P bodies, indicating that A3G can indeed accumulate in these RNP-rich regions of the cytoplasm. It was also clear, however, that not all areas of intense A3G accumulation coincided with specific P-body proteins (this is particularly obvious in the merged panel E), suggesting either that P bodies may be heterogeneous in their composition or that A3G can also localize to other cytoplasmic microdomains.
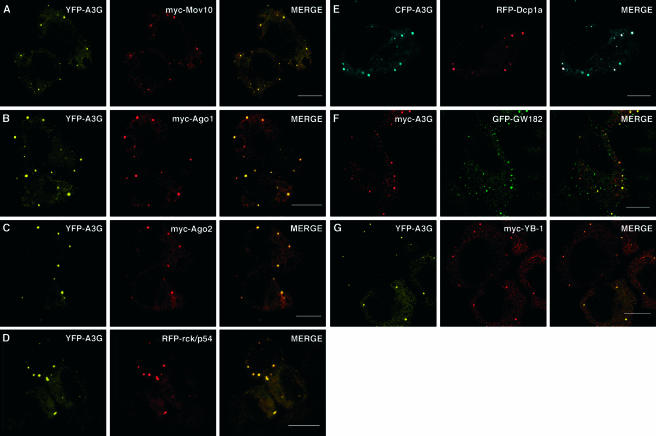
A3G colocalizes with P bodies in the cytoplasm. HeLa cells coexpressing YFP-A3G, CFP-A3G, or myc-A3G (left panels) and the indicated P-body markers myc-Mov10 (A), myc-Ago1 (B), myc-Ago2 (C), RFP-rck/p54 (D), mRFP-Dcp1a (E), GFP-GW182 (F), and myc-YB-1 (G) (middle panels) were fixed and stained with the 9E10 antibody and a mouse-specific secondary antibody conjugated to Alexa Fluor 594 dye. Samples were viewed by confocal microscopy, and merged views are displayed on the right. Scale bars = 10 μm.
We also examined the possible colocalization of A3G with a number of the other proteins identified in NTAP-A3G complexes (Table (Table1).1). Again, unstressed cultures of HeLa cells were cotransfected with combinations of tagged expression vectors and subcellular localization was assessed using double-label confocal microscopy. To summarize a large number of experiments, we did not observe significant colocalization between A3G and LRP130, hnRNP U, La, Ro60, SRP68, PABPC1, or HuR (data not shown; also refer to Fig. Fig.5E).5E). Indeed, in some cases, such as those of hnRNP U and La, the test protein localized to the nucleus, consistent with published results (51, 77). Whether the capacity of these proteins to copurify with A3G-containing RNPs reflects artifactual associations taking place during the purification procedure or the physiologic binding of small fractions of protein naturally present in the cytoplasm remains to be determined. The exception in these findings was YB-1, which displayed a cytoplasmic staining but also clearly colocalized with A3G in cytoplasmic microdomains (Fig. (Fig.4G);4G); the accumulation of YB-1 in P bodies was confirmed in costaining experiments with Dcp1a (data not shown). A number of activities have been ascribed to YB-1, including its presence in translationally inactive RNP complexes, where it competes for initiation factors (35-37, 90). Given the current understanding of P-body function(s), it is perhaps not surprising that YB-1 can accumulate in them.
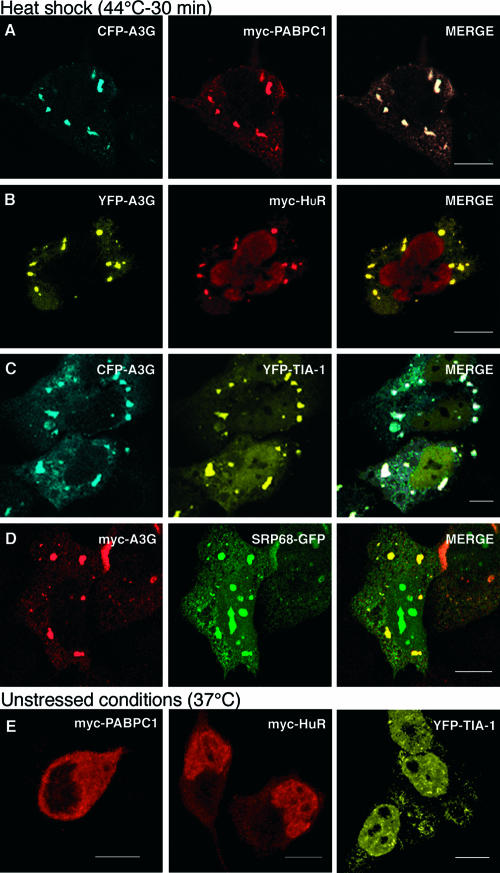
A3G localizes to stress granules after heat shock. HeLa cells coexpressing CFP-A3G, YFP-A3G, or myc-A3G (left panels) and the indicated SG markers myc-PABPC1 (A), myc-HuR (B), YFP-TIA-1 (C), or SRP68-GFP (D) were incubated at 44°C for 30 min to induce SG formation. (E) Patterns of localization of myc-PABPC1, myc-HuR, and YFP-TIA-1 under normal cell growth conditions. Fixed cells were stained with the 9E10 antibody (A, B, D, and the first two panels of E), processed as for Fig. Fig.44 and viewed by confocal microscopy. Merged views are displayed to the right. Scale bars = 10 μm.
