Abstract
Free full text

A Multispecificity Syntaxin Homologue, Vam3p, Essential for Autophagic and Biosynthetic Protein Transport to the Vacuole
Abstract
Protein transport in eukaryotic cells requires the selective docking and fusion of transport intermediates with the appropriate target membrane. t-SNARE molecules that are associated with distinct intracellular compartments may serve as receptors for transport vesicle docking and membrane fusion through interactions with specific v-SNARE molecules on vesicle membranes, providing the inherent specificity of these reactions. VAM3 encodes a 283–amino acid protein that shares homology with the syntaxin family of t-SNARE molecules. Polyclonal antiserum raised against Vam3p recognized a 35-kD protein that was associated with vacuolar membranes by subcellular fractionation. Null mutants of vam3 exhibited defects in the maturation of multiple vacuolar proteins and contained numerous aberrant membrane-enclosed compartments. To study the primary function of Vam3p, a temperature-sensitive allele of vam3 was generated (vam3tsf). Upon shifting the vam3tsf mutant cells to nonpermissive temperature, an immediate block in protein transport through two distinct biosynthetic routes to the vacuole was observed: transport via both the carboxypeptidase Y pathway and the alkaline phosphatase pathway was inhibited. In addition, vam3tsf cells also exhibited defects in autophagy. Both the delivery of aminopeptidase I and the docking/ fusion of autophagosomes with the vacuole were defective at high temperature. Upon temperature shift, vam3tsf cells accumulated novel membrane compartments, including multivesicular bodies, which may represent blocked transport intermediates. Genetic interactions between VAM3 and a SEC1 family member, VPS33, suggest the two proteins may act together to direct the docking and/or fusion of multiple transport intermediates with the vacuole. Thus, Vam3p appears to function as a multispecificity receptor in heterotypic membrane docking and fusion reactions with the vacuole. Surprisingly, we also found that overexpression of the endosomal t-SNARE, Pep12p, suppressed vam3Δ mutant phenotypes and, likewise, overexpression of Vam3p suppressed the pep12Δ mutant phenotypes. This result indicated that SNAREs alone do not define the specificity of vesicle docking reactions.
In eukaryotic cells, the functional properties of a particular intracellular organelle are largely defined by its unique set of resident proteins. Maintenance of organelle identity therefore requires effective sorting and delivery of proteins. Protein trafficking in the secretory pathway requires a series of events including cargo selection and vesicle budding at the donor organelle, followed by transport, docking, and fusion of transport vesicles with the appropriate target organelle.
Protein transport to the vacuole in the budding yeast Saccharomyces cerevisiae is one system in which these processes have been extensively studied. The yeast vacuole is an acidified compartment analogous to the lysosome of mammalian cells, containing a variety of hydrolytic enzymes that are responsible for macromolecular degradation (Klionsky et al., 1990). Resident vacuolar proteins and proteins destined for degradation are delivered to the vacuole via biosynthetic, endocytic, and autophagic transport routes. Thus, the vacuole represents a site of convergence for these distinct pathways. To identify the protein machinery involved in Golgi to vacuole protein transport, several mutant screens have been developed to detect vacuolar protein sorting (vps) (Bankaitis et al., 1986; Rothman and Stevens, 1986; Robinson et al., 1988; Rothman et al., 1989), vacuolar morphology (vam) (Wada et al., 1992), and vacuolar protease activity (pep) (Jones, 1977) mutants. Through these screens, >40 mutant complementation groups have been identified.
Yeast and mammalian cells appear to share a highly conserved machinery that functions in protein sorting and membrane fusion (Bennett and Scheller, 1993; Ferro-Novick and Jahn, 1994; Rothman, 1994). For example, members of the syntaxin, synaptobrevin/VAMP, Sec1p, and Rab protein families function at multiple stages of the secretory pathway (for reviews see Rothman, 1994; Pfeffer, 1996). Syntaxin (t-SNARE) and synaptobrevin (v-SNARE) family proteins are characterized as cytoplasmically oriented integral membrane proteins containing regions predicted to form coiled-coil domains (Bennett et al., 1993). A predominant model for the function of these proteins postulates that interactions between a particular syntaxin molecule (t-SNARE) localized on the target membrane with its corresponding VAMP/synaptobrevin molecule (v-SNARE) localized on the vesicle membrane provides the specificity required for faithful vesicle-mediated transport (Bennett and Scheller, 1993; Sollner et al., 1993). Members of the Sec1p and Rab families of proteins are thought to regulate the formation and/or activity of these SNARE complexes (for reviews see Novick and Brennwald, 1993; Rothman, 1994).
Several representatives of these conserved protein families have been identified in the vacuolar protein sorting pathway. For example, two distinct Rab proteins, Vps21p and Ypt7p (Wichmann et al., 1992; Horazdovsky et al., 1994; Singer-Kruger et al., 1994), and two distinct Sec1p homologues, Vps33p and Vps45p (Banta et al., 1990; Wada et al., 1990; Cowles et al., 1994; Piper et al., 1994), are required for Golgi to vacuole protein transport. These pairs of proteins define the two distinct stages in Golgi to vacuole protein transport. Pep12p, a syntaxin homologue, Vps45p, and Vps21p have been shown to direct Golgi to endosome protein trafficking (Becherer et al., 1996; Burd et al., 1997). Recently, Vam3p was identified as a syntaxin homologue associated with vacuolar membranes (Wada et al., 1997), suggesting that it may function together with Vps33p and Ypt7p in the endosome to vacuole transport reaction.
We describe experiments that test if Vam3p acts with other VPS gene products to direct endosome to vacuole transport. Both subcellular localization studies and analysis of a vam3tsf mutant indicate that the primary function of Vam3p is at the vacuole where it accepts protein traffic from multiple transport pathways. Genetic studies indicate that Vam3p acts in conjunction with Vps33p, a Sec1p homologue, to execute its function. Finally, suppression studies show that Vam3p and Pep12p can partially substitute for one another, suggesting that SNARE molecules do not constitute the only specificity factor in vesicular targeting and fusion events.
Materials and Methods
Strains and Media
S. cerevisiae strains used for these studies are listed in Table TableI.I. Yeast strains were grown in standard yeast extract/peptone/dextrose (YPD),1 yeast extract/peptone/fructose (YPF), or synthetic medium (YNB) containing 2% dextrose and supplemented as necessary with essential amino acids (Sherman et al., 1979). Standard bacterial medium (Miller, 1972) supplemented with 100 μg/ml ampicillin for plasmid retention was used to propagate Escherichia coli. Yeast strains used in EM examination of autophagy were grown in synthetic nitrogen starvation medium without amino acids and ammonium sulfate (SD-N) (Takeshige et al., 1992). Transformation of S. cerevisiae was done by the lithium acetate method (Ito et al., 1983). E. coli transformations were done as described previously (Hanahan, 1983).
Table I
Saccharomyces cerevisiae Strains Used in This Study
Strain | Genotype | Reference or source | ||
---|---|---|---|---|
SEY6210 | MATα leu2-3,112 ura3-52 his3-Δ200 trpl-Δ901 lys2-801 suc2-Δ9 | Robinson et al., 1988 | ||
BHY10 | SEY6210; leu2-3,112::pBHY11(CPY-Inv LEU2) | Horazdovsky et al., 1994 | ||
CJY1 | BHY10; vam3Δ::HIS3 | This study | ||
TDY1 | SEY6210; vam3Δ::HIS3 | This study | ||
TDY2 | SEY6210; vam3Δ::LEU2 | This study | ||
CBY31 | SEY6210; pep12Δ::HIS3 | Burd et al., 1997 | ||
LBY317 | SEY6210; vps33Δ::HIS3 | Banta et al., 1990 | ||
TDY7 | SEY6210; vam3Δ::LEU2 vps33Δ::HIS3 | This study | ||
TDY10 | SEY6210; vam3Δ::HIS3 pep4Δ::LEU | This study | ||
TDY10 | SEY6210; vam3Δ::HIS3 pep4Δ::LEU2 | This study | ||
CBY12 | SEY6210; pep12Δ::LEU2 vps45::HIS3 | Burd et al., 1997 | ||
CCY120 | SEY6210; vps45Δ::HIS3 | Cowles et al., 1994 |
DNA Methods
Recombinant DNA manipulations were performed using standard methods (Maniatis et al., 1982). Restriction and modification enzymes were purchased from Boehringer Mannheim Biochemicals (Indianapolis, IN) and New England Biolabs (Beverly, MA). A YEp13-based genomic library plasmid (p351R1) containing the VAM3 open reading frame (ORF) and a vam3::HIS3 deletion construct were a generous gift from Yoh Wada (University of Tokyo, Japan) (Wada et al., 1997). Plasmids pVAM3.BS, pVAM3.414, pVAM3.424, and pVAM3.416 were generated by subcloning the 2.4-kb BstBI–NsiI fragment (containing the entire VAM3 coding sequence) of p351R1 into pBluescript KS(−) (Stratagene, La Jolla, CA), pRS414, pRS424, and pRS416 (Sikorski and Hieter, 1989), respectively. A VAM3 deletion construct was generated by replacing the BsmI fragment of pVAM3.BS (eliminating 75% of the VAM3 coding sequence) with the LEU2 gene. A linear DNA fragment containing the deletion construct was generated by PCR with primers complementary to the VAM3 sequence. Transformation of wild-type cells with the deletion construct resulted in homologous recombination and insertion of the auxotrophic marker at the chromosomal VAM3 locus. Transformants were selected by amino acid prototrophy and deletions were confirmed by PCR analysis of the chromosomal DNA. Plasmid pVAM3-6.414 containing a temperature-conditional allele of vam3 (vam3.6) was constructed by gapped plasmid repair (Muhlrad et al., 1992). The BamHI–KpnI fragment of pVAM3-6.414 containing vam3.6 was then subcloned into pRS416 (Sikorski and Hieter, 1989) to generate pVAM3-6.416. Plasmid pVPS33-8.415 containing a temperature-conditional allele of vps33 (vps33.8) was generated by gapped plasmid repair. The SalI–NotI fragment of pVPS33-8.415 containing the vps33.8 allele was subsequently subcloned into pRS416 (Sikorski and Hieter, 1989) to generate pVPS33-8.416. Plasmids pCYI50, pCB31, and pLB221 were described previously (Johnson et al., 1987; Banta et al., 1990; Burd et al., 1997).
Metabolic Labeling and Immunoprecipitation
To examine the biosynthetic transport of vacuolar proteins, cells were grown at 26°C in synthetic medium supplemented with amino acids to an OD600 of 0.5–1.0. Cells were harvested and converted to spheroplasts as described previously (Paravicini et al., 1992). Spheroplasts were resuspended at a concentration of 3 OD600/ml in synthetic medium containing amino acids and supplemented with 100 μg/ml α2-macroglobulin and 1 μg/ml BSA to stabilize secreted proteins. Cultures were preincubated at the appropriate experimental temperature for 5 min, and then labeled with 60 μCi [35S]cysteine/methionine per ml of cell suspension. After labeling, cultures were chased with the addition of methionine, cysteine, yeast extract, and glucose to final concentrations of 5 mM, 1 mM, 0.4%, and 0.2%, respectively. After appropriate chase periods, samples were harvested into an equal volume of 2× energy poison buffer (40 mM sodium azide, 40 mM sodium fluoride, 50 mM Tris, pH 7.5, and 1 M sorbitol) and stored on ice for 2 min. The spheroplasts were then spun at 13,000 g for 2 min and separated into intracellular and extracellular fractions. The resulting samples were precipitated by the addition of TCA to a final concentration of 10%. For analysis of carboxypeptidase Y (CPY) sorting in vps33-8 cells, spheroplasting was performed after labeling and chase. After the desired chase period, the cultures were harvested into 2× spheroplast buffer (40 mM sodium azide, 40 mM sodium fluoride, 50 mM Tris, pH 7.5, and 2 M sorbitol, 20 mM DTT) and held on ice for 10 min. Zymolase was added to a concentration of 5 μg/OD600 and the cells were incubated at 30°C for 30 min. The resulting spheroplasts were spun at 13,000 g for 2 min and separated into I and E fractions. Proteins were precipitated by the addition of TCA to a final concentration of 10%. Analysis of aminopeptidase I (API) and CPY performed in whole cells was done as previously described (Gaynor et al., 1994). Proteins were immunoprecipitated with specific antibodies as previously described (Rieder et al., 1996). Antibodies to API were a generous gift from Dan Klionsky (University of California, Davis) (Klionsky et al., 1992). Antibodies to CPY, proteinase A (PrA), alkaline phosphatase (ALP), carboxypeptidase S (CPS), Pep12p, and Vps10p have been previously characterized (Klionsky et al., 1988; Klionsky and Emr, 1989; Marcusson et al., 1994; Becherer et al., 1996; Cowles et al., 1997).
