Abstract
Free full text

Axial Dynamics, Stability, and Interspecies Similarity of Bacterial Community Structure in the Highly Compartmentalized Gut of Soil-Feeding Termites (Cubitermes spp.)
Abstract
The highly compartmentalized gut of soil-feeding termites is characterized by pronounced axial dynamics in physicochemical conditions and microbial processes. In a companion paper (D. Schmitt-Wagner, M. W. Friedrich, B. Wagner, and A. Brune, Appl. Environ. Microbiol. 69:6007-6017, 2003), we demonstrated that the variety of physicochemical conditions in the different gut compartments of Cubitermes spp. is reflected in the diversity of the respective intestinal microbial communities. Here, we used molecular fingerprints of 16S rRNA genes of the bacterial community, obtained by terminal restriction fragment length polymorphism (T-RFLP) analysis, to describe the axial dynamics of the bacterial community structure in the different gut sections. Comparison of the T-RFLP profiles with the predicted terminal restriction fragments of the clones in clone libraries of the gut segments in Cubitermes orthognathus confirmed that all hindgut sections harbored distinct bacterial communities. Morisita indices of community similarity, calculated by comparing the different patterns, revealed large differences between the bacterial communities of soil, gut, and nest material and also among the individual gut sections. By contrast, comparison of the homologous gut segments of different Cubitermes species indicated that the three termite species investigated possessed a similar, gut-specific microbiota that remained comparatively stable even during several months of maintenance in the laboratory.
The hindgut of soil-feeding termites is highly compartmentalized and characterized by pronounced axial dynamics of oxygen, intestinal pH, and redox potential (1, 3, 4, 14) and of microbial processes such as hydrogen production, methanogenesis, and reductive acetogenesis (26, 29). Recent studies have provided strong evidence for an important role of the special physicochemical gut conditions and the pronounced intestinal microbiota in the chemical and microbial transformation of organic matter and microbial biomass during gut passage (11, 12, 13).
A study of the phylogenetic diversity and axial distribution of microorganisms in the intestinal tract of Cubitermes orthognathus revealed that this variety of physicochemical conditions is reflected in the diversity of microbial communities in the different gut compartments and provided first evidence for the presence of a specific intestinal bacterial community (27).
Although sequencing and phylogenetic analysis of cloned 16S rRNA genes provides information on the different phylotypes in a given community, the effort and costs involved in this approach limit investigations of changes in the structure of complex microbial communities over space and time. Molecular fingerprinting methods such as denaturing gradient gel electrophoresis (8, 21, 22) and terminal restriction fragment length polymorphism (T-RFLP) (9, 18, 19) analyses avoid these problems and allow the comparison of microbial communities in a larger number of samples, which has made these methods well-established tools in microbial ecology.
A recent study combining clone analysis and T-RFLP to investigate archaeal community structure in the gut of the soil-feeding termite C. orthognathus provided evidence for pronounced differences among the microbiota not only between the gut and the ingested soil, but also among the different compartments of the intestinal tract (9). Based on the information on the phylogenetic diversity of the bacterial microbiota in the major gut compartments of C. orthognathus described in the companion paper (27), the present study employed T-RFLP analysis to follow changes in the bacterial community structure of ingested soil during gut passage. Comparing the terminal restriction fragment patterns of the homologous gut segments of three different species of Cubitermes, the study also addressed questions regarding the specificity of the gut microbiota for the compartments and the temporal stability of gut microbial communities during maintenance of termites under laboratory conditions.
MATERIALS AND METHODS
Termites and DNA extraction.
Cubitermes orthognathus Emerson was collected in grassland near Busia, and Cubitermes ugandensis Fuller was collected in a glade of the Kakamega rainforest; both sites are located in the highlands of Western Kenya. Species were identified by Julius Muli, National Museums of Kenya. Cubitermes niokoloensis was collected in a dry savannah near Kolda, Senegal, and identified by Alain Brauman, Institut de Recherche pour le Développement, Dakar, Senegal. In addition, partial sequences (≈650 bp) of the mitochondrial cytochrome oxidase II (COII) gene were determined by PCR of DNA extracts from termite heads with previously described primers (28). Voucher specimens of soldiers and workers preserved in alcohol are available from the corresponding author. Whole nests were transported to the laboratory in their country of origin, where they were cut into pieces; termites were distributed into polypropylene containers containing fragments of the nest and soil collected at about 3 m from the nest. The termites were allowed a few days to reconstruct and fix the nest fragments in the containers before being transported to the laboratory in Konstanz.
