Abstract
Free full text

D-β-Hydroxybutyrate rescues mitochondrial respiration and mitigates features of Parkinson disease
Abstract
Parkinson disease (PD) is a neurodegenerative disorder characterized by a loss of the nigrostriatal dopaminergic neurons accompanied by a deficit in mitochondrial respiration. 1-Methyl-4-phenyl-1,2,3,6-tetrahydropyridine (MPTP) is a neurotoxin that causes dopaminergic neurodegeneration and a mitochondrial deficit reminiscent of PD. Here we show that the infusion of the ketone body D-β-hydroxybutyrate (DβHB) in mice confers partial protection against dopaminergic neurodegeneration and motor deficits induced by MPTP. These effects appear to be mediated by a complex II–dependent mechanism that leads to improved mitochondrial respiration and ATP production. Because of the safety record of ketone bodies in the treatment of epilepsy and their ability to penetrate the blood-brain barrier, DβHB may be a novel neuroprotective therapy for PD.
Introduction
Parkinson disease (PD) is the second most common neurodegenerative disease after Alzheimer disease (1). PD is clinically characterized by disabling motor abnormalities, which include tremor, muscle stiffness, paucity of voluntary movements, and postural instability (2), and its main neuropathological feature is the loss of substantia nigra pars compacta (SNpc) dopaminergic neurons (3).
While PD is a sporadic condition of uncertain etiology (2), several lines of evidence suggest that a defect in oxidative phosphorylation contributes to its pathogenesis. For instance, 1-methyl-4-phenyl-1,2,3,6-tetrahydropyridine (MPTP), a neurotoxin that blocks complex I (NADH-ubiquinone oxidoreductase) of the mitochondrial electron transport chain (4), recapitulates in humans the hallmarks of PD (5). Furthermore, reduction in complex I activity has been reported in PD tissues (reviewed in ref. 6). This defect is not confined only to the brain (7), since low complex I activity has also been found in platelets from PD patients (8, 9) and in cybrid cells engineered to contain mitochondria derived from platelets of patients suffering from PD (10).
D-β-Hydroxybutyrate (DβHB) is a ketone body produced by hepatocytes and, to a lesser extent, by astrocytes (11). It is an alternative source of energy in the brain when glucose supply is depleted such as during starvation (12). In vitro DβHB prevents neuronal damage seen following glucose deprivation (13) and mitochondrial poison exposure (14). Herein, we show that DβHB infusion protects SNpc dopaminergic neurons against MPTP in a dose-dependent and stereospecific manner and prevents the development of PD-like motor abnormalities in mice. We also provide in vivo and in vitro evidence that DβHB protects not by alleviating MPTP-related complex I inhibition, but by enhancing oxidative phosphorylation via a mechanism dependent on mitochondrial complex II (succinate-ubiquinone oxidoreductase).
Methods
Animals and treatment.
All animals were 8- to 10-week-old male C57BL mice (Charles River Laboratories, Wilmington, Massachusetts, USA). Mice were divided into four groups: vehicle (i.e., saline), DβHB, L-hydroxybutyrate (LβHB), and DβHB plus 3-nitropropionic acid (3-NP). Vehicle, DβHB (1.6, 0.8, or 0.4 mmol/kg/d in saline, pH 7.4; Sigma-Aldrich, St. Louis, Missouri, USA), and LβHB (1.6 mmol/kg/d in saline, pH 7.4; Sigma-Aldrich) were administered subcutaneously (1 μl/h) using Alzet mini-osmotic pumps (DURECT Corp., Cupertino, California, USA). 3-NP (Sigma-Aldrich; 15 mg/kg, in 0.1 M PBS adjusted to pH 7.4) was given intraperitoneally 2 hours before the implantation on the first day and then once a day until the animals were sacrificed. This dosage of 3-NP was selected to inhibit complex II but not to induce cell loss. After surgery, animals were allowed to rest for 1 day. Each mouse was then randomly assigned to receive four intraperitoneal injections of either MPTP (18 mg/kg of free base in saline; Sigma-Aldrich) or saline at 2-hour intervals.
Tyrosine hydroxylase immunostaining and quantitative morphology.
Seven days after the last MPTP injection, mice were killed and their brains were processed for immunohistochemical studies. Sections (30 μm) were incubated with a polyclonal anti-tyrosine hydroxylase (TH; 1,000 dilution; Calbiochem-Novabiochem Corp., San Diego, California, USA) for 48 hours at 4°C. Biotinylated secondary antibodies followed by avidin-biotin complex were used. Immunoreactivity was visualized by incubation in 3,3′-diaminobenzidine/glucose/glucose oxidase. Total numbers of TH-positive neurons in SNpc were counted stereologically using the optical fractionator method (15). Striatal OD of TH immunostaining, determined by the Scion Image program (Scion Corp., Frederick, Maryland, USA), was used as an index of striatal density of TH innervation (16). The concentration of anti-TH antibody and 3,3′-diaminobenzidine (DAB) and the duration of incubation of striatal sections in DAB were optimized to fall within the linear range of the plot of the immunostaining intensities and the scanned ODs.
Measurement of DβHB and succinate levels.
At different time points after the implantation of the osmotic pumps, blood was collected from tails, and brains were quickly removed, freeze-clamped under liquid nitrogen, and stored at –80°C. Frozen tissues were treated with perchloric acid and neutralized with sodium hydroxide as previously described (17). Both DβHB and succinate were measured spectrophotometrically at 340 nm using commercial kits from Sigma-Aldrich and from Roche Molecular Biochemicals (Indianapolis,Indiana, USA) respectively, following the manufacturers’ instructions.
Measurement of striatal 1-methyl-4-phenylpyridinium levels.
Mice infused with either saline or DβHB (1.6 mmol/kg/d) were injected with MPTP (18 mg/kg) as described above and killed 90 minutes after the fourth injection. HPLC with UV detection (295 nm) was used to measure striatal 1-methyl-4-phenylpyridinium (MPP+) levels as previously described (18) with the following modifications: a reverse-phase Altima C18 column (Alltech Associates Inc., Deerfield, Illinois, USA) and a mobile phase consisting of 89% 50 mM KH2PO4 and 11% acetonitrile were used. Data represent mean ± SEM of five mice per group.
Synaptosomal uptake of MPP+.