Preparation of Antisera against Vam3p
A hybrid protein fusing the amino-terminal 202 amino acids of Vam3p to glutathione-S-transferase (GST) was expressed in E. coli XL1Blue [supE44 thi-1 lac endA1 gyrA96 hsdR17 relA1 F′proAB lacIqZΔM15]. GST–Vam3p fusion protein was isolated by affinity binding to glutathione-coupled Sepharose and further purified by SDS-PAGE. Purified protein was used to immunize New Zealand White rabbits as previously described (Horazdovsky and Emr, 1993). The crude antiserum was affinity purified by binding to cyanogen bromide–coupled GST–Vam3p (Harlow and Lane, 1988). Vam3p was detected in yeast cell lysates by immunoblotting and enhanced chemiluminescence (ECL) detection as previously described (Babst et al., 1997).
Subcellular Fractionation and Gradient Analysis
For intracellular localization of Vam3p, spheroplasts were made from wild-type cells and lysed in hypoosmotic lysis buffer as previously described (Gaynor et al., 1994). The crude lysate was centrifuged at 300 g to remove any unlysed spheroplasts. The 300 g supernatant was sequentially centrifuged at 13,000 g (15 min) and 100,000 g (60 min) to generate both low and high speed pellet and supernatant fractions. Resulting samples were precipitated in 10% TCA and analyzed by immunoblotting and ECL detection as described previously (Babst et al., 1997). Gradient Accudenz (Accurate Chemical and Scientific Corp., Westbury, NY) solutions were prepared (wt/vol) in hypoosmotic lysis buffer. The gradient was generated using the following Accudenz concentration steps from bottom to top: 0.5 ml 60%, 1 ml 50%, 1 ml 43%, 1 ml 37%, 1 ml 31%, 1 ml 27%, 1 ml 23%, 1 ml 20%, 1 ml 17%, 1 ml 13%, and 1 ml 7%. Gradient analysis was performed on 15 OD600 equivalents of spheroplast lysate (300 g supernatant fraction) in a vol of 3 ml loaded on top of the gradient. The gradient was subjected to centrifugation at 4°C in an SW41 rotor (Beckman Instruments, Inc., Fullerton, CA) at 170,000 g for 18 h. 12 fractions were harvested manually from the top of the gradient, and proteins were TCA precipitated and analyzed by immunoblotting. mAbs to ALP were purchased from Molecular Probes, Inc. (Eugene, OR). Quantitation of proteins on gels was done by densitometry using NIH Image.
Construction of VAM3 and VPS33 Mutant Alleles
A temperature-conditional allele of VAM3 was constructed by PCR-based mutagenesis (Muhlrad et al., 1992). Primers complementary to chromosomal sequences immediately adjacent to the start and stop codons of VAM3 were used to amplify a 900-bp fragment (containing the entirety of the VAM3 ORF) under limiting dATP conditions (20 μM). A gapped plasmid was generated by digesting pVAM3.414 with BsmI and by isolating the vector by gel purification. The mutagenized PCR product and gapped plasmid were cotransformed into CJY1 cells, and transformants in which homologous recombination resulted in integration of mutagenized PCR product were selected by amino acid prototrophy. In much the same manner, a temperature-conditional allele of VPS33 was also constructed. Primers complementary to the sequence including both the start and stop codons of VPS33 were used to amplify a 2.1-kb PCR fragment under limiting dATP (20 μM) concentrations. Gapped plasmid was generated by digesting pVPS33.415 with AocI and SmaI and by isolating the vector by gel purification. The resulting PCR product was cotransformed with gapped plasmid into LBY317 harboring the pCYI50 plasmid, and transformants were selected for amino acid prototrophy. In both mutant selections, transformants were replica plated onto YPF, grown at 26°C overnight, and tested by colorimetric invertase assay (Horazdovsky et al., 1994) for temperature-conditional vps phenotype (CPY-invertase secretion) at 26°C and after 6 h of temperature shift to 38°C. Presumptive conditional mutant colonies were picked and retested, and plasmid linkage of the missorting phenotype was confirmed by retransformation of isolated plasmids into either TDY1 or LBY317 cells, respectively.
Fluorescence Microscopy and EM
To examine vacuolar morphology, [N-(3-triethylammoniumpropyl)-4-(p-diethylaminophenylhexatrienyl) pyridium dibromide] (FM4-64) labeling of yeast cell cultures was done as previously described (Vida and Emr, 1995), except the labeling was done at a concentration of 32 μM FM4-64 (Molecular Probes, Inc.) in YPD for 15 min at 30°C. For vacuolar inheritance assays, the time of labeling was increased to 45 min at 26°C and then cultures were chased for an additional hour at 26°C. Labeled cells were harvested, resuspended in fresh medium, and split into two equal aliquots that were further incubated at either 26°C or 38°C for 2 h. For conventional EM morphology studies, cells were grown at 26°C to mid-log phase. A portion of the cells were shifted to a nonpermissive temperature of 38°C for either 1 or 3 h. The cultures were processed for EM as previously described (Rieder et al., 1996). For the examination of autophagy by EM, cells were grown in YNB at 26°C to mid-log phase, harvested, washed once in SD-N medium, and resuspended at 1 OD600 per ml in SD-N medium to induce autophagy (Takeshige et al., 1992). The cultures were split and shifted to either 26°C or 38°C for 2.5 h and then processed for EM.
Results
Vam3p Is a Membrane-bound Protein That Localizes to the Vacuole
The sequence of Vam3p predicts a protein of 283 amino acids that, like other SNARE family members, has a transmembrane domain predicted at the carboxy terminus of the protein. To identify the VAM3 gene product, polyclonal antiserum was raised against a fusion protein consisting of the amino terminal 202 amino acids of Vam3p fused to GST. The resulting antiserum was affinity purified by binding to a GST–Vam3p-coupled cyanogen bromide column (Harlow and Lane, 1988). The affinity-purified antisera recognized a 35-kD protein from wild-type cell extracts by immunoblotting (Fig. (Fig.11 A, lane 2). The 35-kD species was not recognized by preimmune sera (data not shown) and was not present in vam3Δ cells (Fig. (Fig.11 A, lane 1). Cells expressing VAM3 from a 2μ overexpression plasmid showed a 15–20-fold increase in the abundance of the protein (Fig. (Fig.11 A, lane 3). The molecular mass of the protein was consistent with the 32-kD predicted molecular mass of Vam3p. Vam3p protein levels remained stable during a 60-min chase in cells treated with 20 μg/ml cycloheximide to inhibit new protein synthesis (data not shown). The relative abundance of Vam3p was ~20-fold less than CPY (>0.05% of cell protein), indicating that Vam3p is a fairly rare protein (data not shown).
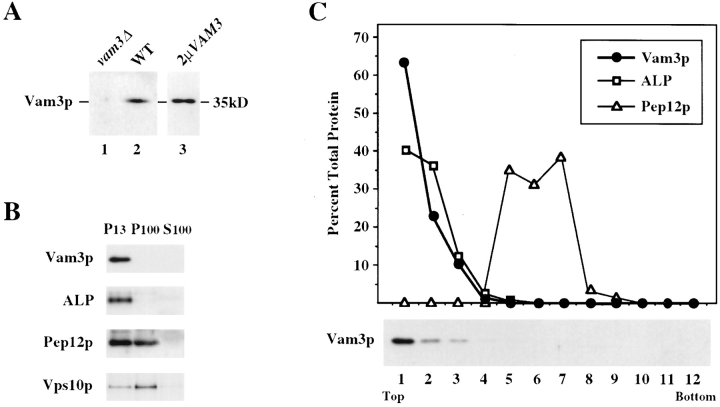
Localization of the VAM3 gene product. (A) SEY6210 (WT), TDY1 (vam3Δ), and SEY6210 cells harboring VAM3 on a multicopy (2μ) plasmid (pVAM3.424) were grown to exponential phase, harvested by centrifugation, and lysed. Total cellular proteins (0.5 OD600 equivalent per lane in lanes 1 and 2, and 0.1 OD600 equivalent in lane 3) were separated by SDS-PAGE and transferred to nitrocellulose. Vam3p was immunoblotted with affinity-purified polyclonal antibody and visualized by ECL fluorography. Note that the exposure time of lane 3 is approximately half that of lanes 1 and 2. (B) Wild-type (SEY6210) cells were labeled with [35S]cysteine/methionine at 30°C for 15 min and chased for an additional 45 min. Lysed spheroplasts were fractionated by differential centrifugation (as described in Materials and Methods) generating P13, P100, and S100 fractions. The fractions were divided into two aliquots. ALP and Vps10p were immunoprecipitated from the first set of fractions, resolved by SDS-PAGE, and visualized by autoradiography. Total proteins (0.5 OD600 equivalents per fraction) from the second set of fractions were subjected to SDS-PAGE, transferred to nitrocellulose, and immunoblotted with specific antiserum to Vam3p or Pep12p. Immunoblotted proteins were visualized by ECL fluorography. (C) A cleared lysate was generated from wild-type (SEY6210) cells, loaded at the top of an Accudenz step gradient, and centrifuged to equilibrium. Fractions were collected starting at the top of the gradient. Total proteins were precipitated from the fractions, separated by SDS-PAGE, and transferred to nitrocellulose. Vam3p, ALP, and Pep12p were detected by immunoblotting and visualized by ECL fluorography. The blot displaying the distribution of Vam3p is shown, and the distributions of Vam3p, ALP, and Pep12p are shown graphically.
Subcellular fractionation was performed to assess the intracellular location of Vam3p. Spheroplasts were prepared from wild-type cells and gently lysed by Dounce homogenization. The cleared cell lysate (300 g supernatant fraction) was subjected to centrifugation at 13,000 g to generate low speed pellet (P13) and supernatant (S13) fractions. The S13 fraction was then spun at 100,000 g to generate high speed pellet (P100) and supernatant (S100) fractions. As shown in Fig. Fig.11 B, Vam3p and the vacuolar protein ALP fractionated nearly identically, with the vast majority of each protein found in the P13 fraction. Consistent with previous observations, the endosomal t-SNARE Pep12p was found distributed between the P13 and P100 fractions (Becherer et al., 1996), and the majority of Vps10p, the vacuolar hydrolase sorting receptor that is enriched in late Golgi membranes, was observed in the P100 fraction (Marcusson et al., 1994).
Further support for vacuolar localization of Vam3p was provided by fractionation of cell lysates on an Accudenz equilibrium density gradient. Spheroplasts were prepared from wild-type cells and lysed as described above. The cleared lysate (300 g supernatant fraction) was loaded at the top of an Accudenz step gradient and centrifuged to equilibrium. Fractions were collected starting at the top of the gradient and analyzed for the presence of Vam3p, Pep12p, and ALP (Fig. (Fig.11 C). Vam3p colocalized with the majority of the vacuolar membrane protein ALP in the least dense region of the gradient (fractions 1–4). In contrast, the endosomal protein Pep12p migrated to a region of greater density (fractions 5–8) that was distinct from both the vacuolar marker ALP and Vam3p. In these gradients, protein markers of the Golgi, ER, mitochondria, and plasma membrane typically migrate into more dense regions of the gradient, also distinct from Vam3p and ALP (Singer-Kruger et al., 1993). Taken together, these data provide strong evidence that Vam3p is localized to vacuolar membranes.