Termites were kept at room temperature in the dark. The containers were inspected regularly, and parts of the nest material were removed and replaced with fresh soil; moisture was controlled by spraying the surface of the nest material with water. For the experiments, only worker caste termites were used. DNA was extracted within a week after collection or at the times indicated below. For this purpose, termites were dissected with sterile, fine-tipped forceps, and guts were separated into six sections (27) (Fig. (Fig.11).
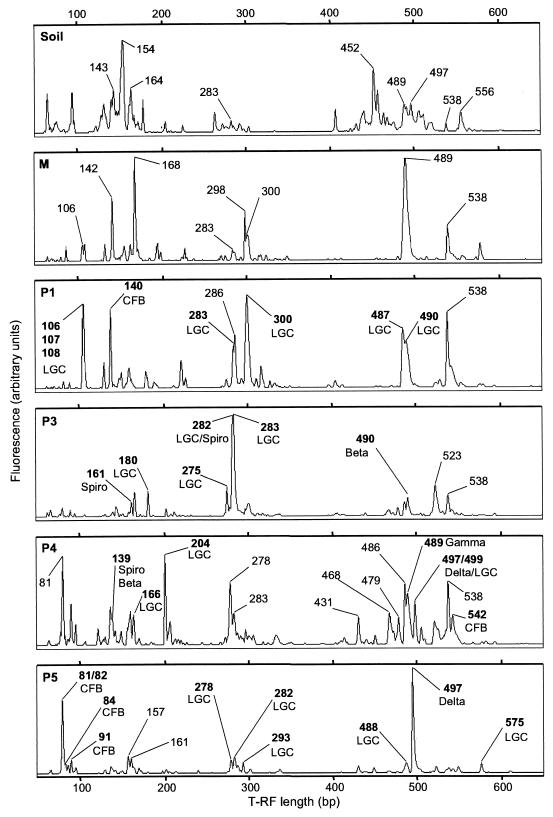
Terminal restriction-fragment length polymorphism (T-RFLP) profiles of 16S rRNA gene fragments, amplified from DNA extracts of different gut segments of C. orthognathus and of the soil from the collection site. PCR products obtained with primers 27F (labeled) and 907R were digested with MspI. Terminal restriction fragment lengths of major peaks (i.e., whose peak height represented more than 2.5% of the sum of all peak heights in the respective terminal restriction fragment pattern) that matched the predicted terminal restriction fragments of clones in the respective clone library are marked in bold and labeled with the phylum of the associated clone (for abbreviations, see Table Table1).1). For orientation, the sizes of several unassigned terminal restriction fragments are also indicated.
Pools of 10 to 20 gut sections each and aliquots (1 g) of soil samples and nest material were extracted with a direct lysis protocol modified after that of Moré et al. (20) that involves bead beating, as described previously in detail (10). DNA was purified from the supernatant by consecutive ammonium acetate, isopropanol, and ethanol precipitation steps. To remove humic substances, the extracts were passed through spin columns filled with polyvinylpolypyrrolidone as described previously (24). DNA concentrations were determined fluorimetrically with Hoechst dye 33258 and a DyNA Quant 200 fluorimeter (Amersham Pharmacia Biotech, Freiburg, Germany) as recommended by the manufacturer. In the case of C. orthognathus, the DNA extracts used for T-RFLP analyses were from the same batch of termites as those used for cloning (27).
T-RFLP analysis.