Striata were dissected out from naive mice and processed for uptake experiments as described previously (19) with a few modifications. Briefly, striata were homogenized in 0.32 M sucrose and centrifuged at 700 g, 4°C, for 10 minutes. The supernatant was removed and centrifuged at 27,000 g for 30 minutes. The resulting synaptosomal pellet was suspended at 1.2 mg/ml (original wet weight) in Krebs-Ringer phosphate buffer (pH 7.4). The uptake reaction was initiated by addition of 0.6 mg of synaptosomes to tubes containing [3H]MPP+ (~4 nM, ~800,000 degradations per minute, specific activity 31.6 Ci/mmol; American Radiolabeled Chemicals Inc., St. Louis, Missouri, USA) in the absence or presence of DβHB (up to 5 mM) at 37°C for 6 minutes. Nonspecific uptake was assessed in the presence of 10 μM mazindol. Data represent mean ± SEM of three mice per group.
Isolation of brain mitochondria.
Brains from C57BL mice were homogenized in isolation buffer (225 mM mannitol, 75 mM sucrose, 1 mM EGTA, 5 mM HEPES, and 2 mg/ml fat-free BSA) using a motorized Dounce homogenizer with eight up-and-down strokes. The homogenate was centrifuged at 1,000 g for 10 minutes, and the resulting supernatant was layered onto 5 ml of 7.5% Ficoll medium on top of 5 ml of 10% Ficoll medium and centrifuged at 79,000 g for 30 minutes (the Ficoll medium contained 0.3 M sucrose, 50 μM EGTA, and 10 mM HEPES). The mitochondrial pellet was resuspended in isolation buffer. Protein concentrations were determined by the bicinchoninic assay (Pierce Chemical Co., Rockford, Illinois, USA) method with BSA as a standard protein.
Mitochondrial accumulation of MPP+.
Brain mitochondria were isolated and resuspended in buffer as described previously (20) but with a few modifications. The uptake reaction was initiated by addition of 0.6 mg of mitochondria to tubes containing 5 μM [3H]MPP+ and 45 μM MPP+ in the absence or presence of DβHB (up to 5 mM) at 25°C for 3 minutes. Nonspecific uptake was assessed in the presence of 5 μM carbonylcyanide p-trifluoromethoxyphenylhydrazone (FCCP). Data represent mean ± SEM of four or five mice per group.
Polarography.
Brain mitochondria were suspended in respiration buffer consisting of 225 mM mannitol, 75 mM sucrose, 10 mM KCl, 5 mM HEPES, 5 mM K2HPO4, and freshly added 1 mg/ml defatted BSA at 30°C, and oxygen-consumption rates were measured in a closed-chamber cuvette with a mini–stirring bar using a Clark-type electrode (Hansatech Instruments Ltd., Norfolk, United Kingdom). For each reading, 300 μg protein was used in a final 1-ml respiration buffer, and all mitochondria preparations had an average respiratory control ratio of at least 5 when 10 mM glutamate and 5 mM malate were used as NADH-linked substrates.
ATP measurements.
Samples were prepared under conditions identical to those of polarographical study. Mitochondria suspended in respiration buffer were incubated in the presence or absence of different substrates or inhibitors using the same incubation times as those of polarographical study. Where 3-NP was used, it was added from the beginning with MPP+ (5 minutes) or rotenone (2.5 minutes) to mitochondria before the addition of DβHB. When the reaction was stopped, mitochondrial suspension from the cuvette was lysed in an equal volume of lysis buffer from the ATP bioluminescence assay kit (Roche Molecular Biochemicals), and the content of ATP was measured according to the manufacturer’s instructions. Light emitted from luciferase-mediated reaction was captured in a tube luminometer and calculated from a log-log plot of the standard curve of known ATP concentrations.
Measurements of mitochondrial H2O2 production.
Samples were prepared under conditions identical to those of polarographical study. Mitochondria suspended in respiration buffer were incubated in the presence or absence of different substrates or inhibitors using the same incubation times as those of polarographical study. Phenazine methosulfate (0.1 mM) was used to oxidize NADH (21). Hydrogen peroxide, converted from superoxide by manganese-superoxide dismutase, was measured using 5 μM Amplex red (Molecular Probes, Eugene, Oregon, USA) and 5 U/ml HRP. Fluorescence was detected by a Perkin-Elmer (Boston, Massachusetts, USA) LS55 spectrofluorometer with an excitation wavelength of 550 nm (slit 1.5 nm) and an emission wavelength of 585 nm (slit 3 nm). H2O2 production was calculated from a standard curve generated from known concentrations of H2O2.
Measurements of mitochondrial transmembrane potential.
Safranine, a cationic fluorescence dye accumulated and quenched inside energized mitochondria (22, 23) was used to measure transmembrane potential (Δψm). Mitochondria were incubated with 10 mM glutamate, 5 mM malate, and 5 μM safranine (Sigma-Aldrich) in respiration buffer 5 minutes before 5 mM DβHB was added, and Δψm was monitored for an additional 5 minutes. FCCP (5 μM) was used as a positive control to collapse Δψm. Fluorescence was detected by a Perkin-Elmer LS55 spectrofluorometer with an excitation wavelength of 495 nm (slit 3 nm) and an emission wavelength of 586 nm (slit 5 nm). Data are reported in arbitrary fluorescence units (AFUs).
Complex I activity.
Largely based on protocols described by Birch-Machin and Turnbull (24), brain mitochondria were lysed by freeze-thawing in hypotonic buffer (25 mM KH2PO4 [pH 7.2], 5 mM MgCl2) three times. To initiate the reaction, 50 μg mitochondria were added to the assay buffer (hypotonic buffer containing 65 μM ubiquinone1, 130 μM NADH, 2 μg/ml antimycin A, and 2.5 mg/ml defatted BSA) in the absence or presence of different concentrations of rotenone (2.5–15 μM) or MPP+ (10–30 mM). The oxidation of NADH by complex I was monitored at 340 nm spectrophotometrically for 3 minutes at 30°C prior to the addition of rotenone (2 μg/ml), after which the activity was measured for an additional 3 minutes. The difference in rate before and after the addition of rotenone (2 μg/ml) was used to calculate complex I activity.
Complex II histochemistry.
