Abstract
Free full text

Loss of talin1 in platelets abrogates integrin activation, platelet aggregation, and thrombus formation in vitro and in vivo
Abstract
Platelet adhesion and aggregation at sites of vascular injury are essential for normal hemostasis but may also lead to pathological thrombus formation, causing diseases such as myocardial infarction or stroke. Heterodimeric receptors of the integrin family play a central role in the adhesion and aggregation of platelets. In resting platelets, integrins exhibit a low affinity state for their ligands, and they shift to a high affinity state at sites of vascular injury. It has been proposed that direct binding of the cytoskeletal protein talin1 to the cytoplasmic domain of the integrin β subunits is necessary and sufficient to trigger the activation of integrins to this high affinity state, but direct in vivo evidence in support of this hypothesis is still lacking. Here, we show that platelets from mice lacking talin1 are unable to activate integrins in response to all known major platelet agonists while other cellular functions are still preserved. As a consequence, mice with talin-deficient platelets display a severe hemostatic defect and are completely resistant to arterial thrombosis. Collectively, these experiments demonstrate that talin is required for inside-out activation of platelet integrins in hemostasis and thrombosis.
Damage to the integrity of the vessel wall results in exposure of the subendothelial extracellular matrix, which triggers adhesion and aggregation of platelets (1, 2). The consequence of this process is the formation of a thrombus that prevents blood loss at sites of injury or leads to occlusion and irreversible tissue damage or infarction in diseased vessels. Integrins play a central role in adhesion and aggregation of platelets (3). Integrins are heterodimeric transmembrane receptors composed of an α and a β subunit that are expressed in a low affinity state in resting platelets. After activation, mediated by other platelet receptors, integrins shift to a high affinity state and efficiently bind their ligands (3).
Cellular control of integrin activation, which is crucial for numerous biological processes such as cell adhesion, motility, differentiation, and apoptosis (4–8), requires transmission of a signal from the small cytoplasmic tails to the large extracellular domains (9). Most of the seminal work on integrin activation was performed on αIIbβ3 integrin, which is the principal integrin expressed on platelets and whose major ligands are fibrinogen, fibronectin, and von Willebrand factor. The affinity of this integrin for ligands is highly modulatable, and upon activation, it mediates platelet adhesion, aggregation, and spreading on the exposed extracellular matrix of injured vessel walls (3) as well as pathological thrombus formation (1). Because of the importance of αIIbβ3 integrin in platelet aggregation, it has become an attractive pharmacological target for the prevention of ischemic cardiovascular events. Strategies to inhibit its function include antibodies (abciximab), cyclic peptides adapted from a snake venom disintegrin (eptifibitide), and nonpeptide analogues of an RGD [Arg-Gly-Asp] peptide (tirofiban and lamifiban) that inhibit ligand binding (10). Although these inhibitors are beneficial for patients undergoing percutaneous coronary intervention, they do not have widespread clinical use because of their side effect of unwanted bleeding (10). Therefore, considerable effort has been made to identify the molecular mechanisms that regulate αIIbβ3 activation to identify potential new targets to inhibit platelet aggregation.
At sites of vascular injury, platelet activation is triggered by exposed subendothelial collagens, thromboxane A2 and ADP released from activated platelets, and thrombin generated by the coagulation cascade (2, 11). Although these different agonists activate different signaling pathways, it has been proposed that the final common step for αIIbβ3 integrin activation is the activation and binding of the cytoskeletal protein talin1 to integrin β tails (12).
Talin1 is an ~270-kD (13) elongated dimeric actin-binding protein and is the only talin isoform expressed in hematopoietic cells (14). Talin1 colocalizes with activated integrins, is a component of focal adhesions, and links integrins with the actin cytoskeleton (15, 16). The best evidence for the requirement of talin for integrin αIIbβ3 activation came from studies with talin1-depleted, mouse embryonic stem cell–derived megakaryocytes, which revealed that different agonists such as ADP or thrombin were unable to induce αIIbβ3 activation (17). Another study demonstrated that mice expressing the L746A mutation of β3 integrin, which is believed to be unable to bind talin, display impaired inside-out activation of αIIbβ3 resulting in protection from thrombosis and a relatively mild bleeding phenotype (18). Although these observations suggest an essential role for talin1 in the activation of αIIbβ3 in platelets, direct in vivo evidence for this hypothesis is still lacking.