Localization of APOBEC3G to stress granules.
As discussed above, SGs are another category of RNP-rich cytoplasmic microdomains involved in cellular mRNA metabolism. They can assemble in response to cellular insult (59), contain many RNA-binding proteins (for a review, see reference 4), and possibly serve as a storage compartment for mRNAs whose translation is concomitantly suppressed (5). Because some components of A3G RNP complexes, such as PABPC1 and HuR, are known to accumulate in SGs under stress conditions (42, 59), we next asked whether A3G can also localize to SGs. To address this, we used the same double-label microscopy strategy described above, except that the transfected HeLa cells were subjected to heat shock at 44°C for 30 min prior to fixation and examination (Fig. (Fig.5).5). Previous work had indicated that this protocol induces the formation of SGs but not P bodies (58).
As seen in the panels displaying A3G staining alone, heat shock triggered dramatic changes in A3G localization such that much of the protein now accumulated in large cytoplasmic granules of irregular morphology. As expected, these areas of intense staining coincided with the localization of PABPC1 (Fig. (Fig.5A),5A), HuR (Fig. (Fig.5B),5B), and a previously defined SG marker, TIA-1 (Fig. (Fig.5C).5C). As discussed above, the striking colocalization of A3G with PABPC1 or HuR could not be observed in the absence of cellular stress (refer to Fig. Fig.5E5E for illustrations of PABPC1, HuR, and TIA-1 localization before heat shock), even though biochemical studies had indicated their presence in the same RNP complexes (Table (Table11 and Fig. Fig.2).2). Accordingly, we suspect that these complexes may contribute to the diffuse cytosolic A3G staining that is seen under unstressed conditions (Fig. (Fig.33 and and4)4) and that likely represents association with polysomes.
We also tested a number of other proteins found in A3G RNP complexes for their ability to colocalize with A3G in SGs following heat shock. Most proteins did not display this phenotype (data not shown); the exception was SRP68 (Fig. (Fig.5D).5D). It is unclear whether other components of the signal recognition particle and the 7SL RNA are also targeted to SGs, and further study will be required to address this question.
Redistribution of APOBEC3G and P-body components to stress granules.
Since A3G can accumulate in P bodies or SGs depending upon the physiologic status of the cell, we next asked whether any other P-body proteins redistribute to SGs under stress conditions. HeLa cells were triply transfected with pCFP-A3G, pEYFP-TIA-1 (to label SGs), and an expression vector for a myc- or mRFP-tagged P-body marker. Cells were later subjected to heat shock and examined by confocal microscopy as described above (Fig. (Fig.6).6). Most P-body proteins that were examined relocalized to SGs together with A3G: results are shown for Mov10 (Fig. (Fig.6A),6A), Ago2 (Fig. (Fig.6B),6B), and YB-1 (Fig. (Fig.6C),6C), but Ago1 and rck/p54 behaved similarly (data not shown; also see reference 125). The exceptions to this pattern were the decapping enzyme Dcp1a, which remained confined to foci of intense staining that were clearly distinct from SGs, and GW182, which remained predominantly associated with P bodies (Fig. (Fig.6D;6D; also data not shown and reference 58). Thus, a subset of P-body proteins continues to colocalize with A3G under changing cellular environments.