VAM3 Null Mutants Exhibit Severe Vacuolar Protein Sorting and Morphology Defects
To determine the phenotypic consequences resulting from loss of Vam3p, deletion strains were constructed. Deletion constructs were generated by replacing ~75% of the VAM3 open reading frame with either the HIS3 or LEU2 genes (see Materials and Methods). Cells deleted for VAM3 were viable and grew at rates comparable to those of wild-type cells at elevated temperatures (38°C), indicating that VAM3 is not required for growth, even at elevated temperatures. Examination of vam3Δ cells by both FM4-64 vital staining and EM revealed that these mutants completely lack normal vacuolar structures. Instead, numerous electron-transparent compartments were present in almost all cells (data not shown). In addition, many cells contained two to five vacuole-like electron-dense structures, but these organelles were significantly smaller than wild-type vacuoles (see Figs. Figs.66 and and9).9). Accumulation of these novel electron-transparent membranous structures is characteristic of a subset of class B vps mutants, including vam3 (Wada et al., 1997) and vps41 (Cowles et al., 1997; Radisky et al., 1997), but not vps5 and vps17 (Kohrer and Emr, 1993; Horazdovsky et al., 1997).
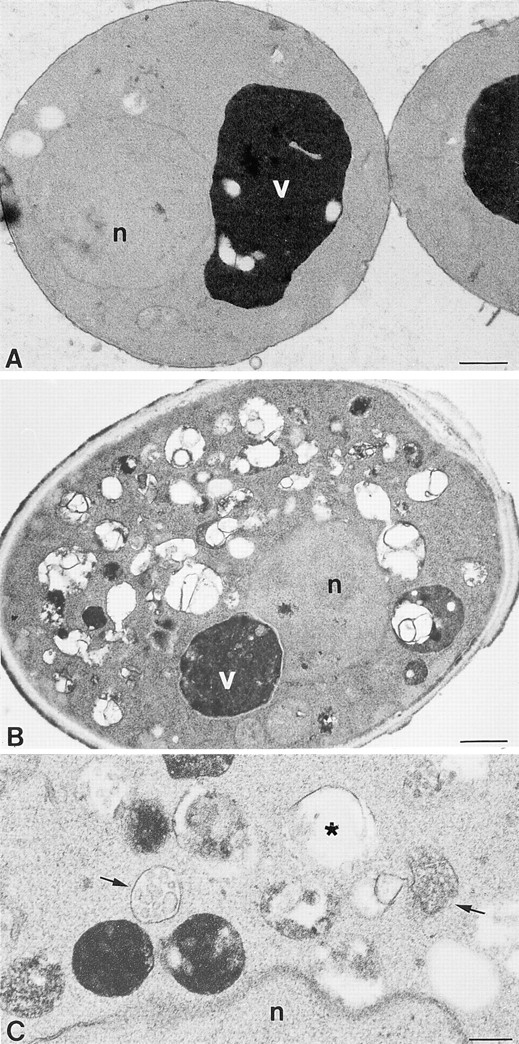
Ultrastructural analysis of vam3tsf mutants. (A) A cross-section of a typical vam3tsf (TDY1 + pVAM3-6.414) cell grown at 26°C, which closely resembles wild-type cells. (B) A cross-section of a vam3tsf cell after temperature shift to 38°C for 3 h. The accumulation of novel compartments is seen in addition to a prominent, electron-dense vacuolar compartment. (C) Enlarged examples of structures seen in vam3tsf cells grown at 38°C for 3 h. (Arrows) Multivesicular bodies; (asterisk) electron-transparent membrane compartment. n, nucleus; v, vacuole. Bars: (A and B) 500 nm; (C) 200 nm.
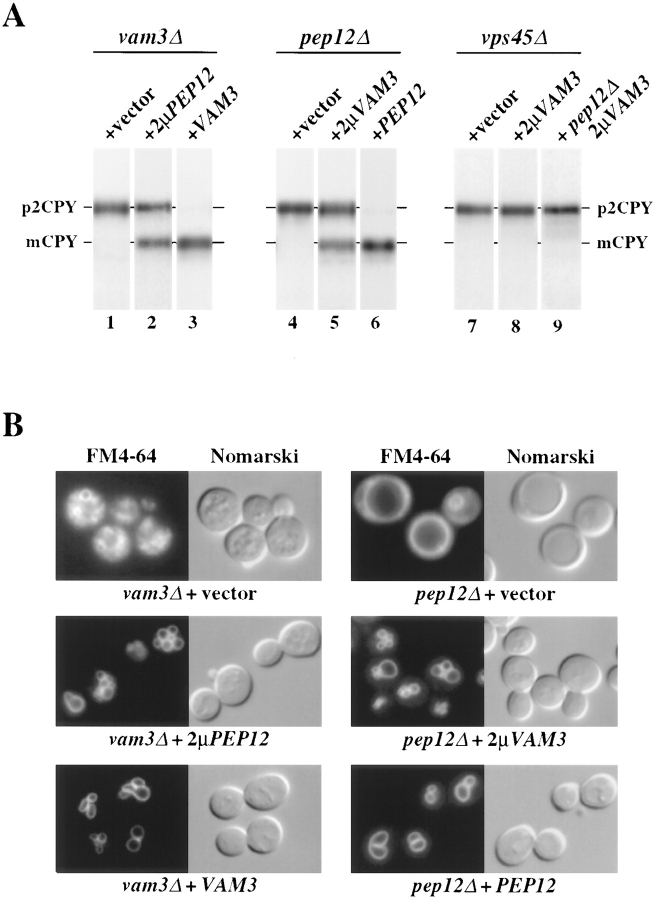
Suppression of vam3Δ and pep12Δ mutant cell phenotypes by overexpression of the reciprocal t-SNARE. (A) TDY1 (vam3Δ) cells containing either vector alone (pRS416), complementing plasmid (pVAM3.416), or PEP12 on a multicopy plasmid (pPEP12.426), and CBY31 (pep12Δ) cells carrying vector only (pRS416), complementing plasmid (pPEP12.416), or multicopy VAM3 plasmid (pVAM3.426) were converted to spheroplasts. CCY120 (vps45Δ) and CBY12 (vps45Δpep12Δ) containing either vector (pRS424) or VAM3 on a multicopy plasmid (pVAM3.424) were harvested as whole cells. All strains were incubated for 5 min at 30°C and then labeled with [35S]cysteine/methionine for 10 min. Chase was initiated by the addition of unlabeled cysteine and methionine and incubation was continued for 30 min. CPY was immunoprecipitated from lysates, resolved by SDS-PAGE, and visualized by autoradiography. Golgi-modified precursor (p2) and mature (m) forms of CPY are indicated. (B) TDY1 (vam3Δ) and CBY31 (pep12Δ) transformed with the identical plasmids as in A were grown at 30°C to exponential phase, harvested, and labeled with FM4-64 for 30 min at 30°C. Chase was initiated by the addition of prewarmed YPD medium and incubation was continued at 30°C for 1 h. Cells were then viewed by fluorescence and Nomarski microscopy.
The abnormal vacuolar morphology of vam3Δ cells suggested that both protein transport to the vacuole and vacuolar function may be compromised in these cells. Therefore, the ability of vam3Δ cells to properly transport newly synthesized resident vacuolar proteins was examined. Spheroplasts prepared from vam3Δ cells were pulse labeled for 10 min with [35S]cysteine/methionine to label newly synthesized proteins, and then were chased with unlabeled cysteine/methionine for 45 min. The spheroplasts were separated into intracellular and extracellular fractions. CPY was immunoprecipitated from the samples and analyzed by SDS-PAGE and autoradiography. The biosynthesis of vacuolar proteins such as CPY can be monitored by posttranslational modifications that correlate with transport through the secretory and vacuolar protein sorting pathways. In the case of CPY, p1CPY is generated as a result of core glycosyl modifications in the ER, which are then elongated in the Golgi complex to generate the slightly larger form, p2CPY. Upon delivery to the vacuole, the precursor CPY is cleaved to generate the mature active form of the enzyme, mCPY. As shown in Fig. Fig.2,2, wild-type cells properly delivered CPY to the vacuole as shown by the presence of mature CPY in the intracellular fraction (Fig. (Fig.2,2, lanes 1 and 2). In vam3Δ cells, CPY accumulated as the Golgi-modified p2 form, ~50% of which was secreted into the extracellular fraction (Fig. (Fig.2,2, lanes 3 and 4). A small portion (~10%) of the CPY that remained in the intracellular fraction was seen as an apparently misprocessed form that migrated as a species slightly smaller than p2CPY, which eventually was processed to mature CPY after prolonged chase (t1/2 > 60 min). The introduction of VAM3 on a single copy, centromere (CEN)–based plasmid complemented the CPY maturation defect of the vam3Δ mutant cells (Fig. (Fig.2,2, lanes 5 and 6).
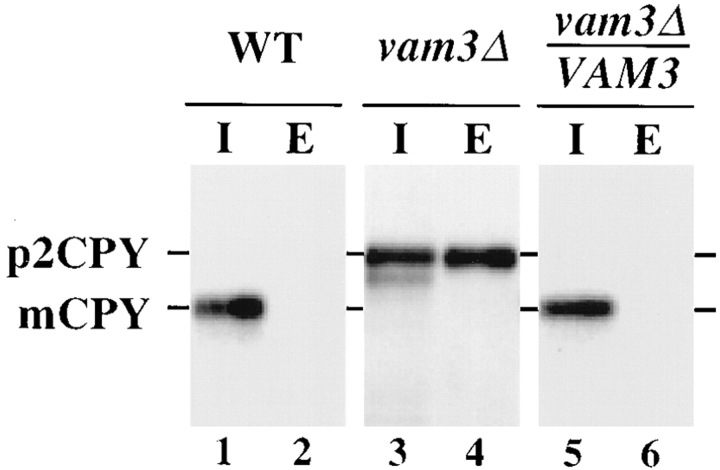
Vacuolar protein sorting in vam3 null cells. SEY6210 (WT), TDY1 (vam3Δ), and TDY1 cells harboring single-copy complementing VAM3 plasmid (pVAM3.414) were converted to spheroplasts, and then pulse labeled with [35S]cysteine/methionine for 10 min at 30°C. Chase medium containing nonradioactive cysteine and methionine was added and incubation was continued for an additional 45 min. The spheroplasts were separated into intracellular (I) and extracellular (E) fractions, and CPY was immunoprecipitated with specific polyclonal antibodies, resolved by SDS-PAGE, and analyzed by autoradiography. The positions of Golgi-modified precursor (p2) and mature (m) CPY are indicated.
A vam3tsf Mutant Is Defective for Transport of Multiple Vacuolar Proteins
Because of the dramatic effects on vacuolar morphology in vam3Δ cells, it is difficult to conclude whether the defects in protein sorting are a direct consequence of loss of Vam3p function, or due to secondary pleiotropic effects resulting from loss of vacuolar integrity in vam3Δ cells. To address the primary role of Vam3p, we generated a temperature-conditional allele of VAM3 by error prone PCR-mediated mutagenesis (Muhlrad et al., 1992; Stack et al., 1995). Approximately 6,000 transformants were screened for a vps phenotype (secretion of CPY–invertase fusion protein) at 26°C and after 6 h of temperature shift to 38°C. Several temperature-conditional alleles were obtained that secreted a substantial amount of CPY–invertase after a temperature shift to 38°C, but not at 26°C. One allele (vam3-6), which will be referred to here as vam3tsf, was chosen and characterized further.
If Vam3p is directly required for vacuolar protein transport, then inactivation of the protein (i.e., by temperature shift) would be expected to result in an immediate block in vacuolar protein processing. We tested vam3tsf cells for sorting of vacuolar hydrolases after a short incubation at nonpermissive temperature. Spheroplasts were prepared from both vam3tsf and wild-type cells. The cultures were split and half was incubated at 26°C while the other half was shifted to 38°C for 5 min. Each culture was then pulse labeled for 10 min with [35S]cysteine/methionine and chased with unlabeled cysteine/methionine for 45 min. Samples were harvested and separated into intracellular and extracellular fractions. Vacuolar proteins were immunoprecipitated with specific antibodies and analyzed by SDS-PAGE. As shown in Fig. Fig.3,3, at the permissive temperature of 26°C, vam3tsf cells matured CPY in a manner indistinguishable from wild-type cells (Fig. (Fig.3,3, lanes 1–4). However, at 38°C, a rapid block in the maturation of CPY was observed (Fig. (Fig.3,3, lanes 5 and 6). Approximately 60% of CPY accumulated as the Golgi-modified p2 precursor form. The remaining 40% of CPY was processed aberrantly and accumulated intracellularly. Less than 5% of the newly synthesized CPY was secreted to the extracellular media fraction. Similar results were observed for another soluble vacuolar hydrolase, PrA. We also examined the processing of two vacuolar membrane proteins, ALP and CPS. In vam3tsf cells at permissive temperature, the processing of both ALP and CPS occurred in a manner identical to wild-type cells (Fig. (Fig.3,3, lanes 1–4). However, in vam3tsf cells after a 5-min incubation at nonpermissive temperature, both proteins were completely blocked as the Golgi-modified precursor forms (Fig. (Fig.3,3, lanes 5 and 6). Vam3p thus appears to play an essential role in vacuolar protein transport of both soluble and integral membrane proteins.