The procedure used for T-RFLP analysis followed that described in detail by Chin et al. (5), with minor modifications. Bacterial 16S rRNA gene sequences were amplified with 6-carboxyfluorescein-labeled primer 27F and primer 907R (5′-CCG TCA ATT CCT TTR AGT TT-3′ ; Escherichia coli positions 907 to 926) (16). PCR (30 cycles) was carried out as described previously (10), except that the annealing temperature was 52°C. Purified PCR products were quantified spectrophotometrically. PCR amplicons were digested for 3 h at 37°C in 0.5-ml reaction tubes containing 50 ng of DNA, 2.5 U of MspI (Promega), 1 μg of bovine serum albumin, and 1 μl of 10× buffer in a total volume of 10 μl. Samples were analyzed on an ABI 373 sequencer (Applied Biosystems) with a GeneScan-1000 ROX standard (5).
The total peak height of a T-RFLP profile was defined as the sum of the peak heights of all peaks of >50 bp. The relative peak height of a given peak was determined by dividing its peak height by the total peak height of the profile. When determining the number of distinct terminal restriction fragments in a given profile, only terminal restriction fragments with a relative peak height larger than 1% of the total peak height were taken into account.
Community similarity.
The Morisita index (IM) of community similarity (equation 1) (15), where λ is Simpson's index of dominance (calculated separately for each community), ni is the number of individuals of species i, and N is the total number of individuals sampled, was used.
Simpson's index of dominance (equation 2) describes the probability that two randomly selected individuals from a community will be of the same species, where s is the total number of species in the community.
The Morisita index ranges from 0 to 1, with 0 indicating that no species are shared between the two communities and 1 indicating complete identity. Because the index takes species abundance into account, communities that contain the same species but have different species abundance will have an index value of less than 1. These equations were adapted to T-RFLP data by considering each terminal restriction fragment a separate species and peak height a measure of species abundance (7).
RESULTS
T-RFLP fingerprinting.
The T-RFLP fingerprints obtained for the different hindgut segments of Cubitermes orthognathus (Fig. (Fig.1)1) confirmed the high diversity of the bacterial community already evidenced by the clone analysis (in the companion paper [27]). There were striking differences among the fingerprint pattern of the different gut segments, including the midgut, and also to that of the parent soil. In the P1 and especially in the P4 sections, the high microbial diversity was reflected by a large number of peaks in the T-RFLP profile, whereas in the P3 and the P5 sections, only a few fragment lengths dominated the patterns (Fig. (Fig.11).
With the predicted lengths of the terminal restriction fragments for the clonal sequences, a correlation between the peaks in the T-RFLP patterns and the clones in the clone library was established. The 102 clones in the clone library yielded 69 different predicted terminal restriction fragments, and almost every predicted terminal restriction fragment was represented in the T-RFLP fingerprints (Table (Table1).1). Vice versa, almost all major peaks in the T-RFLP patterns could be assigned to clones in the clone libraries (Fig. (Fig.1).1). Some peaks matched the predicted terminal restriction fragments of more than one clone (e.g., four P1 clones with different sequences had the same predicted terminal restriction fragment of 106 bp). Often, closely related clones had a very similar predicted terminal restriction fragment length (1- to 2-bp difference), which were not well separated in the T-RFLP patterns (e.g., the predicted terminal restriction fragment length of seven clones of the P1 cluster ranged from 106 to 108 bp).
TABLE 1.