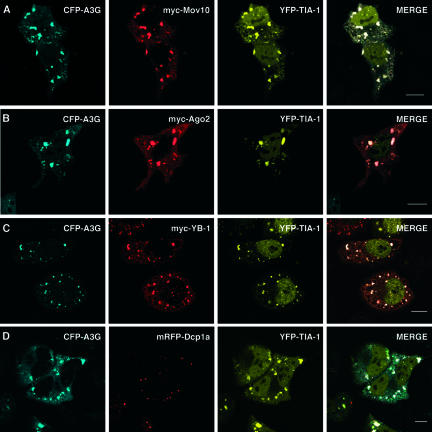
Some P-body proteins colocalize to stress granules with A3G after heat shock. HeLa cells coexpressing CFP-A3G, YFP-TIA-1 (to define SGs), and the P-body markers myc-Mov10 (A), myc-Ago2 (B), myc-YB1 (C), and mRFP-Dcp1a (D) were stressed and analyzed as for Fig. Fig.5.5. Merged views are displayed to the right. Scale bars = 10 μm.
APOBEC3G interacts with P-body proteins.
While the previous experiment revealed that a number of P-body components redistribute to SGs in apparent coordination with A3G under heat shock conditions, only Mov10 and YB-1 were detected in our TAP tag analyses of A3G RNP complexes (Table (Table11 and Fig. Fig.2).2). The reason for this may simply be practical, in that some P-body proteins that are associated with NTAP-A3G complexes were not sequenced (Fig. (Fig.1C).1C). To address this point, we performed a series of additional coimmunoprecipitation experiments to assess interactions between A3G and P-body proteins, as well as their possible dependency upon RNA (Fig. (Fig.7).7). As can be seen in the upper panels of Fig. Fig.7A,7A, Ago1, Ago2, and GW182 are all present in A3G-containing RNP complexes. Like Mov10 and YB-1 (Fig. (Fig.2),2), the interaction with GW182 was lost following treatment with RNase. In contrast, the interactions with the two RISC components Ago1 and Ago2 were partially resistant to digestion with RNase. This was also seen in reciprocal experiments in which the Ago proteins were tagged with a myc epitope and coprecipitating A3G was detected by immunoblotting (Fig. (Fig.7B).7B). While we do not yet appreciate fully the significance of variations in RNase sensitivity, we speculate that A3G's interactions with Ago1 and Ago2 may involve direct protein-protein contacts that are somewhat resilient to the removal of RNA. It is also interesting that Ago1 and Ago2 belong to the subset of P-body proteins that can redistribute to SGs with A3G: it is conceivable that A3G, Ago1, and Ago2 may interact as a more stable complex (or set of complexes) that is able to traffic to different regions or microdomains of the cytoplasm in response to changing environmental cues.
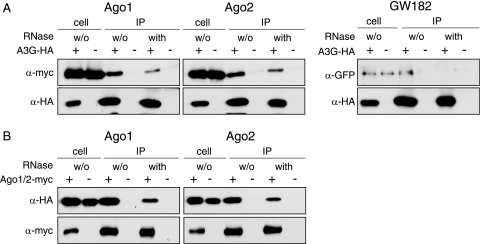
Interactions of A3G with P-body proteins. Transfected 293T cells coexpressing A3G-HA (or empty vector) and tagged versions of P-body markers were lysed, immunoprecipitated for A3G-HA with an anti-HA antibody (A) or for Ago1/Ago2-myc with an anti-myc antibody (B), and subjected to RNase digestion as described for Fig. Fig.2.2. Samples were analyzed by immunoblotting using the indicated primary antibodies. w/o, without.
DISCUSSION
The presence of APOBEC cytidine deaminases in cells means that much of the RNA/DNA content of such cells has the potential to be targeted for editing. However, such sequence-changing events appear to be rare, suggesting that the enzymatic activities of these proteins are tightly regulated and suppressed. For instance, sequence comparisons between human cDNA libraries and the human genome suggest that C-to-U editing of RNA occurs extremely infrequently (65). To begin to address the potential regulatory mechanisms for controlling A3G function, we have used affinity purification to identify proteins that interact with A3G and confocal microscopy to examine subcellular localizations and relative proximities of these proteins in intact cells.
When TAP-tagged A3G was used as the bait, a large number of cellular proteins were found to interact with A3G (Fig. (Fig.1).1). Treatment with RNase resulted in the loss of many of these interactions, indicating that they are predominantly indirect in nature and are detected because multiple proteins, including A3G, simultaneously bind to the same RNA molecules. Indeed, these observations are consistent with the work of Chiu and colleagues, who described A3G- and RNA-containing high-molecular-weight complexes whose formation is reversed by RNase treatment (25). Moreover, these investigators demonstrated that the editing activity of A3G is masked when it is associated with RNP complexes, suggesting that recruitment to such complexes can regulate A3G function.