Animals were injected intraperitoneally with either saline or 3-NP (15 mg/kg) once daily for 8 days, the same regimen used in the animals that received DβHB. As described previously (25), animals were perfused with PBS containing 10% glycerol. Brains were rapidly removed, frozen in dry ice–cooled isopentane, and stored at –80°C. Brains were sectioned at 20 μm throughout the entire nigra and striatum. Sections were mounted onto glass microscope slides, and complex II activity was revealed by incubation of sections at 37°C for 20 minutes in 50 mM phosphate buffer (pH 7.6) containing 50 mM succinate as a substrate and 0.3 mM Nitroblue tetrazolium (NBT) as an electron acceptor.
Immunoblots.
Total tissue proteins from ventral midbrains of MPTP- and saline-treated mice were isolated as described previously (26), and 20 μg proteins were separated on 12% SDS-PAGE. Membranes were blotted with polyclonal anti–β-hydroxybutyrate dehydrogenase (1:100; a generous gift from Andrew Marks, Columbia University, New York, New York, USA) and monoclonal anti–β-actin (1:5,000) overnight at 4°C. Secondary antibodies conjugated with HRP were used. Bands of interest were analyzed and quantified using FluorChem 8800 (Alpha Innotech Corp., San Leandro, California, USA).
Rotarod performance.
The Economex system (Columbus Instruments, Columbus, Ohio, USA), consisting of four rotating rods of 3 cm diameter in separated compartments, enables four mice to be recorded simultaneously. Seven days after MPTP or saline injections, implanted pumps containing 1.6 mmol/kg/d DβHB were removed, and mice (4–13 animals per group) were allowed to recover from surgery and dehydration for an additional 7 days. On the testing day, animals were first pretrained three times (1 hour apart) using an accelerating mode. After these training sessions, the time on the rod, with a maximum recording time of 240 seconds, was recorded for successive rotational speeds (15, 18, 21, 24, 27, 30, 32, 36, and 40 rpm), and the overall rod performance (ORP) for each mouse was calculated by the trapezoidal method as the area under the curve in the plot of time on the rod versus rotation speed (27). To assess the responsiveness of the MPTP-related motor deficit to dopaminergic stimulation, mice were injected intraperitoneally with L-3,4-dihydroxyphenylalanine (L-DOPA) methyl ester/benserazide (100/25 mg/kg), and Rotarod performance was assessed 45 minutes later.
Measurement of dopamine and its metabolite levels in striatal and ventral midbrain tissues.
Animals from the Rotarod study were sacrificed, and their striata and ventral midbrains were dissected out and stored at –80°C until analysis. On the day of the assay, striatal and ventral midbrain tissues were sonicated in 50 and 10 volumes (wt/vol), respectively, of 0.1 M perchloric acid containing 50 ng/ml dihydrobenzylamine as internal standard. After centrifugation at 15,000 g for 15 minutes at 4°C, 20 μl of supernatant was injected onto a C18 reverse-phase HR-80 catecholamine column (ESA Inc., Bedford, Massachusetts, USA). The mobile phase consisted of 94% 50 mM sodium phosphate/0.2 mM EDTA/1.2 mM heptanesulfonic acid (pH 3.2) solution and 6% methanol. The flow rate was 1.5 ml/min. Peaks were detected by an ESA 8 Channel CoulArray system. Data were collected and processed using the CoulArray data analysis program (version 1.12).
Statistical analysis.
All values are expressed as mean ± SEM. Differences between means were analyzed using one-way ANOVA followed by Newman-Keuls post-hoc testing for pairwise comparison. The null hypothesis was rejected when P was greater than 0.05.
Results
MPTP upregulates DβHB-metabolizing enzyme and increases utilization of DβHB in the brain.
To assure sustained high tissue levels of DβHB during the experiment, this short-half-life (28) compound was infused subcutaneously at a dose of 1.6 mmol/kg/d for the entire 7 days. This regimen seemed well tolerated and yielded a stable plasma level of approximately 0.9 mM throughout the 7-day period. Likewise, brain DβHB levels in mice intoxicated with MPTP did not significantly change throughout the experiment (Figure (Figure1a).1a). Brain DβHB levels in mice that received saline instead of receiving MPTP, 3-NP, or both were significantly higher, at least at the beginning of the experiment (Figure (Figure11a).
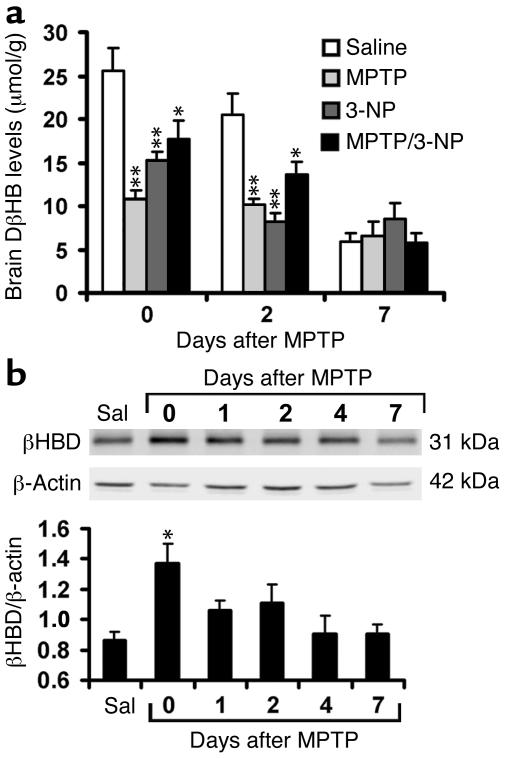
Brain levels of DβHB and β-hydroxybutyrate dehydrogenase (βHBD) under different treatments. (a) One day after implantation of pumps containing DβHB, animals were injected intraperitoneally with saline (Sal), MPTP, or 3-NP as described in Methods, and brain levels of DβHB were measured at 0 days (90 minutes after the fourth injection), 2 days, and 7 days thereafter. The utilization of DβHB was increased when cells were under metabolic stress induced by these toxins. n = 4–6; *P < 0.05 and **P < 0.01 compared with the respective control saline groups. (b) Western blot analysis of ventral midbrains from MPTP-intoxicated mice shows upregulation of this enzyme as early as day 0. n = 4–5 per group; *P < 0.05 compared with the control saline group. β-Actin is used to normalize βHBD values.