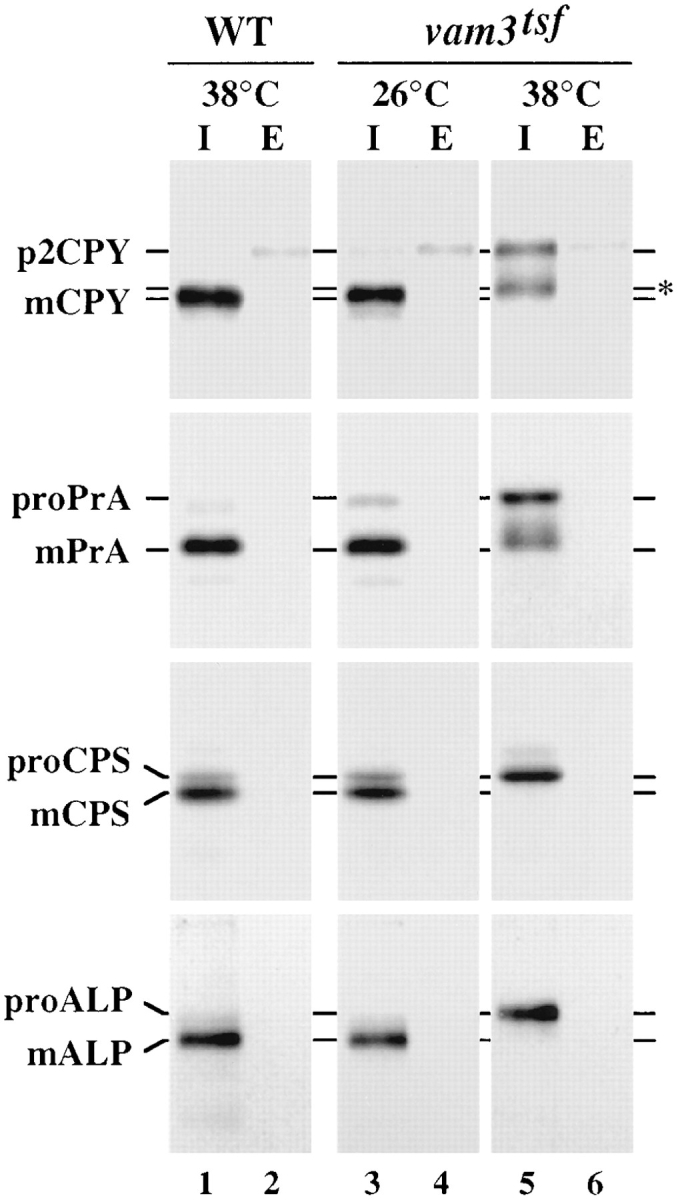
Vacuolar protein sorting in vam3tsf mutant cells. TDY1 (vam3Δ) cells transformed with either complementing plasmid (pVAM3.414) or plasmid containing a temperature-sensitive for function (tsf) allele of vam3 (pVAM3-6.414) were converted to spheroplasts, and then incubated at either permissive (26°C) or nonpermissive (38°C) temperature for 5 min. Cultures were labeled with [35S]cysteine/methionine for 10 min, and then chased for an additional 45 min at the indicated temperature. The cultures were separated into intracellular (I) and extracellular (E) fractions, and the vacuolar proteins CPY, PrA, CPS, and ALP were immunoprecipitated from each fraction, resolved by SDS-PAGE, and followed by autoradiography. CPS samples were treated with endoglycosidase H before electrophoresis. The positions of Golgi-modified precursor (p2, pro) and mature vacuolar (m) proteins are indicated.
Vam3p Function Is Required for Cytoplasm to Vacuole Delivery of API
Some newly synthesized vacuolar proteins do not reach the vacuole via the secretory pathway but instead follow a direct cytosol to vacuole delivery pathway (Klionsky et al., 1992). The precursor form of API is synthesized in the cytoplasm and transported directly into the vacuole where the amino-terminal precursor is cleaved, producing the mature form of the enzyme (Klionsky et al., 1992). Many mutants defective for the processing of API (cvt mutants) are also defective in autophagy (aut and apg mutants), indicating that delivery of API to the vacuole may be mediated by an autophagic mechanism (i.e., macroautophagy) (Harding et al., 1996; Scott et al., 1996). The processing of API was examined in vam3tsf cells to determine if Vam3p is required for delivery of API to the vacuole. Wild-type and vam3tsf cells were shifted to nonpermissive temperature for 5 min and then labeled for 10 min. Samples were harvested after 0, 30, 60, 90, and 120 min of chase. The maturation of API was analyzed by immunoprecipitation, SDS-PAGE, and autoradiography. As shown in Fig. Fig.4,4, cells containing the wild-type copy of VAM3 matured API with normal kinetics; essentially all API was processed to the mature vacuolar form after 120 min of chase. However, vam3tsf cells at nonpermissive temperature (38°C) accumulated newly synthesized API in its precursor form, with virtually no maturation of the protein, even after 120 min of chase. In vam3tsf cells at the permissive temperature of 26°C, API was also matured with kinetics comparable to that of wild-type cells (data not shown). The defect in API processing observed in vam3tsf cells at nonpermissive temperature indicates that Vam3p is directly required for the delivery of API to the vacuole.
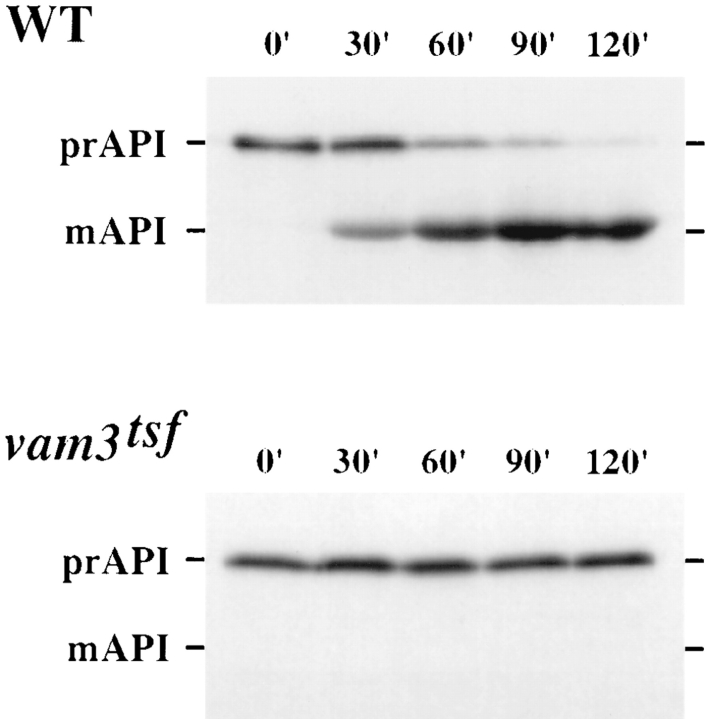
Analysis of API maturation in vam3tsf cells. Wild-type (SEY6210) cells and TDY1 (vam3Δ) cells carrying a vam3tsf plasmid (pVAM3-6.414) were incubated at 38°C for 5 min, labeled with [35S]cysteine/methionine for 10 min, and then chased for the indicated times. Equivalent volumes of labeled culture were harvested at the indicated time points of chase. API was recovered from lysates by immunoprecipitation, subjected to SDS-PAGE, and analyzed by autoradiography. The cytoplasmic precursor (pr) and mature vacuolar (m) forms of API are indicated.
Vam3p Is Required for the Docking and/or Fusion of Autophagosomes with the Vacuole
The block of API maturation in vam3tsf cells suggested that targeting of autophagosomes to the vacuole may be disrupted. Autophagosomes appear by EM as membrane-enclosed structures between 400 and 600 nm in diam (Baba et al., 1994). The compartments are primarily composed of cytoplasmic material, as they direct bulk uptake and vacuolar transport of cytoplasmic constituents, and thus stain with an electron density similar to the cytoplasm (Baba et al., 1994). To further examine possible autophagic defects associated with the loss of Vam3p function, we induced the formation of autophagic intermediates by nitrogen starvation and examined their uptake by the vacuole in both wild-type and vam3tsf cells. vam3tsf cells and complemented vam3Δ cells, both of which were also deleted for the PEP4 gene to prevent vacuolar degradation of autophagic bodies, were grown at 26°C to mid-log phase in YNB medium. The cells were then harvested and resuspended in SD-N. The cultures were split and incubated at either 26°C or 38°C for 2.5 h. Cells were harvested, fixed, and processed for EM. Wild-type cells starved at both 26°C and 38°C, as well as vam3tsf cells that were starved at 26°C, accumulated numerous autophagic bodies in the vacuoles (Fig. (Fig.55 A). In contrast, vam3tsf cells starved at 38°C for 2.5 h accumulated multiple autophagosomes in the cytoplasm, with no detectable accumulation of autophagic bodies in the vacuole (Fig. (Fig.55 B). The double membranes surrounding the cytoplasmic autophagosomes appear to be very fragile and easily disrupted as a result of preparation for EM. Thus, the perimeter of the autophagosomes was often seen as the electron-transparent space that the membrane previously occupied (Fig. (Fig.55 C). The temperature-conditional block of autophagosome delivery to the vacuole suggests that this process is dependent on the function of Vam3p.
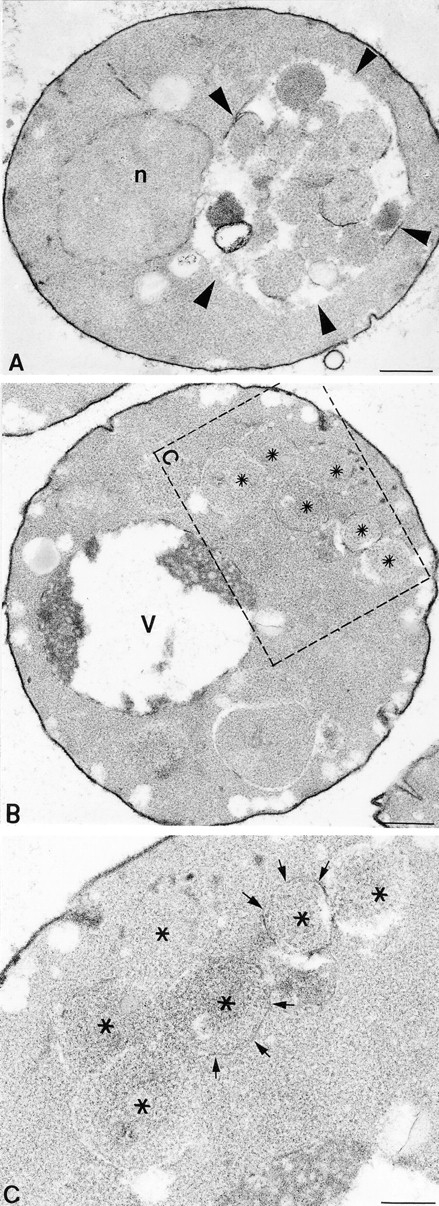
Examination of autophagy by EM. (A) Example of a pep4Δ cell (TDY10 harboring complementing VAM3 plasmid, pVAM3.414) after a 2.5-h induction of autophagy by nitrogen starvation at 38°C. (Arrowheads) The accumulation of autophagic bodies within the vacuole. (B) Cross-section of a vam3tsfpep4Δ cell (TDY10 cells harboring vam3tsf plasmid, pVAM3-6.414) after a 2.5-h induction of autophagy at a nonpermissive temperature of 38°C. An accumulation of autophagosomes in the cytoplasm (asterisks) is enclosed by the dashed box. (C) A region of the cell in B is enlarged in C, and arrows point to the membrane surrounding the autophagosomes. Fragments of the fragile limiting membrane are visible (arrows). n, nucleus; v, vacuole. Bars: (A and B) 500 nm; (C) 200 nm.