RESULTS AND DISCUSSION
We previously showed that disruption of the talin1 (Tln1) gene in mice results in embryonic lethality around gastrulation excluding the usage of Tln1-null animals for studies on platelet function (19). Therefore, we generated mice carrying a Tln1 gene flanked by loxP sites (Tln1 fl; Fig. 1 A) and crossed them with transgenic mice expressing the Cre recombinase under the control of the inducible interferon-sensitive Mx promoter (20) (Tln1 fl/fl/Cre+; referred to as Tln1−/− mice) to induce efficient and permanent Tln1 gene deletion in all hematopoietic cells including megakaryocytes by intraperitoneal injection of polyinosinic-polycytidylic acid (pI-pC) (21).Tln1 fl/fl mice were used as controls and treated identically. 10 d after the last injection, the efficiency of talin deletion was confirmed with Western blots from platelet lysates also showing that the expression levels of other proteins, including filamin A, integrin linked kinase, β1 integrin, c-src, and actin, were unaltered (Fig. 1 B).
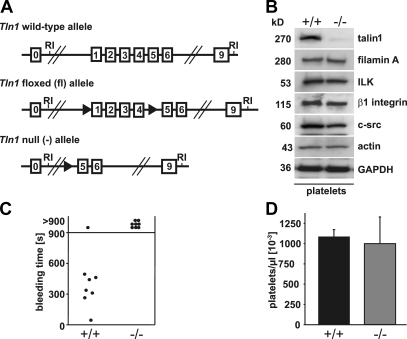
Prolonged bleeding times in Tln−/− mice. (A) Scheme of the targeted alleles. In the conditional allele (fl) exons 1–4 are flanked with loxP sites. Exposure of the conditional (fl) allele to Cre results in the removal of the Tln1 floxed region (exons 1–4) and generates the deleted allele (−). RI, EcoRI. (B) Western blot analyses of platelet lysates from control Tln1 (+/+) and Tln1−/− (−/−) mice. (C) Tail bleeding times in wild-type and Tln1−/− chimeras. (D) Peripheral platelet counts in control and Tln1−/− mice.
To investigate the consequence of talin deficiency on hemostasis, we performed tail bleeding experiments. To restrict the Cre-induced deletion of the Tln1 gene to the hematopoietic system, bone marrow derived from either Tln1 fl/fl/Cre+ or Tln1 fl/fl mice was transferred into irradiated normal recipient mice. 3 wk after transfer, the Tln1 gene was deleted by pI-pC injections and the functional absence of the protein in circulating platelets was confirmed by flow cytometry (not depicted). Although control chimeras arrested bleeding within 7.3 ± 2.2 min, all of the Tln1−/− chimeras bled longer than 15 min (Fig. 1 C). Thus, talin is essential to halt bleeding upon vessel injury.
To test whether the hemostatic disorder was due to defective platelet formation, we assessed platelet counts, which were similar to controls (Fig. 1 D). Flow cytometric analysis demonstrated normal expression levels of prominent surface receptors in mutant platelets, except for αIIbβ3 integrin, which was reduced by ~15% (Table I). Forward scatter/side scatter characteristics were the same as control, suggesting normal size and shape of the mutant platelets. Thus, talin is not required for megakaryocyte differentiation and platelet production but regulates the expression of some platelet surface receptors.
Table I.
Glycoprotein expression on Tln1−/− platelets
Glycoprotein | Wild-type | Tln1−/− |
---|---|---|
Integrin αIIbβ3 | 561.3 ± 24.1 | 476.0 ± 22.6 |
Integrin α2 | 128.5 ± 6.2 | 123.3 ± 2.3 |
Integrin β1 | 141.0 ± 13.5 | 135.0 ± 13.9 |
Integrin α5 | 40.8 ± 2.9 | 38.0 ± 2.6 |
GPIbα | 508 ± 23.5 | 476.3 ± 9.1 |
GPIX | 544.5 ± 27.6 | 590.3 ± 14.2 |
GPV | 336.8 ± 9.5 | 337.0 ± 9.6 |
GPVI | 81.3 ± 2.5 | 72.0 ± 4.4 |
CD9 | 1528.8 ± 52.0 | 1544.0 ± 88.1 |
The data shown are mean ± SD fluorescence intensities of six mice per group.