Circulating DβHB readily crosses the blood-brain barrier and enters mitochondria, where it is metabolized by β-hydroxybutyrate dehydrogenase to acetoacetate; the latter is converted to acetyl-CoA, which feeds into the Krebs cycle (29). In saline-injected control mice, β-hydroxybutyrate dehydrogenase protein content in ventral midbrain (the brain region that contains the SNpc) was detectable (Figure (Figure1b).1b). In MPTP-injected mice, β-hydroxybutyrate dehydrogenase protein content in ventral midbrain rose rapidly and remained elevated for 2 days after the last injection of MPTP (Figure (Figure1b).1b). These data suggest that MPTP-related cellular stress is associated with a β-hydroxybutyrate dehydrogenase upregulation, which in turn may facilitate utilization of DβHB in the brain.
DβHB attenuates MPTP-induced dopaminergic neurodegeneration.
One day after implantation of pumps containing either vehicle or DβHB, mice were injected with MPTP. Seven days later, the brains of these animals were processed for quantification of dopaminergic cell bodies in the SNpc and of projecting dopaminergic fibers in the striatum using TH immunostaining. In saline-injected mice infused with either vehicle or DβHB, numbers of TH-positive neurons in the SNpc were identical (Table (Table1;1; Figure Figure2,2, a and b), as were TH ODs in the striatum (Table (Table1;1; Figure Figure2,2, i and j). In MPTP-injected mice infused with vehicle, there was an approximately 70% loss of SNpc TH-positive neurons and an approximately 90% reduction of striatal TH ODs (Table (Table1;1; Figure Figure2,2, e and m) compared with saline-injected controls (Table (Table1;1; Figure Figure2,2, a and i). In contrast, in MPTP-injected mice infused with DβHB, less reduction in SNpc TH-positive neurons and striatal TH ODs was observed (Table (Table1;1; Figure Figure2,2, f and n). To control for the specificity of DβHB neuroprotection, another set of MPTP-injected mice received infusion of the inactive isomer LβHB. In these mice, the loss of dopaminergic neurons was as severe as in mice infused with vehicle (Table (Table1;1; Figure Figure2,2, g and o). Thus, DβHB, but not its inactive isomer, can attenuate neurotoxic effects of MPTP on dopaminergic cell bodies in the SNpc and nerve fibers in the striatum.
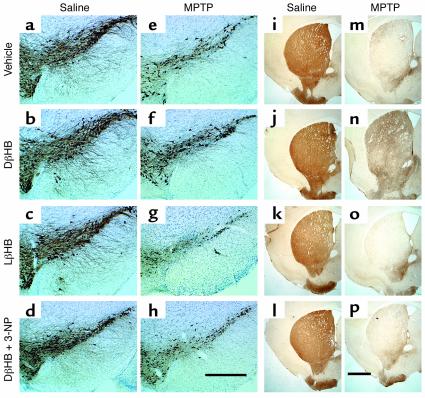
Protective effect of DβHB against MPTP-induced neurodegeneration. (a–h) TH-positive neurons in SNpc, and (i–p) TH-positive terminals in striatum. Animals were infused subcutaneously with vehicle (saline; a, e, i, and m), DβHB (1.6 mmol/kg/d; b, d, f, h, j, l, n, and p), or LβHB (1.6 mmol/kg/d; c, g, k, and o) 1 day before receiving intraperitoneal injections of either saline (a–d and i–l) or MPTP (18 mg/kg; e–h and m–p). There is an extensive loss of TH-positive neurons (e) and terminals (m) in MPTP-injected animals. This loss is attenuated by DβHB (f and n) but not by its inactive isomer LβHB (g and o). The complex II inhibitor 3-NP was given intraperitoneally (15 mg/kg) daily for the entire period of DβHB infusion. In the presence of 3-NP, DβHB does not confer neuroprotection. Scale bars: 500 μm (a–h) and 1 mm (i–p). Please refer to Table Table11 for quantification of neurons and terminals in each animal group.
Table 1
TH- and Nissl-positive neurons in SNpc and striatal TH density
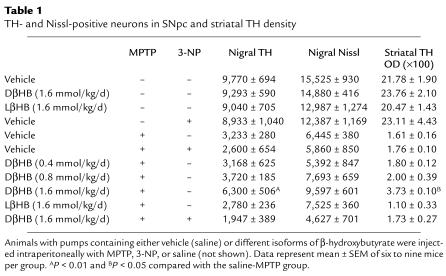
DβHB attenuates the loss of dopamine and the motor deficit induced by MPTP.
To examine whether DβHB protects not only against structural damage but also against functional deficits caused by MPTP, we assessed levels of dopamine and two of its metabolites, dihydroxyphenylacetic acid (DOPAC) and homovanillic acid (HVA), in ventral midbrain and striatum, as well as locomotor activity, in these animals. In MPTP-injected mice that did not receive DβHB, there was a reduction in dopamine and its metabolites (Table (Table2)2) in both ventral midbrain and striatum. Behaviorally, the length of time that these MPTP-injected mice remained on the rotating rods was significantly shorter than that of the saline-injected controls (Figure (Figure3).3). The motor deficit observed in MPTP-treated mice was alleviated by the administration of L-DOPA/benserazide (data not shown), indicating that this motor deficit results from a loss of dopamine. In MPTP-injected mice that did receive DβHB, the levels of dopamine and its metabolites were all significantly higher than those in MPTP-injected mice that did not receive DβHB (Table (Table2).2). Of note, the attenuation of MPTP-induced dopamine loss by DβHB was smaller than the attenuation of MPTP-induced SNpc neuronal death by DβHB. Similarly, MPTP-injected mice that received DβHB performed much better on the rotating rods than MPTP-injected mice that did not receive DβHB (Figure (Figure3).3). Saline-injected mice that received DβHB had similar levels of dopamine and metabolites (Table (Table2)2) and similar motor performance (Figure (Figure3)3) to those of saline-injected mice that did not receive DβHB.
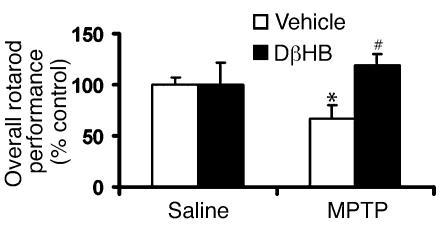
Protective effect of DβHB against motor deficit in MPTP-treated mice. Animals were infused subcutaneously with either vehicle (saline) or DβHB (1.6 mmol/kg/d) 1 day before receiving intraperitoneal injections of either saline or MPTP (18 mg/kg). Pumps were removed at day 7, and animals were allowed to recover from surgery and dehydration for an additional 7 days before their Rotarod performance was assessed. Motor deficit is observed in the MPTP-treated animals, but DβHB significantly improves this impairment. DβHB does not affect base-line motor function in saline-injected mice. n = 4–13; *P < 0.05 compared with the saline-vehicle group; #P < 0.05 compared with the MPTP-vehicle group.