Mass spectrometry was used to determine the identity of a subset of the proteins in A3G RNP complexes (Table (Table1),1), and RNA-dependent interactions with A3G were confirmed in a series of coimmunoprecipitation experiments (Fig. (Fig.2).2). A clear trend was evident in that the majority of the interacting proteins are known RNA-interacting, and particularly mRNA-interacting, proteins; for example, PABPC1, HuR, YB-1, and the hnRNP proteins are all well-established mRNA-binding proteins that have been ascribed many diverse roles in mRNA biogenesis and metabolism. In addition, other proteins, such as Ro60 and La, are known to interact with some RNA polymerase III transcripts (127, 128). We also note that a subset of the identified proteins (PABPC1, RNA helicase A, and hnRNP U) have previously been implicated in various steps in the HIV-1 life cycle (1, 47, 116); whether these proteins can influence the antiviral activities of A3G remains to be addressed.
Indirect immunofluorescence analyses of endogenous A3G as well as ectopically expressed protein revealed pronounced accumulation in distinct cytoplasmic foci as well as more-diffuse localization throughout the cytosol (Fig. (Fig.3).3). In unstressed cells, a series of double-label experiments defined these A3G-rich puncta as P bodies, known sites of mRNA translational repression, storage, and degradation (Fig. (Fig.4).4). Since SGs are morphologically distinct sites of RNA metabolism that are spatially and compositionally linked to P bodies, we also assessed A3G localization to SGs. When heat shock was used to promote SG formation, A3G was shown to redistribute rapidly to these sites of mRNA storage together with other proteins, including some that are found in P bodies (Fig. (Fig.55 and and6).6). For the proteins that are not in P bodies (e.g., PABC1 and HuR), the origin of the proteins destined for retargeting to SGs is presumably the cytosol, where the diffuse staining patterns seen under unstressed conditions (Fig. (Fig.44 and data not shown) likely reflect association with polysomal RNA (discussed further below).
Further coimmunoprecipitation studies with a number of P-body markers then revealed that among those proteins that coordinately redistribute to SGs with A3G, the Ago1, Ago2, and GW182 proteins can also interact with A3G (Fig. (Fig.7).7). However, in contrast to all the other proteins we have examined to date, Ago1 and Ago2 do so in a manner that appears partially RNA independent (Fig. (Fig.7).7). Table Table22 portrays a summary of some of the characteristics of host proteins that we have described as being in proximity with A3G, together with the assays used to support these conclusions.
TABLE 2.
Summary characterization of proteins associated with A3G RNP complexes
Protein | Co-IP with A3G | Immunolocalization with A3G
| |
---|---|---|---|
in P bodies (37°C) | in stress granules (44°C) | ||
Mov10 | + | + | + |
Ago1 | +a | + | + |
Ago2 | +a | + | + |
GW182 | + | + | − |
YB-1 | + | + | + |
Dcp1-a | − | + | − |
PABPC1 | + | − | + |
HuR | + | − | + |
SRP68 | + | − | + |
TIA-1 | − | − | + |
La | + | − | − |
Ro | + | − | − |
hnRNP U | −b | − | − |
LRP130 | −b | − | − |
During the course of this work, two other groups reported findings that are in good general agreement with our own. First, Wichroski et al. demonstrated that A3G (as well as A3F) localizes to P bodies and can interact with certain constituent proteins such as Ago2, rck/p54, and the translation initiation factor eIF4E (123). In contrast to our experiments, all interactions with A3G were described as RNase sensitive, and further work will be required to address this minor discrepancy. Second, Kozak et al. conducted an analogous TAP tag-based screen for A3G-interacting proteins (62). Importantly, many of the same cellular proteins were identified, thus helping to validate our results, though a number of ribosomal proteins were also found. As with our work and that of Wichroski et al., many interactions were shown to be sensitive to treatment with RNase. Additional experiments using sedimentation through density gradients indicated that a significant fraction of A3G is associated with polysomes under unstressed conditions (as suggested above), but that it preferentially associates with dormant mRNA-protein complexes in the presence of an inhibitor of translational initiation (puromycin) or stress inducers (arsenite, cyanide). Microscopy analyses confirmed the inducible nature of A3G accumulation in SGs (62). One difference with the work described herein was that A3G targeting to P bodies was not addressed, though interactions with P-body components such as Mov10 were detected.