To determine whether the severe hemostatic defect is caused by impaired inside-out activation of αIIbβ3, we induced platelet aggregation using different agonists. As shown in Fig. 2 A, Tln1−/− platelets failed to aggregate in response to high concentrations of thrombin, ADP, the stable thromboxane A2 analogue U46619, collagen, and the GPVI-specific agonist collagen-related peptide (CRP). Interestingly, all agonists induced a comparable activation-dependent change from discoid to spherical shape in control and Tln1−/− platelets, which can be seen in aggregometry as a short decrease in light transmission after the addition of agonists. This suggests a selective defect in αIIbβ3-dependent aggregation rather than a general impairment of activation of signaling pathways in Tln1−/− platelets.
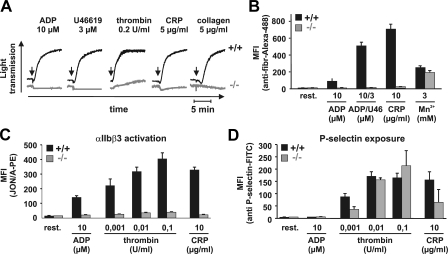
Impaired platelet function in Tln1−/− mice. (A) Platelet aggregation assay reveals impaired aggregation of Tln1−/− platelets (gray lines) in response to ADP, U46619, thrombin, CRP, and collagen when compared with control platelets (black lines). Arrows denote the addition of agonist. (B) Wild-type (black bars), but not Tln1−/− platelets (gray bars), bind fibrinogen in response to 10 μM ADP, 10 μM ADP plus 3 μM U46619, or 10 μg/ml CRP. Treatment with 3 mM MnCl2 triggers comparable binding. (C and D) Tln1−/− platelets (gray bars) reveal a complete block in activation of αIIbβ3 after stimulation with 10 μM ADP, 10 μg/ml CRP, and different thrombin concentrations (0.001–0.1 U/ml), whereas platelet degranulation measured by the surface expression of P-selectin is not or mildly affected. Wild-type platelets (black bars) were used as a control. MFI, mean fluorescence intensity.
To assess integrin αIIbβ3 function more directly, we measured agonist-induced binding of Alexa Fluor 488–tagged fibrinogen by flow cytometry. Control platelets bound fibrinogen, which was inhibited by an αIIbβ3-blocking antibody (not depicted), in response to all agonists tested, whereas Tln1−/− platelets were unable to bind fibrinogen (Fig. 2 B). This defect was due to impaired inside-out activation of αIIbβ3 as Mn2+, known to exogenously activate β1 and β3 integrins (22, 23), induced fibrinogen binding in both control and Tln1−/− platelets (Fig. 2 B). This binding was 24.1% lower in the mutant platelets as compared with the control (P < 0.01), which corresponds well to the reduced surface expression of αIIbβ3 in those cells (Table I). Similar results to those obtained with fibrinogen were seen with the JON/A-PE antibody, which selectively binds to activated αIIbβ3 integrins on mouse platelets (Fig. 2 C) (24). These results demonstrate that αIIbβ3 activation is abolished in Tln1−/− platelets, even at high agonist concentrations. Surface expression of P-selectin was determined as a measure of agonist-induced degranulation. Although normal degranulation was observed in Tln1−/− platelets in response to intermediate and high thrombin concentrations, a significantly reduced response was seen with low thrombin concentrations and the weaker agonist, CRP (Fig. 2 D). Collectively, these data show that the inside-out activation of αIIbβ3 integrin is abrogated in Tln1−/− platelets, but other cellular functions such as shape change and degranulation are either not or slightly affected.