Morphological Analysis of vam3tsf Cells Reveals Accumulation of Aberrant Membrane Compartments
Due to the dramatic accumulation of novel membrane compartments in vam3Δ cells, we also were interested in the onset, severity, and characteristics of morphological defects associated with the vam3tsf allele. vam3tsf cells that had been grown at permissive temperature (26°C) or shifted to nonpermissive temperature (38°C) for either 1 or 3 h were characterized by EM. At 26°C, vam3tsf cells displayed typical wild-type morphology, containing prominent, intact, electron-dense vacuoles (Fig. (Fig.66 A). Although the majority of vam3tsf cells that had been shifted to 38°C for both 1 and 3 h maintained intact vacuoles, an accumulation of 300–500-nm novel membrane-enclosed compartments was also observed (Fig. (Fig.66 B). The accumulation of these aberrant compartments increased with respect to time of incubation at 38°C. The compartments were heterogeneous in both shape and content as indicated by electron-dense or -transparent staining (Fig. (Fig.6,6, B and C). In addition, vam3tsf cells at high temperature displayed an accumulation of multivesicular membrane compartments (Fig. (Fig.66 C). These data are consistent with a requirement for Vam3p in the docking/fusion of multiple transport intermediates with the vacuole.
Vacuolar Inheritance Is Unaffected in vam3tsf Cells
Since multiple fusion events with the vacuole are disrupted because of the inactivation of Vam3p, we were interested in investigating the effect of Vam3p on vacuolar inheritance, a process thought to involve homotypic vacuole–vacuole fusion (Conradt et al., 1992). Therefore, we examined the ability of vam3tsf cells to properly segregate vacuoles into the daughter bud. The vacuoles of both wild-type and vam3tsf cells were labeled with FM4-64 for 45 min at 26°C. The cells then were chased in YPD for 1 h at 26°C and split into two aliquots. One aliquot was maintained at 26°C and the other was shifted to 38°C for 2 h. Cells were harvested and viewed by fluorescence microscopy for the presence of vacuolar segregation structures. At either 26°C or 38°C, vam3tsf cells formed segregation structures in a manner indistinguishable from wild type; in both cases at least 96% of budding cells (n = 200 cells) had vacuoles in both the mother and daughter cells (Fig. (Fig.7).7). Furthermore, vam3tsf cells, even after 2 h at nonpermissive temperature, extended continuous segregation structures into the daughter bud and maintained intact vacuoles. Therefore, the function of Vam3p does not appear to be required for proper vacuolar segregation during mitosis.
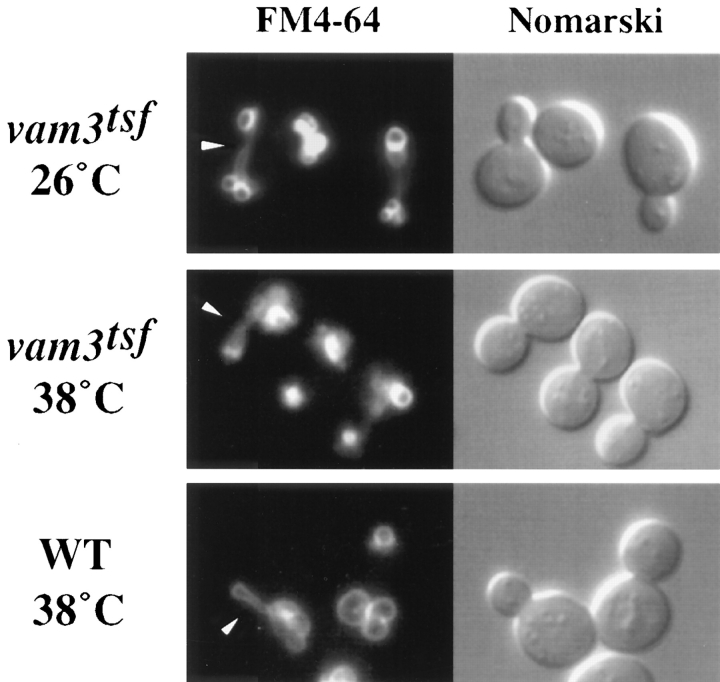
Vacuolar inheritance analysis of vam3tsf cells. TDY1 (vam3Δ) cells carrying either complementing VAM3 plasmid (pVAM3.414) or vam3tsf plasmid (pVAM3-6.414) were grown to exponential phase and then labeled with 32 μM FM4-64 for a period of 45 min. Cultures were chased with excess YPD for 1 h at 26°C and then split into equal aliquots. The aliquots were chased for an additional 2 h at either 26°C or 38°C. The cultures were then examined by fluorescence and Nomarski microscopy for the presence of vacuolar segregation structures. (White arrowheads) Segregation structures.
Vam3p Genetically Interacts with Vps33p, a Sec1p Homologue
Distinct protein complexes are thought to mediate vesicle targeting and fusion at discrete steps in the secretory and vacuolar protein sorting pathways. For example, members of the Sec1 family of proteins are thought to regulate vesicle targeting and fusion through specific interactions with t-SNARE proteins (Garcia et al., 1994; Pevsner et al., 1994). Thus, just as Pep12p has been shown to interact with the Sec1p homologue Vps45p to mediate protein sorting from the Golgi to the endosome (Burd et al., 1997), Vam3p is also likely to interact with regulatory proteins to execute its function. A strong candidate for such an interaction with Vam3p is Vps33p, a Sec1p homologue that may act at a late step in the vacuolar protein sorting pathway (Banta et al., 1990; Wada et al., 1990). Therefore, genetic interactions between VAM3 and VPS33 were examined. First, temperature-conditional alleles of VPS33 were generated by PCR-based mutagenesis in a manner similar to that described for VAM3 (see Materials and Methods). One temperature-conditional mutant (vps33-8), which will be referred here as vps33tsf, was chosen for further characterization. After a 5-min incubation at either permissive (26°C) or nonpermissive (38°C) temperature, vps33tsf cells were pulse labeled for 10 min and then chased for 30 min. After chase, the cells were spheroplasted and separated into intracellular and extracellular fractions. The processing of CPY was analyzed in each fraction by immunoprecipitation, SDS-PAGE, and autoradiography (Fig. (Fig.88 A). At 26°C, vps33tsf cells properly processed the majority of CPY to the mature vacuolar form, with only a minor (~10%) portion delayed intracellularly in the p2 form (Fig. (Fig.88 A, lanes 5 and 6). However, in vps33tsf cells after a 5-min incubation at 38°C, CPY was blocked intracellularly as the Golgi-modified p2 form of CPY. Similar to vam3tsf cells, only a small percentage (<5%) of p2CPY was secreted into the extracellular fraction and a small portion of the p2CPY was misprocessed (Fig. (Fig.88 A, lanes 7 and 8). Wild-type cells properly matured CPY at both temperatures (Fig. (Fig.88 A, lanes 1–4). A defect in the processing of the vacuolar membrane protein ALP also was observed, with a subtle processing defect at the permissive temperature and a complete block in ALP maturation at the nonpermissive temperature (data not shown). Thus, like vam3tsf cells, vps33tsf mutants exhibited a rapid defect in the transport of both CPY and ALP to the vacuole at nonpermissive temperature.
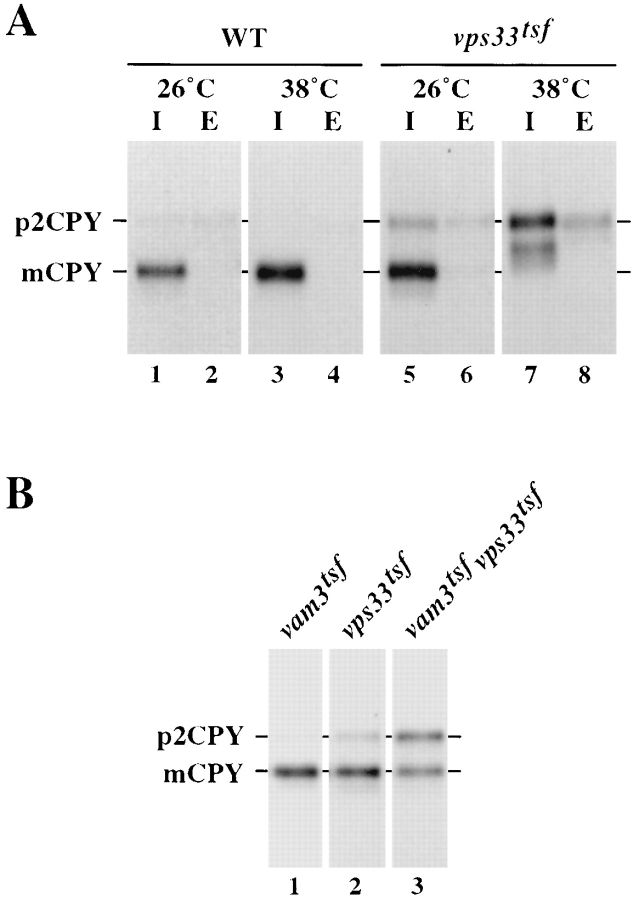
vps33tsfvam3tsf double mutant cells display synthetic vacuolar protein sorting defects. (A) LBY317 (vps33Δ) cells harboring complementing VPS33 plasmid (pVPS33.415) or vps33tsf plasmid (VPS33-8.415) were incubated at either 26°C or 38°C for 5 min, and then labeled with [35S]cysteine/methionine for 10 min. Chase was initiated by the addition of nonradioactive cysteine and methionine and incubation was continued for 30 min. Cells were spheroplasted and separated into intracellular (I) and extracellular (E) fractions. CPY was immunoprecipitated from each fraction, resolved by SDS-PAGE, and viewed by autoradiography. (B) vam3tsf (TDY7 + pVPS33.416 and pVAM3-6.414) and vps33t sf (pTDY7 + pVAM3.414 and pVPS33-8.416) single mutant cells, and vam3tsfvps33tsf (TDY7 + pVAM3-6.414 and pVPS33-8.416) double mutant cells were incubated at 26°C for 5 min, labeled with [35S]cysteine/methionine for 10 min, and chased for 30 min. CPY was recovered by immunoprecipitation, separated by SDS-PAGE, and followed by autoradiography. The positions of both Golgi-modified precursor (p2) and mature (m) CPY are indicated.
To determine whether Vam3p and Vps33p might functionally interact, we examined a vam3tsfvps33tsf double mutant for synthetic vacuolar protein sorting defects. A haploid double mutant strain was constructed and CPY maturation was assayed by metabolic labeling of whole cells. Cells were pulse labeled for 10 min and then chased for an additional 30 min at 26°C. After chase, the cells were lysed, and proteins were immunoprecipitated, separated on SDS-PAGE, and visualized by autoradiography (Fig. (Fig.88 B). Under these conditions, both vam3tsf and vps33tsf single mutant cells matured >90% of CPY (Fig. (Fig.88 B, lanes 1 and 2). However, in vam3tsfvps33tsf double mutant cells, a moderate synthetic sorting defect was observed; ~50% of newly synthesized CPY remained blocked in the p2 precursor form (Fig. (Fig.88 B, lane 3). The synthetic sorting defect of the double mutant strain is not as severe as that of the single mutant strains at nonpermissive temperature, indicating that transport to the vacuole is not completely disrupted in the double mutant cells. However, the increased sorting defect suggests that the two proteins function together to mediate protein sorting to the vacuole.