Predicted terminal restriction fragment lengths after MspI digestion and phylogenetic affiliation of the 16S rRNA gene clonesb in the different clone libraries of C. orthognathus
Terminal fragment length (bp) | Clone library (gut section)
| Phylogenetic groupa | Thermal fragment length (bp) | Clone library (gut section)
| Phylogenetic groupa | |||||||
---|---|---|---|---|---|---|---|---|---|---|---|---|
P1 | P3 | P4 | P5 | P1 | P3 | P4 | P5 | |||||
69 | P3-18 | LGC | ||||||||||
81 | P5-23 | CFB | ||||||||||
82 | P5-27 | CFB | ||||||||||
84 | P5-16 | CFB | ||||||||||
P5-25 | ||||||||||||
87 | P5-22 | CFB | ||||||||||
90 | P3-25 | LGC | ||||||||||
91 | P3-3 | P5-30 | CFB | |||||||||
92 | P5-12 | CFB | ||||||||||
93 | P3-11 | CFB | ||||||||||
96 | P3-5 | P5-29 | CFB | |||||||||
106 | P1-3 | LGC | ||||||||||
P1-17 | ||||||||||||
P1-28 | ||||||||||||
P1-29 | ||||||||||||
107 | P1-2 | LGC | ||||||||||
P1-4 | ||||||||||||
108 | P1-8 | LGC | ||||||||||
131 | P5-7 | Delta | ||||||||||
P5-28 | ||||||||||||
135 | P5-11 | LGC | ||||||||||
P5-19 | ||||||||||||
P5-20 | ||||||||||||
139 | P4-2 | Spiro | ||||||||||
139 | P4-5 | Beta | ||||||||||
140 | P1-11 | CFB | ||||||||||
141 | P4-11 | Beta | ||||||||||
152 | P5-18 | Alpha | ||||||||||
153 | P1-22 | LGC | ||||||||||
161 | P3-9 | Spiro | ||||||||||
163 | P3-21 | P5-2 | Unident. | |||||||||
166 | P4-22 | LGC | ||||||||||
166 | P4-6 | Plancto | ||||||||||
171 | P4-16 | LGC | ||||||||||
180 | P3-1 | LGC | ||||||||||
183 | P1-10 | LGC | ||||||||||
188 | P3-20 | LGC | ||||||||||
204 | P4-23 | P5-26 | LGC | |||||||||
P4-26 | ||||||||||||
206 | P5-14 | LGC | ||||||||||
P5-15 | ||||||||||||
210 | P4-12 | LGC | ||||||||||
216 | P5-21 | LGC | ||||||||||
218 | P4-7 | LGC | ||||||||||
219 | P3-30 | LGC | ||||||||||
227 | P1-25 | LGC | ||||||||||
275 | P3-2 | LGC | ||||||||||
278 | P5-4 | LGC | ||||||||||
281 | P1-6 | LGC | ||||||||||
P1-7 | ||||||||||||
282 | P1-9 | P3-29 | P5-10 | LGC | ||||||||
P5-24 | ||||||||||||
282 | P3-22 | Spiro | ||||||||||
283 | P1-18 | P3-6 | LGC | |||||||||
P1-24 | P3-7 | |||||||||||
P3-17 | ||||||||||||
P3-23 | ||||||||||||
P3-24 | ||||||||||||
292 | P1-5 | P3-4 | LGC | |||||||||
P1-13 | ||||||||||||
293 | P5-1 | LGC | ||||||||||
295 | P5-6 | LGC | ||||||||||
296 | P3-12 | LGC | ||||||||||
297 | P1-19 | P4-30 | LGC | |||||||||
299 | P1-1 | LGC | ||||||||||
P1-14 | ||||||||||||
300 | P1-15 | LGC | ||||||||||
P1-21 | ||||||||||||
303 | P4-24 | Unident. | ||||||||||
309 | P3-28 | LGC | ||||||||||
310 | P1-23 | LGC | ||||||||||
373 | P4-15 | Spiro | ||||||||||
385 | P3-15 | LGC | ||||||||||
402 | P1-30 | LGC | ||||||||||
421 | P4-14 | LGC | ||||||||||
430 | P5-5 | Beta | ||||||||||
446 | P3-14 | LGC | ||||||||||
464 | P4-9 | LGC | ||||||||||
487 | P1-16 | LGC | ||||||||||
488 | P1-20 | P5-9 | LGC | |||||||||
489 | P4-8 | Gamma | ||||||||||
490 | P3-26 | P5-8 | Beta | |||||||||
490 | P1-26 | LGC | ||||||||||
497 | P4-29 | P5-3 | Delta | |||||||||
499 | P4-25 | LGC | ||||||||||
512 | P3-19 | LGC | ||||||||||
542 | P4-1 | CFB | ||||||||||
P4-4 | ||||||||||||
575 | P5-13 | LGC |
The large phylogenetic diversity of low G+C content (LGC) clones was reflected in 46 different predicted terminal restriction fragments among a total of 69 clones (Table (Table1).1). Most major peaks in the profile of the P1 segment could be assigned to clones from the termite-specific clusters within the LGC bacteria. Terminal restriction fragments of 106 to 108 bp and 299 to 300 bp, representing all nine clones of the P1 cluster, were present only in the profiles of the P1 segment and the midgut, which is in agreement with the absence of such clones in the clone libraries of the posterior gut sections. In contrast, terminal restriction fragments of 281 to 283 bp, matching the predicted terminal restriction fragments of LGC clones in the Sporobacter cluster (27), were present in the profiles of all segments but represented the largest peak and also the largest number of LGC clones in P3.