Table 2
Levels of dopamine and its metabolites in ventral midbrain and striatal tissues
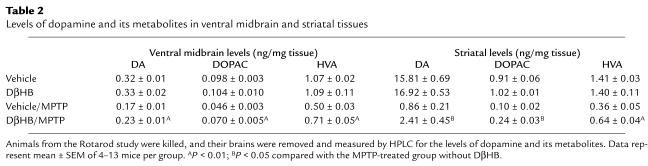
DβHB does not affect MPTP activation.
MPTP is a protoxin whose effect correlates with the striatal content of its active metabolite MPP+ (30). Striatal levels of MPP+ 90 minutes after the last injection of MPTP did not differ between mice that received DβHB (30.9 ± 1.6 μg/g tissue) or vehicle [26.8 ± 1.3 μg/g tissue; Student’s t test with 6 degrees of freedom (t(6)) = 1.98; P = 0.1]. MPTP-induced dopaminergic neurotoxicity relies on the entry of MPP+ into dopaminergic neurons via dopamine transporters (31). DβHB did not impair the uptake of [3H]MPP+ by striatal synaptosomes at concentrations up to 5 mM, which is more than five times the plasma concentration found in DβHB-infused animals (vehicle, 100% ± 2.3% of control; DβHB, 99.1% ± 1.8% of control; t(6) = 0.3; P = 0.8). Inside dopaminergic neurons, MPP+ is concentrated within mitochondria by a mechanism that depends on mitochondrial Δψm (20). At 5 mM, DβHB did not alter the uptake of [3H]MPP+ by purified brain mitochondria (vehicle, 100% ± 4.1% of control; DβHB, 93.2% ± 0.5% of control; t(6) = 1.7; P = 0.1). Thus, it is unlikely that the neuroprotective effect of DβHB in the MPTP model of PD results from alterations in the key MPTP toxicokinetic steps described above.
DβHB increases mitochondrial oxygen consumption.
DβHB has been used as a mitochondrial substrate (32, 33). We thus asked whether DβHB could support oxidative phosphorylation in brain mitochondria, and, if so, whether it may rescue mitochondrial respiration depressed by MPP+-mediated complex I blockade (34). Consistent with DβHB being a mitochondrial substrate, we found that it increased oxygen consumption in a dose-dependent manner (Figure (Figure4,4, a and b). The effects of DβHB in supporting mitochondrial respiration are stereospecific, since the inactive isomer LβHB failed to improve oxidative phosphorylation (Figure (Figure4c).4c). We also found that DβHB ameliorated oxygen consumption impaired by different concentrations of MPP+ (Figure (Figure4a)4a) and of another complex I inhibitor, rotenone (Figure (Figure4b).4b). At 25 μM MPP+ and 25 nM rotenone, which we found to inhibit about 25% of the oxygen consumption in glutamate- and malate-supported mitochondria, DβHB restored completely the oxygen consumption depressed by these inhibitors (Figure (Figure4,4, a and b). At 100 μM MPP+ and 100 nM rotenone inhibits more than 90% of the oxygen consumption in glutamate- and malate-supported mitochondrial respiration (data not shown). At these concentrations, DβHB restored completely the oxygen consumption inhibited by MPP+, but only partially that inhibited by rotenone (Figure (Figure4,4, a and b).
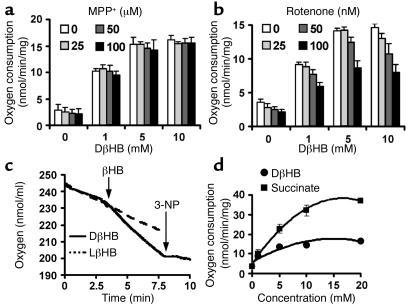
DβHB increases oxygen consumption in purified brain mitochondria. Mitochondria (300 μg) were incubated in the absence or presence of MPP+ (5 minutes; a) or rotenone (2.5 minutes; b) at 30°C, and then 5 mM DβHB was added to induce oxygen consumption. DβHB attenuated inhibition of mitochondrial respiration induced by MPP+ (a) or rotenone (b) at indicated concentrations, which blocked about 25–90% of oxygen consumption when glutamate and malate were used as NADH-linked substrates (data not shown). (c) The improvement of oxygen consumption by DβHB is stereospecific and is blocked by 10 mM 3-NP, a complex II inhibitor. (d) DβHB increases oxygen consumption in a dose-dependent and saturable fashion as seen with succinate, a complex II substrate, although not as efficiently as succinate does on an equimolar basis. n = 3–4.
DβHB does not uncouple mitochondria.
To assure that the increase in rate of oxygen consumption induced by DβHB is not an artifact of uncoupled mitochondria, we measured Δψm. As expected, the uncoupler FCCP at 5 μM collapsed the Δψm in isolated mitochondria (FCCP, 419 ± 23 AFUs; no FCCP, 69 ± 2 AFUs). Conversely, DβHB at concentrations as high as 5 mM had no effect on mitochondrial Δψm (DβHB, 68.58 ± 3.07 AFUs; no DβHB, 65.21 ± 3.03 AFUs; n = 5 per group; P > 0.05). We also found that the increase in oxygen-consumption rate produced by DβHB could be blocked by antimycin A, a complex III inhibitor (base line, 4.49 ± 0.62 nmol/min/mg; DβHB, 14.14 ± 0.43 nmol/min/mg; DβHB + antimycin A, 5.99 ± 0.95 nmol/min/mg; n = 3 per group; P > 0.05 comparing base line with the DβHB + antimycin A group). These experiments indicate that DβHB does not uncouple mitochondria at concentrations that increased oxygen consumption.
Effects of DβHB on mitochondrial respiration seem driven by complex II.
One product generated from the metabolism of DβHB is NADH, which provides the driving force for the mitochondrial respiration through complex I. Can an increase in availability of NADH compensate for the loss of oxygen consumption due to complex I inhibition? To test this possibility, freeze-thawed disrupted brain mitochondria were incubated with MPP+, or rotenone, and NADH. Concentrations of MPP+ and rotenone were selected to produce complex I inhibition ranging from about 40% to 100%, and supplementation of NADH ranged from 0.5 to 2.5 times the normal concentration used in the assay (Figure (Figure5,5, a and b). These changes in NADH supplementation did not modify the degree of complex I inhibition (Figure (Figure5,5, a and b). This indicates that DβHB-derived NADH cannot explain the improvement seen in mitochondrial respiration produced by DβHB.