Pep12p and Vam3p Can Partially Substitute for One Another in the Vacuolar Protein Transport Pathway
Although Vam3p shares significant homology with many members of the syntaxin family, it is most similar to Pep12p, an endosomal t-SNARE. To address whether these SNARE proteins can functionally substitute for one another, we overexpressed each SNARE in the reciprocal null mutant background and examined the strains for improved transport of CPY to the vacuole. Spheroplasts were prepared from each strain, radiolabeled, and chased; proteins were then immunoprecipitated and analyzed by SDS-PAGE (Fig. (Fig.99 A). In both vam3Δ and pep12Δ cells, CPY accumulated as the Golgi-modified precursor form (Fig. (Fig.99 A, lanes 1 and 4). Introduction of CEN plasmids harboring VAM3 or PEP12 into vam3Δ or pep12Δ cells, respectively, complemented the CPY sorting defects of these mutants (Fig. (Fig.99 A, lanes 3 and 6). Interestingly, the overexpression of Pep12p in vam3Δ cells and Vam3p in pep12Δ cells resulted in significant conversion of CPY to the mature vacuolar form (Fig. (Fig.99 A, lanes 2 and 5). In addition to suppression of the CPY sorting defect, the ALP maturation defects were similarly suppressed (data not shown). To further determine the requirements of VAM3 suppression of pep12Δ cells, we examined the effect of VAM3 overexpression in vps45Δ and vps45Δpep12Δ cells. VPS45 gene encodes a member of the Sec1p family of proteins (Cowles et al., 1994; Piper et al., 1994) and is required, with Pep12p, for Golgi to endosome protein transport (Burd et al., 1997). Cultures of each strain were radiolabeled and chased, and proteins were immunoprecipitated and resolved by SDS-PAGE (Fig. (Fig.99 A). In both vps45Δ mutant cells and vps45Δpep12Δ double mutant cells, CPY was blocked in the Golgi-modified precursor form (Fig. (Fig.99 A, lanes 7 and 8). Overexpression of VAM3 was unable to suppress the CPY sorting defects of either mutant strain (Fig. (Fig.99 A, lanes 8 and 9). These results indicate that VAM3 requires VPS45 to facilitate CPY transport in pep12Δ cells. In a reciprocal experiment we found that overexpression of PEP12 does not suppress a vps33Δ mutant (data not shown).
Suppression of morphological defects associated with vam3Δ and pep12Δ mutant cells was also examined by vital staining of the vacuoles with FM4-64. As shown in Fig. Fig.99 B, wild-type cells typically contain several brightly staining vacuolar structures that corresponded to vacuolar depressions in Nomarski images (Vida and Emr, 1995). In contrast, vam3Δ cells displayed a punctate staining pattern throughout the cytoplasm. pep12Δ cells stained with FM4-64 displayed a single, large, vacuolar structure, characteristic of class D vps mutants (Vida and Emr, 1995). Overexpression of Pep12p dramatically suppressed the morphological defects of vam3Δ cells, resulting in several normal appearing vacuole structures. Similarly, pep12Δ cells that overproduced Vam3p contained near wild-type vacuolar structures. The introduction of VAM3 or PEP12 on low copy plasmids completely complemented the morphological defects of both the vam3Δ cells and pep12Δ cells, respectively. Overexpression of other presumptive yeast SNARE molecules (Sec22p, Bos1p, ORF #D8035.11p, ORF #YLR0936) or other class D proteins (Vps45p, Vac1p, Vps9p, Vps8p) did not improve vacuolar protein sorting of vam3Δ cells, indicating that the suppression by Pep12p is specific.
Discussion
We report on the primary function of Vam3p, a syntaxin homologue, in the docking and/or fusion of transport intermediates from multiple protein sorting pathways to the vacuole/lysosome. Subcellular fractionation and density gradient data indicated that Vam3p colocalized with vacuolar membranes. Conditional vam3tsf mutants exhibited a rapid defect in the transport of multiple resident vacuolar proteins after shifting to the nonpermissive temperature, indicating that Vam3p is a component of the vacuolar protein transport machinery. Additionally, autophagic pathway intermediates and API precursor accumulated in vam3tsf cells, indicating that Vam3p is also required for autophagy. Finally, EM analysis of these conditional mutants revealed a temperature-dependent accumulation of novel membrane structures, including multivesicular bodies, which may represent distinct transport intermediates destined for fusion with the vacuole. Thus, our data are most consistent with Vam3p residing on the vacuolar membrane where it functions as a multispecificity receptor required for docking and/or fusion of multiple prevacuolar transport intermediates.
Members of the syntaxin family of proteins are thought to act at specific intracellular organelles as receptors for the selective docking of appropriate transport intermediates. For instance, in neuronal cells, syntaxin is localized to the plasma membrane where it mediates the docking and fusion of secretory vesicles containing neurotransmitters (Bennett et al., 1992, 1993). In yeast, Sso1p and Sso2p, two redundant syntaxin homologues whose function is required for secretion, are localized to the plasma membrane (Aalto et al., 1993; Brennwald et al., 1994). Furthermore, Pep12p, an endosomal protein, is required for Golgi to endosome protein transport (Becherer et al., 1996; Burd et al., 1997). Thus, the membrane localization of such proteins is suggestive of their site of function. We have demonstrated through subcellular localization experiments that the majority of Vam3p resides in vacuolar membranes. It has also been reported recently that Vam3p is localized to vacuolar membranes by indirect immunofluorescence (Wada et al., 1997). Together, these data provide strong evidence that Vam3p resides on the vacuole, consistent with its function as a vacuolar t-SNARE.
Vam3p Functions as a Multispecificity t-SNARE
Our analysis of a temperature-conditional vam3 mutant (vam3tsf) suggests a direct involvement of Vam3p in a late step of protein transport to the vacuole. Multiple transport pathways to the vacuole were blocked even after a short incubation of vam3tsf cells at nonpermissive temperature. There are at least two pathways of biosynthetic traffic to the vacuole. CPY, PrA, and CPS transit through an endosomal compartment before final delivery to the vacuole. ALP is delivered by an alternate Golgi-to-vacuole transport pathway, possibly bypassing the endosomal compartment (Cowles et al., 1994, 1997; Stack et al., 1995; Burd et al., 1996, 1997; Horazdovsky et al., 1996). vam3tsf cells displayed immediate defects in the transport of CPY, PrA, CPS, and ALP, which is indicative of a direct involvement of Vam3p in both biosynthetic transport pathways.
Interestingly, the fate of accumulated p2CPY in vam3tsf cells suggests that Vam3p acts at a late step in the vacuolar protein sorting pathway. Defects in receptor-mediated transport of CPY from the Golgi to the endosome result in the rapid secretion of CPY (Cowles et al., 1994; Piper et al., 1994; Stack et al., 1995; Burd et al., 1997). Unlike these early acting conditional vps mutants (pep12tsf and vps45tsf mutants) that secrete p2CPY immediately upon inactivation, vam3tsf cells accumulated p2CPY intracellularly. The lack of CPY secretion in vam3tsf cells at nonpermissive temperature indicates that transport and recycling of the Vps10p hydrolase sorting receptor between the Golgi and the prevacuolar endosome is relatively unaffected and is thus consistent with a block in transport at a point beyond the endosomal intermediate. Additionally, the accumulated intracellular p2CPY in vam3tsf cells at nonpermissive temperature is slowly processed to aberrant forms. This processing is unlikely to be occurring in the vacuole. These cells have normal vacuoles populated with active processing proteases that would rapidly process p2CPY to its mature form. Similar aberrant processing of vacuolar protein precursors has been observed in other vps mutants that have defects in endosome to vacuole transport as a result of accumulation in a proteolytically competent endosomal compartment (Babst et al., 1997). Extended accumulation of CPY in a late prevacuolar compartment in vam3tsf cells results in slow maturation of p2CPY (t1/2 of 60 min). Consistent with these observations, at nonpermissive temperature, vam3tsf cells accumulated novel membrane-enclosed compartments that may represent these blocked transport intermediates.
The maturation of API, a protein delivered to the vacuole directly from the cytosol, possibly by an autophagic pathway (Klionsky et al., 1992), is also completely blocked at nonpermissive temperature in vam3tsf cells. Furthermore, the docking/fusion of large autophagosomes with the vacuole is disrupted in vam3tsf cells at nonpermissive temperature. Thus, the delivery of autophagic intermediates appears to require a functional vacuolar t-SNARE. If the mechanism of autophagic vesicle fusion uses conserved components such as SNAREs, autophagosomes may contain complementary components on their membranes that specifically target them to the vacuole via interactions with Vam3p.
The vam3 mutant was originally identified as a vacuolar morphology mutant (Wada et al., 1992). This phenotype is consistent with a primary role for Vam3p in maintenance of vacuolar morphology. However, in vam3tsf cells, the vacuoles remain stable for >3 h at nonpermissive temperature. Instead, vam3tsf cells accumulate novel membrane structures, which suggests that the primary defect associated with the loss of Vam3p function is impaired docking and/or fusion of transport intermediates with the vacuole. Thus, the complex morphology of vam3Δ cells appears to result from the accumulation of these transport intermediates, rather than instability and fragmentation of the vacuoles. Recent in vitro studies analyzing extracts from vam3Δ cells indicated that Vam3p is involved in homotypic vacuole–vacuole fusion (Nichols et al., 1997). While our data do not rule out a role for Vam3p in homotypic fusion, they suggest that these in vitro observations may correspond to heterotypic fusion of isolated prevacuolar intermediates from vam3Δ cells with wild-type vacuoles. Moreover, because we observed no obvious vacuolar inheritance defects in vam3tsf cells incubated for extended time at nonpermissive temperature, Vam3p function does not appear to play an essential role in vacuole–vacuole fusion during the inheritance process. Similar in vitro fusion experiments analyzing vacuoles isolated from vam3tsf cells may offer a means to determine the role, if any, of Vam3p in homotypic vacuole fusion reactions.
The accumulation of protein precursors and membrane-bound intermediate compartments that transit to the vacuole via distinct routes is consistent with a transport block at a site where these various pathways converge, the vacuole (Fig. (Fig.10).10). While we cannot rule out the possibility that Vam3p mediates the delivery of an unstable component, which in turn is required for the docking and fusion of other transport intermediates, this explanation seems unlikely because other vps mutants (i.e., pep12) do not show similar general transport defects (Burd et al., 1997; Cowles et al., 1997). Thus, analysis of protein transport and vacuolar morphology in vam3tsf cells is consistent with Vam3p acting as a multispecificity vacuolar t-SNARE that mediates the docking/fusion of multiple distinct late transport intermediates with the vacuole. The early Golgi t-SNARE, Sed5p, may also function as a multispecificity t-SNARE as it has been shown to function in both biosynthetic ER-to-Golgi and retrograde late-to-early Golgi transport (Hardwick and Pelham, 1992; Banfield et al., 1994, 1995).
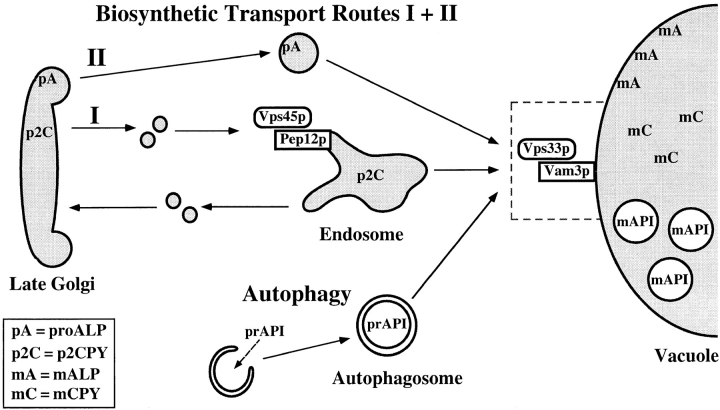
Vam3p acts as a receptor at the vacuole for the docking and fusion of multiple transport intermediates. Pep12p, the endosomal t-SNARE, functions together with Sec1p family member Vps45p in Golgi to endosome transport of p2CPY as well as other soluble vacuolar hydrolases. Our analyses of Vam3p indicate that it functions on the vacuole together with Vps33p in the docking and fusion of multiple transport intermediates. Proteins that are targeted to the vacuole through two separate biosynthetic pathways, as well as those that are targeted to the vacuole directly from the cytoplasm by autophagy, are shown. In the absence of functional Vam3p at the vacuole, the various transport intermediates are unable to fuse with the vacuole and accumulate as novel membrane compartments in the cytoplasm.