αIIbβ3 integrin is also important for firm platelet adhesion to the extracellular matrix, where it acts in concert with β1 integrins, most notably the collagen-binding α2β1 integrin (21, 25). Therefore, platelet adhesion to a collagen-coated surface was tested in whole blood perfusion assays under conditions of low and high shear stress (150 and 1,000 s−1, respectively). Control platelets readily established firm adhesions on the collagen fibers and rapidly built stable three-dimensional aggregates, both at high and low shear (Fig. 3, A and B, and not depicted), whereas virtually all Tln1−/− platelets either detached within a few seconds or translocated along the fibers before they were released. As a consequence, virtually no Tln1−/− platelets were attached to the collagen surface at the end of the experiment, whereas control platelets covered 53.3 ± 8.6% of the surface area (Fig. 3, A and B). Furthermore, Tln1−/− platelets failed to adhere to soluble type I collagen under static conditions, a process known to be mediated exclusively by α2β1 integrin (unpublished data) (2, 21). Thus, activation of both αIIbβ3 as well as α2β1 is abrogated in Tln1−/− platelets (21, 25).

Defective adhesion and spreading of Tln1−/− platelets. Whole blood from control and talin1−/− mice was perfused over a collagen-coated surface at a wall shear rate of 1,000 s−1. Representative phase contrast images (A) and surface area coverage ± SD for four mice per group (B) taken at the end of the perfusion period (4 min). Bar, 30 μm. (C) Washed wild-type and Tln1−/− platelets were stimulated with 0.01 U/ml thrombin, and then allowed to adhere to immobilized fibrinogen for 45 min. The differential interference contrast images shown are representative of four individual experiments. Bar, 3 μm.
Ligand-occupied integrins transduce signals leading to the activation of Src family kinases resulting in cell spreading. The role of talin in this process was tested by analyzing the adhesion of washed control and Tln1−/− platelets to fibrinogen under static conditions. As mouse platelets, in contrast to human platelets, do not spread well on immobilized fibrinogen without cellular activation (26), the experiments were performed in the presence of 0.01 U/ml thrombin. Comparable adhesion of control and Tln1−/− platelets to the fibrinogen matrix occurred, confirming the previous observation that αIIbβ3 activation is not required for static adhesion of platelets to fibrinogen (27). However, although control platelets readily formed lamellipodia and spread within 10–15 min, Tln1−/− platelets only formed filopodia, with occasional transient small lamellipodia, and completely failed to spread for up to 45 min (Fig. 3 C). Thus, talin1 is also required for αIIbβ3-dependent outside-in signaling. These results differ from observations made with platelets expressing a talin binding–deficient β3 (L746A) integrin mutant, which spread on fibrinogen in the presence of agonists (18). A possible explanation for this discrepancy is that the β3(L746A) mutation only partially disrupts the β3 and talin interaction.
Pathological thrombus formation in vivo was determined by intravital microscopy of injured mesenteric arterioles in bone marrow chimeric Tln1−/− mice. For this, platelets were fluorescently labeled in vivo and injury was induced by topical application of 20% FeCl3. In control mice, platelets rapidly interacted with the injured vessel wall and were firmly attached 5 min after injury (Fig. 4, A and D), after which additional platelets were recruited from the circulation resulting in aggregate formation (Fig. 4 B) and complete vessel occlusion in all mice by 20 min (mean occlusion time, 15.3 ± 2.0 min; Fig. 4, C and D). In contrast, in Tln1−/− mice platelets only transiently attached to the site of injury, no thrombi were formed, and blood flow was maintained throughout the observation period in all analyzed vessels. Thus, talin is essential for platelet attachment to the injured vessel wall, a process mediated by the concerted action of β1 and β3 integrins (28).

In vivo analysis of Tln1−/− platelets in thrombosis. (A) Mesenteric arterioles were injured by FeCl3, and the number of attached fluorescently labeled platelets per mm2 was measured 5 min later. In parallel, the onset of thrombus formation (B) and the time when occlusion occurred (C) were determined. (D) In vivo video microscopy reveals the beginning of thrombus formation after ~7 min, which then leads to vessel occlusion at ~15 min. In contrast to wild-type platelets, Tln1−/− platelets did not adhere to the vessel wall and thombus formation did not occur. Bar, 30 μm.
The molecular mechanisms regulating the inside-out activation of integrins have been studied intensively during the last two decades. One central result of these studies was the proposal that the cytoskeletal protein talin might be a central regulator of this process. Our study now provides unambiguous in vivo evidence for the unique and essential role of talin for the activation of αIIbβ3 and α2β1 integrins in mammals. This was not certain despite recent reports showing that mutations of critical residues in the talin binding site of the β1 and β3 integrin tails, respectively, abrogate integrin activation and lead to severe developmental and hemostatic defects in vivo (8, 18, 29). Integrin β tails bind a large number of focal adhesion proteins, and it was therefore impossible to clarify whether the mutations affect talin only or additional tail-binding proteins that contribute to integrin activation.