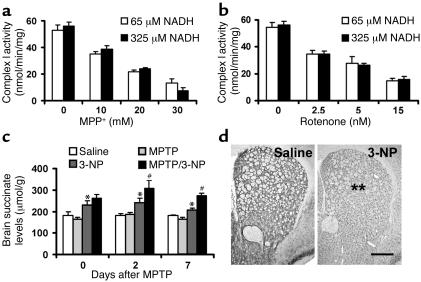
Dose-response study of NADH in complex I activity (a and b) and brain levels of succinate (c). In mitochondria lysed by freeze-thawing, when the inhibition of complex I activity was titrated with different concentrations of MPP+ (a) or rotenone (b), different amounts of NADH did not produce different responses in complex I activity (n = 4 per group). (c) Levels of succinate were measured in the brains of animals treated with 18 mg/kg/d MPTP or 15 mg/kg 3-NP, or both. Levels of succinate in the group that received DβHB (1.6 mmol/kg/d) are significantly increased in the presence of 3-NP. n = 3–10 per group; *P < 0.05 compared with the control saline group; #P < 0.05 compared with the 3-NP group. (d) Histochemical analysis in striatal sections shows that when animals were treated with 3-NP (right panel) at this concentration for 8 days, there was approximately 40% reduction in complex II activity in the striatum compared with that in the group treated with saline (left panel). n = 5 per group; **P < 0.01. Scale bar: 500 μm.
Based on its metabolic pathway, DβHB can also generate succinate, which is capable of stimulating the rate of oxygen consumption in isolated brain mitochondria through complex II. In keeping with this metabolic pathway, we found that both DβHB and succinate did improve oxygen consumption in a dose-dependent and saturable manner, although DβHB was not as potent as succinate (Figure (Figure4d).4d). This is not unexpected, since DβHB has to go through several metabolic steps to generate succinate. In addition, we found that the beneficial effects of DβHB on mitochondrial respiration in the presence of MPP+ or rotenone were completely abolished by two different complex II inhibitors, 3-NP at 10 mM (Figure (Figure4c)4c) and malonate at 10 mM (data not shown). Together, these data are consistent with the idea that DβHB increases mitochondrial respiration in the face of complex I inhibition by a complex II–dependent mechanism.
DβHB neuroprotection is abrogated by mitochondrial complex II inhibition in vivo.
To determine whether our in vitro data are relevant to DβHB neuroprotection seen in vivo, we first measured succinate levels in the brains of DβHB-infused mice. Upon inhibition of complex II, DβHB infusion indeed increased levels of succinate in the brain (Figure (Figure5c).5c). Next, MPTP-injected mice infused with DβHB were injected with 3-NP. This irreversible complex II inhibitor was administered daily for the entire period of DβHB infusion at a dosage of 15 mg/kg/d. As illustrated in Figure Figure5d,5d, this regimen of 3-NP inhibited approximately 40% of complex II activity in the striatum without causing cell death in either the SNpc (Table (Table1)1) or the striatum, as evidenced by TH or Nissl staining (Table (Table1;1; data not shown for striatal Nissl staining). As before, DβHB protected against MPTP neurotoxicity in mice that did not receive 3-NP. However, DβHB failed to reduce MPTP-induced dopaminergic neurodegeneration in mice that did receive 3-NP (Table (Table1;1; Figure Figure2,2, h and p). Supporting the effectiveness of the 3-NP regimen in blocking complex II is our demonstration that succinate levels in the brain were higher in mice that received 3-NP than in those that did not (Figure (Figure5c).5c). Thus, these results are consistent with the hypothesis that complex II is a pivotal mediator in DβHB’s neuroprotective effects.
DβHB does not have antioxidant effects but increases ATP production.
Inhibition of complex I by MPP+ and rotenone generates reactive oxygen species (ROS), raising the possibility that the beneficial effects of DβHB are mediated by an antioxidant action, as previously suggested (14). In isolated mitochondria, DβHB did not reduce but stimulated ROS production in the presence of rotenone or MPP+ (see Table Table4).4). To elucidate the basis of DβHB-related ROS production, 3-NP was added to the incubation mixture (see Table Table4).4). This complex II inhibitor was unable to block the DβHB-related ROS production, thus ruling out the possibility of a reversed flux of electrons from complex II to complex I as the ROS generator (22, 23). Instead, we suspected that the DβHB-related ROS resulted from additional NADH generated by DβHB metabolism. To test this alternative possibility, phenazine methosulfate, a compound that oxidizes NADH (21), was included in the incubation mixture. Consistent with this possibility, phenazine methosulfate abolished ROS production (see Table Table4).4). These data argue against DβHB having antioxidant properties, at least in this in vitro setting.
Table 4
H2O2 measurements in purified brain mitochondria
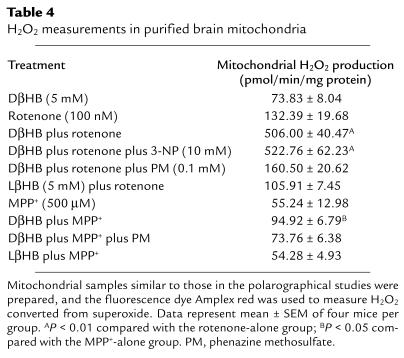
Inhibition of complex I by MPP+ and rotenone also impairs ATP production, raising the possibility that the beneficial effects of DβHB are mediated by attenuation of ATP depletion. We thus measured ATP production in isolated brain mitochondria under conditions similar to those of polarographical study. As shown in Table Table3,3, DβHB increased ATP production from a base line of 5.37 ± 0.30 nmol/mg protein to 76.16 ± 6.11 nmol/mg protein. The increase of ATP production was not detected with the inactive isomer LβHB (3.85 ± 0.24 nmol/mg protein). In agreement with the oxygen-consumption data, DβHB prevented the loss of ATP production caused by 100 μM MPP+ or 100 nM rotenone (Table (Table3).3). Yet, upon addition of 3-NP, DβHB-related ATP production was abolished (Table (Table3).3). Together, these data are consistent with the contention that the effects of DβHB seen in the polarographical studies correspond to an increase in oxidative phosphorylation.