Taken together, these independent studies reveal that A3G associates with cellular RNP complexes that contain complex mixtures of proteins involved (or implicated) in a wide range of activities that pertain to RNA function and metabolism. For unstressed conditions, we favor a model where A3G is mostly in equilibrium between P bodies and polysomes (the latter being reflected by diffuse cytosolic staining). Following the onset of cellular stress, significant levels of A3G, as well as a number of other components of A3G RNP complexes, redistribute to SGs. We therefore predict that the composition of the A3G-containing RNP complexes varies in response to changing localization, cellular growth conditions, and so forth. Characterizing and understanding such changes are important future directions for this work.
Another central question regarding A3G RNP complexes is the determination of their RNA components. Kozak et al. have begun to address this point and have found that HIV-1 RNA and A3G mRNA, but not actin mRNA, are detectable in A3G complexes (62). The presence of HIV-1 RNA is perhaps not so surprising, since RNA plays an important role (along with the nucleocapsid portion of Gag) in the packaging of A3G into assembling virus particles (2, 20, 60, 70, 98, 132). It is worth noting, however, that A3G RNP complexes are present in cells in the absence of HIV-1 infection, demonstrating that viral RNA is not required for the formation of A3G-containing RNP complexes. One category of RNAs that is likely to be present in a subset of A3G complexes includes the transcripts of various retrotransposons. As summarized earlier, the mobility of many of these can be restricted by A3G and it is possible that RNP complexes participate in the inhibitory process(es). However, based on the identity of proteins present in A3G RNP complexes (Table (Table1)1) (62), a prediction is that these RNP complexes contain additional mRNAs and noncoding RNAs. Accordingly, defining these RNAs will provide insight into the RNA substrates whose function and fate may be regulated by A3G action. We anticipate that the editing-dependent as well as -independent activities of A3G may participate in this regulation, though one might predict the latter activities to feature prominently, since A3G's incorporation into RNPs is known to mask cytidine deaminase activity (25).
An intriguing series of questions that emerges from these results and those of others concerns the possible role(s) of P bodies in the life cycles of retroviruses and their intracellular relatives, retrotransposons. It is plausible that P bodies could contribute positively or negatively. For instance, the integrity of P bodies as well as certain P-body components are important for the transposition of the yeast retrotransposon Ty3 (11, 52), thus posing the question regarding a possible role for P bodies in promoting retroviral replication, assembly, or production (110). On the other hand, the inhibition of RNA silencing and RISC assembly through the removal of Dicer from cells is associated with retrotransposon mobilization in mice (109), and the inhibition of miR-32 function (a cellular micro-RNA) promotes the replication of the retrovirus primate foamy virus type-1 (63). Since RISC is localized to P bodies, these findings might suggest that P bodies participate in suppressing retroviral infection and transposition. Moreover, as A3G associates with the RISC components Ago1 and Ago2 (Fig. (Fig.7),7), it is further possible that pathways involved in RNA silencing may be recruited to invading RNA by A3G as a means of controlling retroviral infection and/or transposition. Evidently, much work will be required to test these ideas and to elucidate the regulation of the function of A3G in the context of its contributions to cell function and integrity.
While this work was under review, Chiu and colleagues (25a) also reported a proteomic analysis of A3G-interacting factors. Consistent with our results and those of Kozak et al. (62), many cellular RNA-binding proteins were reported to interact with A3G, mostly in an RNase-sensitive manner. These investigators also found that A3G RNPs are enriched for Alu and hY RNAs, two classes of endogenous retroelement, and that A3G inhibits Alu element retrotransposition. These results support the model that the sequestration of retroelement RNAs in A3G RNPs is important for limiting transposition.
Acknowledgments
This work was supported by the United Kingdom Medical Research Council. S.G.-M. is a Fellow of the European Molecular Biology Organization, and M.H.M. is an Elizabeth Glaser Scientist supported by the Elizabeth Glaser Pediatric AIDS Foundation.
We thank Monica Agromayor, Paul Anderson, Edward Chan, Greg Hannon, Vicky Katsanou, Nancy Kedersha, Megerditch Kiledjian, Dimitris Kontoyiannis, Tim Ley, Jidong Liu, Zhi Hong Lu, Jens Lykke-Andersen, Juan Martin-Serrano, Gunter Meister, Thoru Pederson, Serafín Piñol-Roma, Ger Pruijn, Thomas Tuschl, and Dominique Weil for reagents and invaluable advice.