The profile of the P4 segment was characterized by a strong increase in terminal restriction fragment length diversity and a significant shift to longer fragment sizes, which were not present in the P3 profile. Also, a number of shorter terminal restriction fragments were not present in the P3 profile. The largest peak of 204 bp matched two LGC clones from the P4 clone library (Table (Table1).1). Several other peaks in this profile matched the predicted terminal restriction fragments of clones from the Cytophaga-Flexibacter-Bacteroides phylum, the spirochetes, or different subgroups of the Proteobacteria. However, two of these peaks could not be clearly assigned because clones from other phyla had similar or identical predicted terminal restriction fragments.
The T-RFLP profile of the P5 segment was dominated by two major peaks. The terminal restriction fragment of 81 bp, which was also present in the profile of P4, and the following smaller peaks (82 to 96 bp) matched a group of eight clones belonging to the Cytophaga-Flexibacter-Bacteroides group from the P5 section clone library (Table (Table1),1), which clustered exclusively with clones derived from the guts of other termites (27). The other major peak in the P5 profile, representing a terminal restriction fragment of 497 bp, matched a clone (P5-3) belonging to the δ-Proteobacteria. This peak was also present in the P4 profile, where it matched another clone belonging to the δ-Proteobacteria (P4-29) closely related to clone P5-3 (27).
The T-RFLP profiles of the soil sample and the nest material were at least as diverse as those of any gut section. However, only a few peaks were shared between soil and midgut, and the peaks with identical terminal restriction fragment lengths changed tremendously in their relative abundance. Terminal restriction fragments representing LGC clones from the termite-specific clusters (106 to 108, 283, and 300 bp) were not present or gave only minor peaks in the soil fingerprints. Also, the characteristic terminal restriction fragments matching the termite-specific clones of the Cytophaga-Flexibacter-Bacteroides group (81, 82, 140, and 542 bp) were not present in the soil sample or the midgut, which indicated that the gut bacterial community is not merely a reflection of that found in the soil and also differs in the individual gut compartments.
Morisita index of community similarity.
Morisita indices were calculated as a measure of community similarity between different pairs of T-RFLP profiles. In most cases, the profiles of neighboring gut sections were more similar than those of sections that were not directly connected to each other (Table (Table2).2). However, in no case did the Morisita indices exceed a value of 0.5, underlining that the community structure in neighboring segments also differed considerably, even if their T-RFLP profiles shared individual peaks. Morisita indices between gut segments and soil were very low, which substantiates that the shift from the soil to the gut community takes place in the foregut and is completed in the midgut. It is interesting that there was also little similarity in the profiles of the rectum (P5) and the nest material, indicating that the microbial community also changes strongly between rectal contents and nest material (which is constructed from soil and feces). The highest Morisita index (0.76) was found when the T-RFLP profile of the soil was compared to that of the nest material.
TABLE 2.
Morisita indices of community similarity between the soil from the collection site, the different gut sections of C. orthognathus, and the nest materiala
Sample | Morisita index
| ||||||
---|---|---|---|---|---|---|---|
Crop | Midgut | P1 | P3 | P4 | P5 | Nest | |
Soil | 0.27 | 0.26 | 0.12 | 0.09 | 0.17 | 0.14 | 0.76 |
Crop | 0.01 | 0.17 | 0.11 | 0.13 | 0.25 | 0.15 | |
Midgut | 0.32 | 0.17 | 0.22 | 0.04 | 0.34 | ||
P1 | 0.28 | 0.35 | 0.10 | 0.16 | |||
P3 | 0.29 | 0.15 | 0.15 | ||||
P4 | 0.49 | 0.14 | |||||
P5 | 0.08 |
Low similarities between T-RFLP profiles of the individual hindgut segments were also obtained with Cubitermes ugandensis and Cubitermes niokoloensis (Table (Table3),3), confirming that each segment is colonized by a specific bacterial community. When the profiles obtained for the hindgut segments of C. orthognathus were compared with those of the other two species (Table (Table4),4), the Morisita indices were much higher than those between the individual gut segments of the same termite, which indicates that homologous hindgut segments harbor similar microbial communities. The highest value (0.97) was obtained for the P3 segments of C. ugandensis and C. niokoloensis, which indicates an almost identical profile.