Table 3
ATP levels in purified brain mitochondria
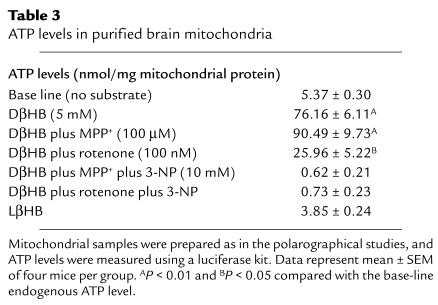
Discussion
The present study shows that the ketone body DβHB, a crucial alternative source of glucose for brain energy, confers protection against the structural and functional deleterious effects of the parkinsonian toxin MPTP; these include degeneration of SNpc dopaminergic neurons and striatal dopaminergic fibers, loss of striatal dopamine, and PD-like motor deficit. The beneficial effects of DβHB were achieved by its subcutaneous infusion using osmotic mini-osmotic pumps, which, without apparent distress, allowed its reliable continuous delivery to the brain. While DβHB levels in the brain were stable in DβHB-infused mice exposed to MPTP, in mice injected with saline they were higher at the beginning and then dropped during the experimental period of 7 days. Although the basis for these differences remains to be elucidated, it is possible that the utilization of DβHB in the brain increases rapidly following exposure to mitochondrial poisons such as MPTP and augments progressively in normal brain as part of a metabolic adaptation to sustained high DβHB concentrations.
Utilization of DβHB in the brain is contingent on its conversion to acetoacetate by β-hydroxybutyrate dehydrogenase, which is scarce in the adult brain, especially in the basal ganglia (35). The activity of β-hydroxybutyrate dehydrogenase correlates with its protein content (36), and, following MPTP administration, it is upregulated in the ventral midbrain. MPTP-induced β-hydroxybutyrate dehydrogenase upregulation precedes peak dopaminergic neuronal death in this model (37). It can thus be envisioned that β-hydroxybutyrate dehydrogenase activity increases early enough to allow effective utilization of DβHB by the compromised dopaminergic neurons.
A critical step in activation of MPTP is its conversion into MPP+ by monoamine oxidase (38). The possibility that DβHB infusion confers protection by interfering with monoamine oxidase activity can be ruled out given the fact that brain levels of MPP+ were similar between mice that received and those that did not receive DβHB. Also arguing against the possibility that DβHB confers protection by impairing MPTP activation is the fact that DβHB attenuates dopaminergic neuronal death in primary ventral midbrain cultures exposed to MPP+ (14). DβHB also did not interfere with other key aspects of MPTP metabolism (39), such as entry of MPP+ into dopaminergic neurons and mitochondria at concentrations as high as 5 mM. Together these data indicate that DβHB protects not by a pre–complex I mechanism but rather by mitigating the deleterious effects of complex I inhibition on the survival of dopaminergic neurons.
In isolated brain mitochondria, DβHB improves oxygen consumption in the presence of the complex I poisons MPP+ and rotenone. The DβHB effect is dose dependent and stereospecific. The metabolism of DβHB leads to an elevated mitochondrial [NADH]/[NAD+] ratio due to NADH generated from the conversion of DβHB to acetoacetate and also from the tricarboxylic acid (TCA) cycle, whose turnover is increased by high levels of acetyl-CoA produced by acetoacetate. NADH is used by complex I to drive mitochondrial respiration. DβHB may increase oxygen consumption by fueling mitochondria with NADH. However, in the presence of complex I inhibition by MPP+ or rotenone, NADH oxidation is impaired and, as shown in this study, an increase in NADH content is unable to alleviate complex I blockade.
In addition to generating NADH, increased TCA turnover, in theory, should also lead to increases in production of other TCA intermediates such as succinate. Here, we show that DβHB infusion does increase brain succinate content. While succinate is a TCA cycle substrate, its oxidation by succinate dehydrogenase is coupled to a transfer of electrons to ubiquinone of the mitochondrial respiratory chain, and thus succinate is routinely used to support oxygen consumption in the presence of complex I blockade. We demonstrate that inhibition of complex II (a) abrogates DβHB-mediated increases in oxygen consumption in isolated mitochondria and (b) abolishes DβHB-mediated protective effects on SNpc dopaminergic neurons and striatal dopaminergic fibers after MPTP administration. Thus, these data strongly support our hypothesis that the beneficial effect of DβHB in the MPTP model of PD involves a complex II–dependent mechanism.
It has been proposed that the ability of DβHB to decrease MPP+ neurotoxicity in primary ventral midbrain cultures is related to the oxidation of the coenzyme Q couple, which should, by decreasing the semiquinone, decrease ROS production (14). Contrary to this prediction, we found, at least in isolated mitochondria, that rather than decreasing ROS production induced by MPP+ or rotenone, DβHB enhanced it even further. These findings cast doubt that DβHB protects the nigrostriatal pathway through an antioxidant mechanism. How can DβHB increase ROS? Succinate is the most effective ROS-generating substrate in intact brain mitochondria (22, 23), by stimulating a reversed flux of electrons from complex II to complex I (22, 23). However, rotenone blocks this ROS signal (22, 23); thus, in the context of the present study, in which complex I is inhibited, this mechanism may not be operative. Instead, our data suggest that DβHB-derived NADH, by feeding complex I, increases the accumulation of electrons upstream to the blockade, thereby stimulating ROS production.
Mitochondrial respiration is tightly linked to ATP synthesis (40). It may thus be speculated that DβHB, by restoring oxygen consumption in MPTP-intoxicated animals, may increase ATP cellular stores. Ablation and inhibition of poly(ADP-ribose) polymerase-1 (41, 42) and creatine supplements (43) mitigate MPTP-induced death of dopaminergic neurons in the SNpc by buffering ATP depletion. These studies underscore the importance of ATP deficit in the MPTP neurodegenerative process. In normal rodents, dopaminergic structures represent less than 15% of the cellular elements in the striatum (44) and hardly more in the ventral midbrain. This renders precarious any detection of ATP changes in brain tissues of MPTP-intoxicated mice (45). To avoid this problem, we studied the effects of DβHB on ATP production in isolated brain mitochondria. By this approach, we were able to demonstrate that DβHB does increase ATP levels in both the absence and the presence of complex I inhibitors. Consistent with the oxygen-consumption data, we also found that the stimulation of ATP production by DβHB likely relies on complex II, as inhibitors of this electron transport chain enzyme eliminated the effect. Data generated in isolated mitochondria may only approximate the more complex situation found in vivo. Despite this caveat, we believe that the most parsimonious explanation for DβHB-induced neuroprotection in the MPTP model of PD is that energy crisis is attenuated by an enhancement of oxidative phosphorylation. It is thus tempting to conclude that, under the current DβHB regimen, the benefit due to the improved ATP production overcomes the possible detriment due to the increased ROS formation in this PD model.