Interaction between Vam3p and Late Acting Vacuolar Transport Components
t-SNAREs are thought to act in conjunction with members of other well-conserved protein families (such as the v-SNARE, Sec1p, and Rab GTPase families) to execute their proposed function in targeting and fusion (for reviews see Rothman, 1994; Pfeffer, 1996). Thus, it is likely that Vam3p associates with accessory proteins in the same manner as other t-SNAREs. Mutations in VPS33 and YPT7, which encode Sec1p and Rab GTPase homologues, respectively, result in morphological and protein sorting defects similar to those observed in vam3 mutants (Banta et al., 1990; Wada et al., 1990; Wichmann et al., 1992), suggesting that they may mediate a common transport step. Although vps33 null alleles tend to exhibit more severe phenotypes than vam3 and ypt7 null mutants, these differences may be attributable to a more stringent requirement for Vps33p in late vacuolar protein transport. The observed genetic interaction between VAM3 and VPS33 indicates that these proteins do in fact function together to direct the docking and fusion of transport intermediates with the vacuole. These genetic data do not yet reveal the precise molecular mechanism of this interaction; however, several possibilities can be envisioned. For example, direct in vitro binding of neuronal Sec1 to syntaxin has been demonstrated in mammalian systems (Hata et al., 1993; Garcia et al., 1994; Pevsner et al., 1994). Thus, Vps33p may bind directly to Vam3p, and thereby regulate the function of Vam3p by either preventing the formation of inappropriate interactions or by facilitating the assembly of the correct components. Further studies investigating physical interactions between the Vps33p and Vam3p, as well as other protein factors that may regulate these interactions, such as Ypt7p, are in progress and should facilitate our understanding of the molecular mechanisms involved in this process.
Some insight into the function of syntaxin and Sec1p homologues can be gained by our observations that overexpression of Vam3p partially suppresses both the vacuolar protein sorting and morphological defects of a pep12Δ strain and, conversely, that overexpression of Pep12p results in partial suppression of the sorting and morphological defects associated with a vam3Δ strain. Apparently, increased cellular levels of these t-SNAREs results in redistribution of the excess protein to compartments other than their normal resident organelles, thus allowing them to function at inappropriate compartments. Indeed, overexpression of Pep12p results in a substantial pool of the protein redistributing to the vacuolar membrane (data not shown). Interestingly, overexpression of VAM3 and PEP12 was not capable of suppressing deletions of VPS45 and VPS33, respectively. Therefore, it is possible that under these conditions Pep12p and Vam3p may transiently interact with inappropriate components of the docking/fusion machinery to execute their function (i.e., Vam3p with Vps45p, and Pep12p with Vps33p). These observations indicate that SNARE molecules may not be the only specificity factors necessary for protein targeting. In fact, other studies indicate additional factors are required to direct docking and fusion of transport intermediates. For example, in mammalian cells, syntaxin is ubiquitously distributed throughout the neuronal plasma membrane, yet fusion occurs primarily at the active site of the nerve terminal, indicating that other factors are acting to direct fusion to this specific region (Bennett et al., 1992; Sollner et al., 1993; Garcia et al., 1995). Several candidate proteins that may be involved in this additional specificity function include members of the Sec1 and Rab protein families.
Studies examining the physical and genetic relationships between Vam3p and other transport components, as well as in vitro reconstitution of protein transport to the vacuole, are necessary to determine the mechanistic details of this final step of protein trafficking. Through these types of investigations, significant progress will be made in elucidating the molecular mechanisms of protein and membrane transport in yeast and other eukaryotic organisms.
Acknowledgments
This work was supported by grants GM32703 and CA58689 from the National Institutes of Health to S.D. Emr. S.D. Emr is an investigator of the Howard Hughes Medical Institute.
Abbreviations used in this paper
ALP | alkaline phosphatase |
API | aminopeptidase I |
CPS | carboxypeptidase S |
CPY | carboxypeptidase Y |
ECL | enhanced chemiluminescence |
FM4-64 | [N-(3-triethylammoniumpropyl)- 4-(p-diethylaminophenylhexatrienyl) pyridinum dibromide] |
GST | glutathione-S-transferase |
ORF | open reading frame |
PrA | proteinase A |
YPD | yeast extract/peptone/dextrose |
Footnotes
We are very grateful to Michael McCaffery and Tammie McQuistan for outstanding EM work (Electron Microscopy Core B, Program Project grant CA58689). We thank Yoh Wada and Dan Klionsky for helpful discussions and for generously providing plasmids and antisera. We also thank Colin Jamora for assistance with initial experiments, and members of the Emr laboratory, especially Erin Gaynor, Marcus Babst, and Chris Burd, for helpful comments and for critical reading of this manuscript.
Please address all correspondence to Scott D. Emr, Division of Cellular and Molecular Medicine and Department of Biology, Howard Hughes Medical Institute, University of California, San Diego, School of Medicine, La Jolla, CA 92093-0668. Tel.: (619) 534-6462. Fax: (619) 534-6414.
References
- Aalto MK, Ronne H, Keranen S. Yeast syntaxins Sso1p and Sso2p belong to a family of related membrane proteins that function in vesicular transport. EMBO (Eur Mol Biol Organ) J. 1993;12:4095–4104. [Europe PMC free article] [Abstract] [Google Scholar]
- Baba M, Takeshige K, Baba N, Ohsumi Y. Ultrastructural analysis of the autophagic process in yeast: detection of autophagosomes and their characterization. J Cell Biol. 1994;124:903–913. [Europe PMC free article] [Abstract] [Google Scholar]
- Babst M, Sato TK, Banta LM, Emr SE. Endosomal transport function in yeast requires a novel AAA-type ATPase, Vps4p. EMBO (Eur Mol Biol Organ) J. 1997;16:1820–1831. [Europe PMC free article] [Abstract] [Google Scholar]
- Banfield DK, Lewis MJ, Rabouille C, Warren G, Pelham HR. Localization of Sed5, a putative vesicle targeting molecule, to the cis-Golgi network involves both its transmembrane and cytoplasmic domains. J Cell Biol. 1994;127:357–371. [Europe PMC free article] [Abstract] [Google Scholar]
- Banfield DK, Lewis MJ, Pelham HR. A SNARE-like protein required for traffic through the Golgi complex. Nature (Lond) 1995;375:806–809. [Abstract] [Google Scholar]
- Bankaitis VA, Johnson LM, Emr SD. Isolation of yeast mutants defective in protein targeting to the vacuole. Proc Natl Acad Sci USA. 1986;83:9075–9079. [Europe PMC free article] [Abstract] [Google Scholar]
- Banta LM, Vida TA, Herman PK, Emr SD. Characterization of yeast Vps33p, a protein required for vacuolar protein sorting and vacuole biogenesis. Mol Cell Biol. 1990;10:4638–4649. [Europe PMC free article] [Abstract] [Google Scholar]
- Becherer KA, Rieder SE, Emr SD, Jones EW. Novel syntaxin homologue, Pep12p, required for the sorting of lumenal hydrolases to the lysosome-like vacuole in yeast. Mol Biol Cell. 1996;7:579–594. [Europe PMC free article] [Abstract] [Google Scholar]
- Bennett MK, Scheller RH. The molecular machinery for secretion is conserved from yeast to neurons. Proc Natl Acad Sci USA. 1993;90:2559–2563. [Europe PMC free article] [Abstract] [Google Scholar]
- Bennett MK, Calakos N, Scheller RH. Syntaxin: a synaptic protein implicated in docking of synaptic vesicles at presynaptic active zones. Science (Wash DC) 1992;257:255–259. [Abstract] [Google Scholar]
- Bennett MK, Garcia-Arraras JE, Elferink LA, Peterson K, Fleming AM, Hazuka CD, Scheller RH. The syntaxin family of vesicular transport receptors. Cell. 1993;74:863–873. [Abstract] [Google Scholar]
- Brennwald P, Kearns B, Champion K, Keranen S, Bankaitis V, Novick P. Sec9 is a SNAP-25-like component of a yeast SNARE complex that may be the effector of Sec4 function in exocytosis. Cell. 1994;79:245–258. [Abstract] [Google Scholar]
- Burd CG, Mustol PA, Schu PV, Emr SD. A yeast protein related to a mammalian Ras-binding protein, Vps9p, is required for localization of vacuolar proteins. Mol Cell Biol. 1996;16:2369–2377. [Europe PMC free article] [Abstract] [Google Scholar]
- Burd CG, Peterson M, Cowles CR, Emr SE. A novel Sec18p/ NSF-dependent complex required for Golgi to endosome transport in yeast. Mol Biol Cell. 1997;8:1089–1104. [Europe PMC free article] [Abstract] [Google Scholar]
- Conradt B, Shaw J, Vida T, Emr S, Wickner W. In vitro reactions of vacuole inheritance in Saccharomyces cerevisiae. . J Cell Biol. 1992;119:1469–1479. [Europe PMC free article] [Abstract] [Google Scholar]
- Cowles CR, Emr SD, Horazdovsky BF. Mutations in the VPS45 gene, a SEC1homologue, result in vacuolar protein sorting defects and accumulation of membrane vesicles. J Cell Sci. 1994;107:3449–3459. [Abstract] [Google Scholar]
- Cowles CR, Snyder WB, Burd CG, Emr SD. An alternative Golgi to vacuole delivery pathway in yeast: identification of a sorting determinant and required transport component. EMBO (Eur Mol Biol Organ) J. 1997;16:2769–2782. [Europe PMC free article] [Abstract] [Google Scholar]
- Ferro-Novick S, Jahn R. Vesicle fusion from yeast to man. Nature (Lond) 1994;370:191–193. [Abstract] [Google Scholar]
- Garcia EP, Gatti E, Butler M, Burton J, De Camilli P. A rat brain Sec1 homologue related to Rop and UNC18 interacts with syntaxin. Proc Natl Acad Sci USA. 1994;91:2003–2007. [Europe PMC free article] [Abstract] [Google Scholar]
- Garcia EP, McPherson PS, Chilcote TJ, Takei K, De Camilli P. rbSec1a and b colocalize with syntaxin 1 and SNAP-25 throughout the axon, but are not in a stable complex with syntaxin. J Cell Biol. 1995;129:105–120. [Europe PMC free article] [Abstract] [Google Scholar]
- Gaynor EC, te Heesen S, Graham TR, Aebi M, Emr SD. Signal-mediated retrieval of a membrane protein from the Golgi to the ER in yeast. J Cell Biol. 1994;127:653–665. [Europe PMC free article] [Abstract] [Google Scholar]
- Hanahan D. Studies on transformation of Escherichia coliwith plasmids. J Mol Biol. 1983;166:557–580. [Abstract] [Google Scholar]
- Harding TM, Hefner-Gravink A, Thumm M, Klionsky DJ. Genetic and phenotypic overlap between autophagy and the cytoplasm to vacuole protein targeting pathway. J Biol Chem. 1996;271:17621–17624. [Abstract] [Google Scholar]
- Hardwick KG, Pelham HR. SED5 encodes a 39-kD integral membrane protein required for vesicular transport between the ER and the Golgi complex. J Cell Biol. 1992;119:513–521. [Europe PMC free article] [Abstract] [Google Scholar]
- Harlow, E., and D.L. Lane. 1988. Antibodies: A Laboratory Manual. Cold Spring Harbor Laboratory, Cold Spring Harbor, NY.