Interestingly, Mx-cre–induced loss of talin1 did not significantly affect peripheral platelet counts. Because talin2, which is very similar to talin1, is not expressed in significant amounts in hematopoietic cells (14), our findings suggest that talin is dispensable for megakaryocyte maturation, pro-platelet formation, and platelet shedding. In addition, flow cytometric forward scatter/side scatter profiles of control and Tln1 −/− platelets are indistinguishable, indicating that size and shape of the mutant cells are largely normal as is the expression profile of prominent surface glycoproteins. The only exception is an ~15% reduction in surface expression of integrin αIIbβ3, which, however, does not account for the hemostasis defect because mice carrying a heterozygous-null mutation in the β3 integrin express even less (50%) αIIbβ3 integrin on their platelets without developing a bleeding defect (30).
This indicates that talin does neither make a significant contribution to platelet shape, which has been proposed to depend on the integrity of the cortical cytoskeleton, nor to cytoskeletal rearrangements after cellular activation. However, at low thrombin concentrations or in response to the weaker agonist CRP, Tln1−/− platelets displayed reduced degranulation (Fig. 2 D), indicating that talin deficiency either directly or indirectly impairs granule release. Further studies will be required to address this question.
Both our ex vivo flow adhesion studies as well as the in vivo analysis of platelet adhesion to the injured arterial wall indicate that loss of talin abrogates not only the activation of αIIbβ3 integrin, but also the activation of β1 integrins, which are known to contribute to platelet adhesion to the extracellular matrix in vitro and in vivo (25, 28). This complete abolishment of integrin function resulted in a profound protection from arterial thrombosis but also in a complete loss of primary hemostasis as indicated by infinite bleeding from a tail wound (Fig. 1 D). We did, however, not observe spontaneous intestinal or subcutaneous bleeding in Tln1−/− chimeric mice, as recently reported in mice with a mutation in the β3 integrin subunit (β3 [Y747A]) that abrogates interaction with several intracellular binding partners, including talin and filamin (18). This indicates that the loss of platelet integrin function alone is not sufficient to cause such a severe phenotype in mice, at least not when the deficiency is induced in adult animals. It is possible that the severe spontaneous bleeding observed in β3 (Y747A) mice is caused by a combined defect in platelets and other cells of the vascular system that express β3, such as endothelial cells. Interestingly, another mutation (β3 [L746A]) that is thought to only disrupt talin binding also abrogated inside-out activation of αIIbβ3 in platelets but did not produce severe spontaneous bleedings (18). Interestingly, these platelets exhibited only partial defects in their ability to spread on fibrinogen in the presence of agonists or Mn2 +, indicating that outside-in signaling through αIIbβ3 is intact in those cells. We found that Tln1−/− platelets were completely unable to spread on fibrinogen, suggesting that talin is essential for outside-in signaling. An explanation why outside-in signaling was not completely disrupted in β3 (L746A) platelets could be that talin may still be able to weakly interact with talin. Thus, although reducing the interactions of talin and integrin tails may have promise as a therapeutic modality to reduce thrombus formation in patients with thrombotic disorders (18), complete inhibition of talin1 function results in catastrophic bleeding. These findings have important implications for the development of novel agents to prevent or treat ischemic cerebro- and cardiovascular diseases.
MATERIALS AND METHODS
Mice.
Conditional talin1-deficient mice were generated by introducing loxP sites flanking coding exons 1–4 by gene targeting. Targeting of the Tln1 locus was confirmed by Southern blot of EcoR1-digested genomic DNA hybridized with an external 5′ cDNA probe. Mice were genotyped by PCR using forward primers 5′-aagcaggaacaaaagtaggtctcc-3′ (intron 1) and reverse primers 5′-gcatcgtcttcaccacattcc-3′ (exon 5).
To generate bone marrow chimeras, 4 × 106 cells from the bone marrow of talin1fl/fl-Mx-Cre or talin1fl/fl mice were injected into the tail vein of lethally irradiated (10 Gray) recipient C57BL/6 mice. 3–4 wk after transfer, a single intraperitoneal injection of pI-pC (GE Healthcare) was performed to induce the knockout. Platelets were isolated from whole blood collected from the retro-orbital plexus.