The present study demonstrates that modulation of body DβHB levels may be a straightforward neuroprotective strategy for the treatment of neurodegenerative diseases such as PD. Relevant to this view is the demonstration that mice subjected to dietary restriction (e.g., alternate-day fasting) exhibit higher serum DβHB concentrations and are more resistant to kainic acid–induced hippocampus damage (46) and to MPTP-induced SNpc damage (47). At this point, however, the long-term effects of the chronic use of DβHB on the cell metabolism and, especially, on the mitochondrial function are not known. DβHB has been administered orally for several months to two 6-month-old infants with hyperinsulinemic hypoglycemia (48). Despite the high dosage (up to 32 g/d), these patients seem to tolerate quite well. In addition, the ketogenic diets, which result in high levels of DβHB, have been used for more than 70 years in humans as a treatment for refractory epilepsy and have proven safe and well tolerated.
Acknowledgments
We wish to thank Andrew Marks (Columbia University) for his generous gift of the antibody against β-hydroxybutyrate dehydrogenase, Norma Romero for her assistance in animal care, and Soliman Bakr for his assistance in the measurement of succinate and of DβHB, Salvatore DiMauro (Columbia University), Richard L. Veech (NIH), Ian J. Reynolds (University of Pittsburgh, Pittsburgh, Pennsylvania, USA), and Gary Fiskum (University of Maryland, Baltimore, Maryland, USA), for their insightful comments on this manuscript. This study is supported by NIH/National Institute of Neurological Disorders and Stroke grants R29 NS37345, R01 NS38586, R01 NS42269, P50 NS38370, and P01 NS11766-27A1; US Department of Defense grants DAMD 17-99-1-9471 and DAMD 17-03-1; the Lowenstein Foundation; the Lillian Goldman Charitable Trust; and the Parkinson’s Disease Foundation. P. Teismann is the recipient of grant TE 343/1-1 from the German Research Foundation.
Footnotes
Conflict of interest: The authors have declared that no conflict of interest exists.
Nonstandard abbreviations used: Parkinson disease (PD); substantia nigra pars compacta (SNpc); 1-methyl-4-phenyl-1,2,3,6-tetrahydropyridine (MPTP); D-β-hydroxybutyrate (DβHB); l-β-hydroxybutyrate (LβHB); 3-nitropropionic acid (3-NP); tyrosine hydroxylase (TH); 1-methyl-4-phenylpyridinium (MPP+); carbonylcyanide p-trifluoromethoxyphenylhydrazone (FCCP); transmembrane potential (Δψm); arbitrary fluorescence units (AFUs); dihydroxyphenylacetic acid (DOPAC); homovanillic acid (HVA); reactive oxygen species (ROS); tricarboxylic acid (TCA).
References
Articles from The Journal of Clinical Investigation are provided here courtesy of American Society for Clinical Investigation
Citations & impact
Impact metrics
Citations of article over time
Alternative metrics
Smart citations by scite.ai
Explore citation contexts and check if this article has been
supported or disputed.
https://scite.ai/reports/10.1172/jci18797
Article citations
Small Molecules, α-Synuclein Pathology, and the Search for Effective Treatments in Parkinson's Disease.
Int J Mol Sci, 25(20):11198, 18 Oct 2024
Cited by: 0 articles | PMID: 39456980 | PMCID: PMC11508228
Review Free full text in Europe PMC
Comparative Efficacy of Low-Carbohydrate and Ketogenic Diets on Diabetic Retinopathy and Oxidative Stress in High-Fat Diet-Induced Diabetic Rats.
Nutrients, 16(18):3074, 12 Sep 2024
Cited by: 0 articles | PMID: 39339674 | PMCID: PMC11435414
Crossing epigenetic frontiers: the intersection of novel histone modifications and diseases.
Signal Transduct Target Ther, 9(1):232, 16 Sep 2024
Cited by: 1 article | PMID: 39278916 | PMCID: PMC11403012
Review Free full text in Europe PMC
Implications of Butyrate Signaling Pathways on the Motor Symptomatology of Parkinson's Disease and Neuroprotective Effects-Therapeutic Approaches: A Systematic Review.
Int J Mol Sci, 25(16):8998, 19 Aug 2024
Cited by: 0 articles | PMID: 39201684 | PMCID: PMC11354563
Review Free full text in Europe PMC
Exploring the ketogenic diet's potential in reducing neuroinflammation and modulating immune responses.
Front Immunol, 15:1425816, 12 Aug 2024
Cited by: 0 articles | PMID: 39188713 | PMCID: PMC11345202
Review Free full text in Europe PMC
Go to all (291) article citations
Similar Articles
To arrive at the top five similar articles we use a word-weighted algorithm to compare words from the Title and Abstract of each citation.
Protective role of SIRT5 against motor deficit and dopaminergic degeneration in MPTP-induced mice model of Parkinson's disease.
Behav Brain Res, 281:215-221, 23 Dec 2014
Cited by: 58 articles | PMID: 25541039
L-3-hydroxyacyl-CoA dehydrogenase II protects in a model of Parkinson's disease.
Ann Neurol, 56(1):51-60, 01 Jul 2004
Cited by: 31 articles | PMID: 15236401
Systemically administered neuregulin-1β1 rescues nigral dopaminergic neurons via the ErbB4 receptor tyrosine kinase in MPTP mouse models of Parkinson's disease.
J Neurochem, 133(4):590-597, 26 Jan 2015
Cited by: 16 articles | PMID: 25581060
Mitochondrial dysfunction in Parkinson's disease.
Biochem Soc Symp, 66:85-97, 01 Jan 1999
Cited by: 167 articles | PMID: 10989660
Review
Funding
Funders who supported this work.
NINDS NIH HHS (9)
Grant ID: R01 NS038586
Grant ID: P50 NS38370
Grant ID: P50 NS038370
Grant ID: R29NS37345
Grant ID: R01 NS042269
Grant ID: P01 NS11766-27A1
Grant ID: R01 NS38586
Grant ID: R01 NS42269
Grant ID: P01 NS011766