- Hata Y, Slaughter CA, Sudhof TC. Synaptic vesicle fusion complex contains unc-18 homologue bound to syntaxin. Nature (Lond) 1993;366:347–351. [Abstract] [Google Scholar]
- Horazdovsky BF, Emr SD. The VPS16gene product associates with a sedimentable protein complex and is essential for vacuolar protein sorting in yeast. J Biol Chem. 1993;268:4953–4962. [Abstract] [Google Scholar]
- Horazdovsky BF, Busch GR, Emr SD. VPS21encodes a rab5-like GTP binding protein that is required for the sorting of yeast vacuolar proteins. EMBO (Eur Mol Biol Organ) J. 1994;13:1297–1309. [Europe PMC free article] [Abstract] [Google Scholar]
- Horazdovsky BF, Cowles CR, Mustol P, Holmes M, Emr SD. A novel RING finger protein, Vps8p, functionally interacts with the small GTPase, Vps21p, to facilitate soluble vacuolar protein localization. J Biol Chem. 1996;271:33607–33615. [Abstract] [Google Scholar]
- Horazdovsky, B.F., M.N.J. Seaman, S.A. McLaughlin, S.-H. Yoon, and S.D. Emr. 1997. Vps5p, a sorting nexin homologue, forms a complex with Vps17p and is required for vacuolar protein sorting. Mol. Biol. Cell. In press. [Europe PMC free article] [Abstract]
- Ito H, Fukuda Y, Murata K, Kimura A. Transformation of intact yeast cells treated with alkali cations. J Bacteriol. 1983;153:163–168. [Europe PMC free article] [Abstract] [Google Scholar]
- Johnson LM, Bankaitis VA, Emr SD. Distinct sequence determinants direct intracellular sorting and modification of a yeast vacuolar protease. Cell. 1987;48:875–885. [Abstract] [Google Scholar]
- Jones EW. Proteinase mutants of Saccharomyces cerevisiae. . Genetics. 1977;85:23–33. [Europe PMC free article] [Abstract] [Google Scholar]
- Klionsky DJ, Emr SD. Membrane protein sorting: biosynthesis, transport and processing of yeast vacuolar alkaline phosphatase. EMBO (Eur Mol Biol Organ) J. 1989;8:2241–2250. [Europe PMC free article] [Abstract] [Google Scholar]
- Klionsky DJ, Banta LM, Emr SD. Intracellular sorting and processing of a yeast vacuolar hydrolase; proteinase A propeptide contains vacuolar targeting information. Mol Cell Biol. 1988;8:2105–2116. [Europe PMC free article] [Abstract] [Google Scholar]
- Klionsky DJ, Herman PK, Emr SD. The fungal vacuole: composition, function, and biogenesis. Microbiol Rev. 1990;54:266–292. [Europe PMC free article] [Abstract] [Google Scholar]
- Klionsky DJ, Cueva R, Yaver DS. Aminopeptidase I of Saccharomyces cerevisiaeis localized to the vacuole independant of the secretory pathway. J Cell Biol. 1992;119:287–299. [Europe PMC free article] [Abstract] [Google Scholar]
- Kohrer K, Emr SD. The yeast VPS17 gene encodes a membrane associated protein required for the sorting of soluble vacuolar hydrolases. J Biol Chem. 1993;268:559–569. [Abstract] [Google Scholar]
- Maniatis, T., E.F. Fritsch, and J. Sambrook. 1982. Molecular Cloning: A Laboratory Manual. Cold Spring Harbor Laboratory, Cold Spring Harbor, NY.
- Marcusson EG, Horazdovsky BF, Cereghino JL, Gharakhanian E, Emr SD. The sorting receptor for yeast vacuolar carboxypeptidase Y is encoded by the VPS10 gene. Cell. 1994;77:579–586. [Abstract] [Google Scholar]
- Miller, J. 1972. Experiments in Molecular Genetics. Cold Spring Harbor Laboratory, Cold Spring Harbor, NY.
- Muhlrad D, Hunter R, Parker R. A rapid method for localized mutagenesis of yeast genes. Yeast. 1992;8:79–82. [Abstract] [Google Scholar]
- Nichols BJ, Ungermann C, Pelham HRB, Wickner WT, Haas A. Homotypic vacuolar fusion mediated by t- and v- SNAREs. Nature (Lond) 1997;387:199–202. [Abstract] [Google Scholar]
- Novick P, Brennwald P. Friends and family: the role of Rab GTPases in vesicular traffic. Cell. 1993;75:597–601. [Abstract] [Google Scholar]
- Paravicini G, Horazdovsky BF, Emr SD. Alternative pathways for the sorting of soluble vacuolar proteins in yeast: a vps35null mutant missorts and secretes only a subset of vacuolar hydrolases. Mol Biol Cell. 1992;3:415–427. [Europe PMC free article] [Abstract] [Google Scholar]
- Pevsner J, Hsu SC, Scheller RH. n-Sec1: a neural-specific syntaxin-binding protein. Proc Natl Acad Sci USA. 1994;91:1445–1449. [Europe PMC free article] [Abstract] [Google Scholar]
- Pfeffer SR. Transport vesicle docking: SNAREs and associates. Annu Rev Cell Dev Biol. 1996;12:441–461. [Abstract] [Google Scholar]
- Piper RC, Whitters EA, Stevens TH. Yeast Vps45p is a Sec1p-like protein required for the consumption of vacuole-targeted, post-Golgi transport vesicles. Eur J Cell Biol. 1994;65:305–318. [Abstract] [Google Scholar]
- Radisky DC, Snyder WB, Emr SE, Kaplan J. Identification of VPS41, a gene required for vacuolar trafficking and the assembly of the yeast high affinity iron transport system. Proc Natl Acad Sci USA. 1997;94:5662–5666. [Europe PMC free article] [Abstract] [Google Scholar]
- Rieder SE, Banta LM, Kohrer K, McCaffery JM, Emr SD. Multilamellar endosome-like compartment accumulates in the yeast vps28. Mol Biol Cell. 1996;7:985–999. [Europe PMC free article] [Abstract] [Google Scholar]
- Robinson JS, Klionsky DJ, Banta LM, Emr SD. Protein sorting in Saccharomyces cerevisiae: isolation of mutants defective in the delivery and processing of multiple vacuolar hydrolases. Mol Cell Biol. 1988;8:4936–4948. [Europe PMC free article] [Abstract] [Google Scholar]
- Rothman JE. Mechanisms of intracellular protein transport. Nature (Lond) 1994;372:55–63. [Abstract] [Google Scholar]
- Rothman JH, Stevens TH. Protein sorting in yeast: mutants defective in vacuole biogenesis mislocalize vacuolar proteins into the late secretory pathway. Cell. 1986;47:1041–1051. [Abstract] [Google Scholar]
- Rothman JH, Howald I, Stevens TH. Characterization of genes required for protein sorting and vacuolar function in the yeast Saccharomyces cerevisiae. . EMBO (Eur Mol Biol Organ) J. 1989;8:2057–2065. [Europe PMC free article] [Abstract] [Google Scholar]
- Scott SV, Hefner-Gravink A, Morano KA, Noda T, Ohsumi Y, Klionsky DJ. Cytoplasm-to-vacuole targeting and autophagy employ the same machinery to deliver proteins to the yeast vacuole. Proc Natl Acad Sci USA. 1996;93:12304–12308. [Europe PMC free article] [Abstract] [Google Scholar]
- Sherman, F., G.R. Fink, and L.W. Lawrence. 1979. Methods in Yeast Genetics: a Laboratory Manual. Cold Spring Harbor Laboratory, Cold Spring Harbor, NY.
- Sikorski RS, Hieter P. A system of shuttle vectors and yeast host strains designed for efficient manipulation of DNA in Saccharomyces cerevisiae. . Genetics. 1989;122:19–27. [Europe PMC free article] [Abstract] [Google Scholar]
- Singer-Kruger B, Frank R, Crausaz F, Riezman H. Partial purification and characterization of early and late endosomes from yeast. J Biol Chem. 1993;268:14376–14386. [Abstract] [Google Scholar]
- Singer-Kruger B, Stenmark H, Dusterhoft A, Philippsen P, Yoo JS, Gallwitz D, Zerial M. Role of three rab5 like GTPases, Ypt51p, Ypt52p, and Ypt53p, in the endocytic and vacuolar protein sorting pathways of yeast. J Cell Biol. 1994;125:283–298. [Europe PMC free article] [Abstract] [Google Scholar]
- Sollner T, Whiteheart SW, Brunner M, Erdjument-Bromage H, Geromanos S, Tempst P, Rothman JE. SNAP receptors implicated in vesicle targeting and fusion. Nature (Lond) 1993;362:318–324. [Abstract] [Google Scholar]
- Stack JH, DeWald DB, Takegawa K, Emr SD. Vesicle-mediated protein transport: regulatory interactions between the Vps15 protein kinase and the Vps34 PtdIns 3-kinase essential for protein sorting to the vacuole in yeast. J Cell Biol. 1995;129:321–334. [Europe PMC free article] [Abstract] [Google Scholar]
- Takeshige K, Baba M, Tsuboi S, Noda T, Ohsumi Y. Autophagy in yeast demonstrated with proteinase-deficient mutants and conditions for its induction. J Cell Biol. 1992;119:301–311. [Europe PMC free article] [Abstract] [Google Scholar]
- Vida TA, Emr SD. A new vital stain for visualizing vacuolar membrane dynamics and endocytosis in yeast. J Cell Biol. 1995;128:779–792. [Europe PMC free article] [Abstract] [Google Scholar]
- Wada Y, Kitamoto K, Kanbe T, Tanaka K, Anraku Y. The SLP1 gene of Saccharomyces cerevisiaeis essential for vacuolar morphogenesis and function. Mol Cell Biol. 1990;10:2214–2223. [Europe PMC free article] [Abstract] [Google Scholar]
- Wada Y, Ohsumi Y, Anraku Y. Genes for directing vacuolar morphogenesis in Saccharomyces cerevisiae. . J Biol Chem. 1992;267:18665–18670. [Abstract] [Google Scholar]
- Wada Y, Nakamura N, Ohsumi Y, Hirata A. Vam3p, a new member of syntaxin related proteins, is required for vacuolar assembly in the yeast Saccharomyces cerevisiae. . J Cell Sci. 1997;110:1299–1306. [Abstract] [Google Scholar]
- Wichmann H, Hengst L, Gallwitz D. Endocytosis in yeast: evidence for the involvement of a small GTP binding protein (Ypt7p) Cell. 1992;71:1131–1142. [Abstract] [Google Scholar]
Articles from The Journal of Cell Biology are provided here courtesy of The Rockefeller University Press
Full text links
Read article at publisher's site: https://doi.org/10.1083/jcb.138.3.517
Read article for free, from open access legal sources, via Unpaywall:
http://jcb.rupress.org/content/138/3/517.full.pdf
Citations & impact
Impact metrics
Citations of article over time
Smart citations by scite.ai
Explore citation contexts and check if this article has been
supported or disputed.
https://scite.ai/reports/10.1083/jcb.138.3.517
Article citations
Intracellular trafficking of HIV-1 Gag via Syntaxin 6-positive compartments/vesicles: Involvement in tumor necrosis factor secretion.
J Biol Chem, 300(3):105687, 26 Jan 2024
Cited by: 0 articles | PMID: 38280430 | PMCID: PMC10891346
The dynamin Vps1 mediates Atg9 transport to the sites of autophagosome formation.
J Biol Chem, 299(5):104712, 14 Apr 2023
Cited by: 3 articles | PMID: 37060997 | PMCID: PMC10196871
SNARE Proteins in Synaptic Vesicle Fusion.
Adv Neurobiol, 33:63-118, 01 Jan 2023
Cited by: 3 articles | PMID: 37615864
VAMP724 and VAMP726 are involved in autophagosome formation in Arabidopsis thaliana.
Autophagy, 19(5):1406-1423, 13 Oct 2022
Cited by: 6 articles | PMID: 36130166 | PMCID: PMC10240985
The entry of unclosed autophagosomes into vacuoles and its physiological relevance.
PLoS Genet, 18(10):e1010431, 13 Oct 2022
Cited by: 4 articles | PMID: 36227834 | PMCID: PMC9562215
Go to all (224) article citations
Data
Similar Articles
To arrive at the top five similar articles we use a word-weighted algorithm to compare words from the Title and Abstract of each citation.
Vam7p, a SNAP-25-like molecule, and Vam3p, a syntaxin homolog, function together in yeast vacuolar protein trafficking.
Mol Cell Biol, 18(9):5308-5319, 01 Sep 1998
Cited by: 130 articles | PMID: 9710615 | PMCID: PMC109116
The Saccharomyces cerevisiae v-SNARE Vti1p is required for multiple membrane transport pathways to the vacuole.
Mol Biol Cell, 10(6):1719-1732, 01 Jun 1999
Cited by: 100 articles | PMID: 10359592 | PMCID: PMC25363
Acidic di-leucine motif essential for AP-3-dependent sorting and restriction of the functional specificity of the Vam3p vacuolar t-SNARE.
J Cell Biol, 142(4):913-922, 01 Aug 1998
Cited by: 83 articles | PMID: 9722605 | PMCID: PMC2132875
Multiple sorting pathways between the late Golgi and the vacuole in yeast.
Biochim Biophys Acta, 1404(1-2):211-230, 01 Aug 1998
Cited by: 121 articles | PMID: 9714809
Review
Funding
Funders who supported this work.
NCI NIH HHS (1)
Grant ID: CA58689
NIGMS NIH HHS (1)
Grant ID: GM32703