Mice were housed in the animal facility of the Max-Planck Institute of Biochemistry. Mouse breeding and all experimental procedures were approved by the Regierung von Oberbayern and the Regierung von Unterfranken.
Chemicals.
Anesthetic drugs xylazine (Rompun) and ketamine (Imalgene 1000) were from Bayer and Mérial, respectively. High molecular weight heparin and ADP (Sigma-Aldrich) and collagen (Kollagenreagent Horm; Nycomed) were purchased. Monoclonal antibodies conjugated to FITC or PE were from Emfret Analytics.
Western blot analysis.
Platelet lysates were subjected to a 5–15% gradient SDS-PAGE. After blotting, PVDF membranes were probed with anti-talin, anti-actin (both from Sigma-Aldrich), anti-integrin–linked kinase (Transduction Laboratories), anti–β1-integrin, anti-GAPDH (both from Chemicon), anti–c-src (Biosource), and anti–filamin A (Cell Signaling Technology).
Aggregometry.
To determine platelet aggregation, light transmission was measured using washed platelets (200 μl with 0.5 × 106 platelets/μl) in the presence of 70 μg/ml human fibrinogen. Transmission was recorded on a Fibrintimer 4 channel aggregometer (APACT Laborgeräte und Analysensysteme) over 10 min and was expressed as arbitrary units with 100% transmission adjusted with buffer.
Flow cytometry.
Heparinized whole blood was diluted 1:20 with modified Tyrode-Hepes buffer (134 mM NaCl, 0.34 mM Na2HPO4, 2.9 mM KCl, 12 mM NaHCO3, 20 mM Hepes [N-2-hydroxyethylpiperazine-N'-2-ethanesulfonic acid], pH 7.0) containing 5 mM glucose, 0.35% bovine serum albumin (BSA), and 1 mM CaCl2. For glycoprotein expression and platelet count, blood samples were incubated with appropriate fluorophore-conjugated monoclonal antibodies for 15 min at room temperature and directly analyzed on a FACSCalibur (Becton Dickinson). Activation studies were performed with blood samples washed twice with modified Tyrode-Hepes buffer, which then were activated with the indicated agonists for 15 min, stained with fluorophore-labeled antibodies for 15 min at room temperature, and directly analyzed.
Adhesion under flow conditions.
Rectangular coverslips (24 × 60 mm) were coated with 0.25 mg/ml fibrillar type I collagen (Nycomed) for 1 h at 37°C and blocked with 1% BSA. Perfusion of heparinized whole blood was performed as described previously (21). In brief, transparent flow chambers with a slit depth of 50 μm and equipped with the collagen-coated coverslips were rinsed with Hepes buffer and connected to a syringe filled with the anti-coagulated blood. Perfusion was performed at room temperature using a pulse-free pump at low (150 s−1) and high shear stress (1,000 s−1). During perfusion, microscopic phase-contrast images were real-time recorded. Thereafter, the chambers were rinsed by a 10-min perfusion with Hepes buffer, pH 7.45, at the same shear stress, and phase-contrast pictures were recorded from at least five different microscopic fields (×63). Image analysis was performed off-line using Metamorph software (Visitron). Thrombus formation results are expressed as the mean percentage of total area covered by thrombi.
Analysis of bleeding time.
Mice were anesthetized, and a 3-mm segment of the tail tip was cut off with a scalpel. Tail bleeding was monitored by gently absorbing the bead of blood with a filter paper without contacting the wound site. When no blood was observed on the paper after 15-s intervals, bleeding was determined to have ceased. The experiment was stopped after 15 min.
Intravital microscopy of thrombus formation in FeCl3 injured mesenteric arterioles.
4–5-wk-old mice were anesthetized, and the mesentery was gently exteriorized through a midline abdominal incision. Arterioles (35–60 μm diameter) were visualized with a Zeiss Axiovert 200 inverted microscope (×10) equipped with a 100-W HBO fluorescent lamp source and a CCD camera (CV-M300). Injury was induced by topical application of a 3-mm2 filter paper tip saturated with FeCl3 (20%) for 10 s. Adhesion and aggregation of fluorescently labeled platelets in arterioles was monitored for 40 min or until complete occlusion occurred (blood flow stopped for >1 min).