TABLE 3.
Morisita indices of community similarity between the four major hindgut segments of Cubitermes ugandensis and Cubitermes niokoloensis.
Species and segment | Morisita index
| ||
---|---|---|---|
P3 | P4 | P5 | |
C. ugandensis | |||
![]() ![]() ![]() ![]() | 0.14 | 0.28 | 0.15 |
![]() ![]() ![]() ![]() | 0.32 | 0.21 | |
![]() ![]() ![]() ![]() | 0.34 | ||
C. niokoloensis | |||
![]() ![]() ![]() ![]() | 0.12 | 0.08 | 0.15 |
![]() ![]() ![]() ![]() | 0.19 | 0.15 | |
![]() ![]() ![]() ![]() | 0.36 |
TABLE 4.
Morisita indices of community similarity in homologous hindgut segments among three different species of Cubitermes
Segment and species | Morisita index
| |
---|---|---|
C. ugandensis | C. niokoloensis | |
P1 | ||
![]() ![]() ![]() ![]() | 0.89 | 0.43 |
![]() ![]() ![]() ![]() | 0.48 | |
P3 | ||
![]() ![]() ![]() ![]() | 0.69 | 0.67 |
![]() ![]() ![]() ![]() | 0.97 | |
P4 | ||
![]() ![]() ![]() ![]() | 0.57 | 0.40 |
![]() ![]() ![]() ![]() | 0.58 | |
P5 | ||
![]() ![]() ![]() ![]() | 0.70 | 0.41 |
![]() ![]() ![]() ![]() | 0.54 |
T-RFLP analysis was used also to assess the temporal stability of the bacterial communities in the different gut segments of C. ugandensis. Profiles were obtained directly after collection and after 2 and 5 months of storage of the termites in the laboratory, and Morisita indices were calculated for the samples of each gut section collected at different times (Table (Table5).5). Shifts in community structure were evident in the case of the P1 and P4 segments and seemed to occur mostly during the first 2 months after collection. However, the high similarity of the P3 profiles documents considerable stability of the microbial community of this segment over time.
TABLE 5.
Morisita indices documenting changes in community structure within the individual hindgut segments of C. ugandensis during maintenance of the termites in the laboratorya
Time interval (mo) | Morisita index
| |||
---|---|---|---|---|
P1 | P3 | P4 | P5 | |
0-2 | 0.77 | 0.94 | 0.50 | 0.80 |
2-5 | 0.88 | 0.92 | 0.84 | 0.80 |
0-5 | 0.62 | 0.93 | 0.50 | 0.76 |
DISCUSSION
The results of this study clearly demonstrate that the gut of soil-feeding termites (Cubitermes spp.) harbors a specific bacterial microbiota and that structural and functional differences of the individual gut segments are reflected by changes in the composition of the bacterial community. The combined results of the molecular fingerprints obtained in the present study and the results of clone analysis and fluorescence in situ hybridization presented in the companion paper (27) provide strong support for the presence of specific bacterial populations in the different gut compartments. This is in agreement with a previous cultivation-independent study that demonstrated the presence of specific populations of methanogens in the different gut segments of C. orthognathus (9).
Soils and intestinal tracts are environments with highly complex microbial communities. There is good evidence for several thousand microbial species in a single soil sample (25, 30), and the gastrointestinal tract of pigs contains almost 400 different bacterial phylotypes when a 16S rRNA gene sequence similarity of <97% is used as a differential criterion (17). Therefore, a cloning approach may suffice to characterize microbial communities at the phylogenetic-group level, but cannot be used to document detailed differences in community structure with the necessary resolution.