REFERENCES
Articles from Journal of Virology are provided here courtesy of American Society for Microbiology (ASM)
Full text links
Read article at publisher's site: https://doi.org/10.1128/jvi.02287-06
Read article for free, from open access legal sources, via Unpaywall:
https://europepmc.org/articles/pmc1865933?pdf=render
Citations & impact
Impact metrics
Citations of article over time
Alternative metrics
Smart citations by scite.ai
Explore citation contexts and check if this article has been
supported or disputed.
https://scite.ai/reports/10.1128/jvi.02287-06
Article citations
Ro60-Roles in RNA Processing, Inflammation, and Rheumatic Autoimmune Diseases.
Int J Mol Sci, 25(14):7705, 14 Jul 2024
Cited by: 0 articles | PMID: 39062948 | PMCID: PMC11277228
Review Free full text in Europe PMC
Potential Role of APOBEC3 Family Proteins in SARS-CoV-2 Replication.
Viruses, 16(7):1141, 16 Jul 2024
Cited by: 0 articles | PMID: 39066304 | PMCID: PMC11281575
Protein Interaction Map of APOBEC3 Enzyme Family Reveals Deamination-Independent Role in Cellular Function.
Mol Cell Proteomics, 23(5):100755, 27 Mar 2024
Cited by: 1 article | PMID: 38548018 | PMCID: PMC11070599
Antiviral Defence Mechanisms during Early Mammalian Development.
Viruses, 16(2):173, 24 Jan 2024
Cited by: 1 article | PMID: 38399949 | PMCID: PMC10891733
Review Free full text in Europe PMC
APOBEC3G Is a p53-Dependent Restriction Factor in Respiratory Syncytial Virus Infection of Human Cells Included in the p53/Immune Axis.
Int J Mol Sci, 24(23):16793, 27 Nov 2023
Cited by: 0 articles | PMID: 38069117 | PMCID: PMC10706465
Go to all (195) article citations
Other citations
Data
Data behind the article
This data has been text mined from the article, or deposited into data resources.
BioStudies: supplemental material and supporting data
Genes & Proteins (Showing 22 of 22)
- (3 citations) UniProt - Q9HC16
- (2 citations) UniProt - P67809
- (2 citations) UniProt - P11940
- (2 citations) UniProt - P10155
- (2 citations) UniProt - Q00839
- (1 citation) UniProt - P07437
- (1 citation) UniProt - P07910
- (1 citation) UniProt - P51991
- (1 citation) UniProt - P68363
- (1 citation) UniProt - P36542
- (1 citation) UniProt - Q15717
- (1 citation) UniProt - O60506
- (1 citation) UniProt - Q9HCC0
- (1 citation) UniProt - Q08211
- (1 citation) UniProt - Q9UHB9
- (1 citation) UniProt - Q9HCE1
- (1 citation) UniProt - P11142
- (1 citation) UniProt - P08670
- (1 citation) UniProt - P82650
- (1 citation) UniProt - P09012
- (1 citation) UniProt - P05166
- (1 citation) UniProt - P05455
Show less
Similar Articles
To arrive at the top five similar articles we use a word-weighted algorithm to compare words from the Title and Abstract of each citation.
High-molecular-mass APOBEC3G complexes restrict Alu retrotransposition.
Proc Natl Acad Sci U S A, 103(42):15588-15593, 09 Oct 2006
Cited by: 180 articles | PMID: 17030807 | PMCID: PMC1592537
The anti-HIV-1 editing enzyme APOBEC3G binds HIV-1 RNA and messenger RNAs that shuttle between polysomes and stress granules.
J Biol Chem, 281(39):29105-29119, 03 Aug 2006
Cited by: 132 articles | PMID: 16887808
Comparison of cellular ribonucleoprotein complexes associated with the APOBEC3F and APOBEC3G antiviral proteins.
J Virol, 82(11):5636-5642, 26 Mar 2008
Cited by: 68 articles | PMID: 18367521 | PMCID: PMC2395208
Regulation of Antiviral Innate Immunity Through APOBEC Ribonucleoprotein Complexes.
Subcell Biochem, 93:193-219, 01 Jan 2019
Cited by: 6 articles | PMID: 31939152
Review
Funding
Funders who supported this work.
Medical Research Council (1)
HIV-Host Interactions
Professor Michael Malim, King's College London
Grant ID: G0401570