Spreading experiments.
Coverslips were coated overnight with 1 mg/ml human fibrinogen and then blocked for 1 h with 1% BSA in PBS. Washed platelets of mutant or wild-type mice were resuspended at a concentration of 0.5 × 106 platelets/μl, and then further diluted 1:10 in Tyrodes-Hepes buffer. Shortly before seeding platelets on the fibrinogen-coated coverslip, they were activated with 0.01 U/ml thrombin. Platelets were allowed to spread for 45 min and analyzed by differential interference contrast microscopy. At least 10 images of each sample were taken and analyzed using Metavue software (Visitron).
Acknowledgments
We thank Dr. Catrin Pritchard for help in the design and generation of the Tln1 floxed mice and Dr. Roy Zent for insightful discussions and critically reading the manuscript.
This work was supported by the Wellcome Trust, the Rudolf Virchow Center, the Deutsche Forschungsgemeinschaft, and the Max-Planck Society.
The authors have no conflicting financial interests.
References
Articles from The Journal of Experimental Medicine are provided here courtesy of The Rockefeller University Press
Full text links
Read article at publisher's site: https://doi.org/10.1084/jem.20071827
Read article for free, from open access legal sources, via Unpaywall:
https://rupress.org/jem/article-pdf/204/13/3113/1160543/jem_20071827.pdf
Citations & impact
Impact metrics
Citations of article over time
Alternative metrics
Smart citations by scite.ai
Explore citation contexts and check if this article has been
supported or disputed.
https://scite.ai/reports/10.1084/jem.20071827
Article citations
How Protein Depletion Balances Thrombosis and Bleeding Risk in the Context of Platelet's Activatory and Negative Signaling.
Int J Mol Sci, 25(18):10000, 17 Sep 2024
Cited by: 0 articles | PMID: 39337488 | PMCID: PMC11432290
Review Free full text in Europe PMC
Development of the Integrated Computer Simulation Model of the Intracellular, Transmembrane, and Extracellular Domain of Platelet Integrin α IIb β 3 (Platelet Membrane Glycoprotein: GPIIb-IIIa).
TH Open, 8(1):e96-e105, 01 Jan 2024
Cited by: 0 articles | PMID: 38425453 | PMCID: PMC10904213
Talin-1 inhibits Smurf1-mediated Stat3 degradation to modulate β-cell proliferation and mass in mice.
Cell Death Dis, 14(10):709, 31 Oct 2023
Cited by: 1 article | PMID: 37903776 | PMCID: PMC10616178
[Molecular dynamics simulation of force-regulated interaction between talin and Rap1b].
Sheng Wu Yi Xue Gong Cheng Xue Za Zhi, 40(4):645-653, 01 Aug 2023
Cited by: 0 articles | PMID: 37666754 | PMCID: PMC10477389
The critical role of Rap1-GAPs Rasa3 and Sipa1 in T cells for pulmonary transit and egress from the lymph nodes.
Front Immunol, 14:1234747, 20 Jul 2023
Cited by: 2 articles | PMID: 37545505
Go to all (170) article citations
Other citations
Wikipedia
Data
Similar Articles
To arrive at the top five similar articles we use a word-weighted algorithm to compare words from the Title and Abstract of each citation.
Talin is required for integrin-mediated platelet function in hemostasis and thrombosis.
J Exp Med, 204(13):3103-3111, 17 Dec 2007
Cited by: 198 articles | PMID: 18086863 | PMCID: PMC2150986
A talin mutant that impairs talin-integrin binding in platelets decelerates αIIbβ3 activation without pathological bleeding.
Blood, 123(17):2722-2731, 28 Feb 2014
Cited by: 35 articles | PMID: 24585775 | PMCID: PMC3999757
Kindlin-3 is essential for integrin activation and platelet aggregation.
Nat Med, 14(3):325-330, 17 Feb 2008
Cited by: 443 articles | PMID: 18278053
The relative importance of platelet integrins in hemostasis, thrombosis and beyond.
Haematologica, 108(7):1734-1747, 01 Jul 2023
Cited by: 7 articles | PMID: 36700400
Review
Funding
Funders who supported this work.