Fingerprinting techniques, on the other hand, are able to generate an image of all gene fragments amplified from the community rather than just a clonal subset. Moreover, the integrative nature of the method, i.e., the ability to sum all clones of a phylogenetic cluster within the same restriction fragment, helps to reduce the complexity to a manageable format and offers the possibility of comparing a large number of samples.
Since clones from different phylogenetic groups could have identical first restriction sites, which could result in identical terminal restriction fragment lengths, a single peak in a T-RFLP profile does not necessarily represent only one phylogenetic group. For this reason, T-RFLP should always be combined with sequence data from clone analysis (9) and, if necessary, performed with more than one restriction enzyme. The advantages of this approach are documented by the combined results of the present study and its companion (27). The fingerprint patterns of the different gut sections of C. orthognathus and the other Cubitermes spp. document that the compositions of the complex microbial communities of the individual segments consist of different phylotypes. However, only when the individual terminal restriction fragments were assigned to their corresponding clones in the clone libraries did it become apparent that the pattern of P1 is caused mainly by bacteria from one phylum (the LGC group), whereas that of the P4 segment is due the presence of bacteria from a variety of phyla.
Presence of gut-specific microbiota.
Mathematical approaches such as the Morisita index provide an objective measure of community similarity in different samples (15). This procedure not only allows a peak-to-peak pairwise comparison of the presence and relative abundance of terminal restriction fragments in the different patterns but is also insensitive to differences in the absolute signal between the profiles. Although originally introduced into ecological research to compare communities at the species level, it has been adapted for use with T-RFLP profiles by treating the individual terminal restriction fragments like different species (7). Although the operational definition has its weaknesses (one peak may represent more than one phylotype), it is nevertheless a powerful tool to compare complex community profiles with a single index value.
The composition of the microbial soil community and also of the nest material is fundamentally different from that in the intestinal tract of soil-feeding termites. The Morisita indices show distinct differences between soil and gut bacterial communities. Also, the microbiota colonizing the nest material, which is largely constructed of soil and feces (6), resembles that of the soil rather than that of the rectal contents, adding further evidence for the presence of a gut-specific microbiota.
Community structure in different gut compartments.
In general, the differences between the T-RFLP profiles of soil and anterior hindgut sections are enormous. Extremely low Morisita indices were obtained between soil and crop or midgut samples, which indicated that the changes in community structure are not due only to the high alkalinity in the anterior hindgut of Cubitermes spp. (pH > 12 [4]), but probably also due to digestion of soil microorganisms in the anterior gut regions of soil-feeding termites, as discussed in the companion paper (27).
The passage of the gut contents through the intestinal tract is relatively fast; transit times of 36 to 48 h have been reported for the soil-feeding termite Procubitermes aburiensis (2). It is possible that minor populations of allochthonous bacteria are specifically enriched and give rise to transient populations in those gut compartments that provide favorable environmental conditions. On the other hand, the soil collected from the vicinity of the nest will have been processed by soil-feeding termites for many years, and the presence of gut bacteria in soil material has to be expected, especially in the case of the LGC clones, many of which may form endospores. To our knowledge, transfer of microbial symbionts by proctodeal trophallaxis, which is well established in lower termites (23), has not yet been documented for soil-feeding Termitinae. However, even an inoculation of the intestinal tract via fecal material contained in the food soil would not contradict the existence of autochthonous microbial populations, represented by bacterial lineages found (so far) only in the gut of soil-feeding termites.
Also, the relative stability of the community composition over time, as documented for C. ugandensis in this study, supports the presence of gut-specific bacterial communities, especially in the P3 segment. Nevertheless, the fact that the largest changes in community structure occurred during the first 2 months of maintenance in the laboratory underlines that it is important to perform such studies with material preserved immediately after collection.
Interspecies comparison of gut communities.
Further support for the presence of a specific gut microbiota is provided by the observation that the profiles of homologous gut segments in different soil-feeding termite species from different geographic locations were more similar to each other than the profiles of the different gut segments within a single termite species. The specificity of the intestinal microbiota in soil-feeding termites is obviously independent of the geographic location and the soil of the collection site. Although it is not possible to make correlations between T-RFLP profiles and specific genotypes in the absence of a clone library, it is reasonable to assume that the large number of identical terminal restriction fragments reflects the presence of bacteria from phylogenetically related groups and therefore shows a specificity of the microbiota of soil-feeding termites without the restriction of a termite species barrier.
Acknowledgments
This study was supported by the Deutsche Forschungsgemeinschaft and by the Max Planck Society.
We thank Hamadi Boga (Jomo Kenyatta University of Agriculture and Technology, Nairobi, Kenya), Lucie Rogo and Nixon Onyimbo (International Centre of Insect Physiology and Ecology, Nairobi), Wanja Kinuthia and the late Julius Muli (National Museum of Kenya), and Alain Brauman (Institut de Recherche pour le Développement, Dakar, Senegal) for help with termite collection and identification and Karen A. Brune for critically reading the manuscript.
REFERENCES
Articles from Applied and Environmental Microbiology are provided here courtesy of American Society for Microbiology (ASM)
Full text links
Read article at publisher's site: https://doi.org/10.1128/aem.69.10.6018-6024.2003
Read article for free, from open access legal sources, via Unpaywall:
https://europepmc.org/articles/pmc201195?pdf=render
Citations & impact
Impact metrics
Citations of article over time
Smart citations by scite.ai
Explore citation contexts and check if this article has been
supported or disputed.
https://scite.ai/reports/10.1128/aem.69.10.6018-6024.2003
Article citations
Developmental Shifts in the Microbiome of a Cosmopolitan Pest: Unraveling the Role of Wolbachia and Dominant Bacteria.
Insects, 15(2):132, 16 Feb 2024
Cited by: 0 articles | PMID: 38392551 | PMCID: PMC10888865
The gut microbiota diversity of five Orthoptera (Insecta, Polyneoptera) insects determined by DNA metabarcoding.
Biodivers Data J, 11:e98162, 15 Mar 2023
Cited by: 2 articles | PMID: 38327358 | PMCID: PMC10848783
Environmental Factors Affect the Bacterial Community in Diaphorina citri, an Important Vector of "Candidatus Liberibacter asiaticus".
Microbiol Spectr, e0529822, 28 Mar 2023
Cited by: 3 articles | PMID: 36975996 | PMCID: PMC10100744
Gut bacterial communities and their assembly processing in Cnaphalocrocis medinalis from different geographic sources.
Front Microbiol, 13:1035644, 15 Dec 2022
Cited by: 2 articles | PMID: 36590437 | PMCID: PMC9797858
Polyene-Producing Streptomyces spp. From the Fungus-Growing Termite Macrotermes barneyi Exhibit High Inhibitory Activity Against the Antagonistic Fungus Xylaria.
Front Microbiol, 12:649962, 01 Apr 2021
Cited by: 8 articles | PMID: 33868208 | PMCID: PMC8047067
Go to all (48) article citations
Similar Articles
To arrive at the top five similar articles we use a word-weighted algorithm to compare words from the Title and Abstract of each citation.
Phylogenetic diversity, abundance, and axial distribution of bacteria in the intestinal tract of two soil-feeding termites (Cubitermes spp.).
Appl Environ Microbiol, 69(10):6007-6017, 01 Oct 2003
Cited by: 77 articles | PMID: 14532056 | PMCID: PMC201194
Axial differences in community structure of Crenarchaeota and Euryarchaeota in the highly compartmentalized gut of the soil-feeding termite Cubitermes orthognathus.
Appl Environ Microbiol, 67(10):4880-4890, 01 Oct 2001
Cited by: 66 articles | PMID: 11571197 | PMCID: PMC93244
Microbial community structure in midgut and hindgut of the humus-feeding larva of Pachnoda ephippiata (Coleoptera: Scarabaeidae).
Appl Environ Microbiol, 69(11):6659-6668, 01 Nov 2003
Cited by: 113 articles | PMID: 14602626 | PMCID: PMC262301
Molecular phylogenetic identification of the intestinal anaerobic microbial community in the hindgut of the termite, Reticulitermes speratus, without cultivation.
Extremophiles, 2(3):155-161, 01 Aug 1998
Cited by: 24 articles | PMID: 9783160
Review