Abstract
Free full text

Rsk-2 activity is necessary for epidermal growth factor-induced phosphorylation of CREB protein and transcription of c-fos gene
Abstract
Activation by growth factors of the Ras-dependent signaling cascade results in the induction of p90 ribosomal S6 kinases (p90rsk). These are translocated into the nucleus upon phosphorylation by mitogen-activated protein kinases, with which p90rsk are physically associated in the cytoplasm. In humans there are three isoforms of the p90rsk family, Rsk-1, Rsk-2, and Rsk-3, which are products of distinct genes. Although these isoforms are structurally very similar, little is known about their functional specificity. Recently, mutations in the Rsk-2 gene have been associated with the Coffin–Lowry syndrome (CLS). We have studied a fibroblast cell line established from a CLS patient that bears a nonfunctional Rsk-2. Here we document that in CLS fibroblasts there is a drastic attenuation in the induced Ser-133 phosphorylation of transcription factor CREB (cAMP response element-binding protein) in response to epidermal growth factor stimulation. The effect is specific, since response to serum, cAMP, and UV light is unaltered. Furthermore, epidermal growth factor-induced expression of c-fos is severely impaired in CLS fibroblasts despite normal phosphorylation of serum response factor and Elk-1. Finally, coexpression of Rsk-2 in transfected cells results in the activation of the c-fos promoter via the cAMP-responsive element. Thus, we establish a link in the transduction of a specific growth factor signal to changes in gene expression via the phosphorylation of CREB by Rsk-2.
Growth factors act by binding to cell-surface receptors to elicit regulation of cell growth and differentiation. This, in turn, triggers a variety of intracellular signaling pathways that ultimately control cell physiology. Activation of signaling cascades results in changes in the pattern of gene expression through the functional modulation of various transcription factors. These processes allow cells to coordinate long-term physiological adaptation.
The epidermal growth factor (EGF) belongs to the tyrosine kinase class of membrane receptors. EGF binding to its receptor results in activation of the Ras-dependent mitogen-activated protein kinase (MAPK) cascade (1, 2). Coupled phosphorylation events induce mitogen-activated protein kinase kinase (MEK), MAPKs (or extracellular signal-regulated kinases, ERKs), and the p90 ribosomal S6 kinase (p90rsk; refs. 3–5). Nuclear translocation of p90rsk is thought to influence gene expression through phosphorylation of transcription factors (6–9).
The human p90rsk family comprises three closely related isoforms: Rsk-1, Rsk-2, and Rsk-3 (10). A highly conserved feature of these is the presence of two, nonidentical kinase catalytic domains (11). The N-terminal domain appears to be responsible for the phosphorylation of in vitro targets such as S6, c-Fos, serum response factor (SRF), and Nur77 (12). The N-terminal domain seems to be regulated upon activation of the C-terminal catalytic domain by ERKs (13). Little is known about the specificity of the phosphotransferase activity of the different p90rsk members toward a given substrate and upon a specific stimulus. The importance of Rsk-2 within the p90rsk family is demonstrated by the recent finding that mutations in the human Rsk-2 gene are associated with Coffin–Lowry syndrome (CLS). This is a human X-linked disorder, characterized by severe psychomotor retardation, facial and digital dysmorphisms, and progressive skeletal deformations (14, 15). CLS mutations affect Rsk-2 domains critical for kinase activity (14).
Recent evidence indicates that p90rsk may be responsible for cAMP response element-binding protein (CREB) phosphorylation at Ser-133, both in vitro and in vivo, in response to growth factor stimulation (8, 16, 17). CREB is a transcription factor responsive to cAMP. Upon increase of intracellular cAMP, CREB is phosphorylated at Ser-133 by the catalytic subunit of protein kinase A and turns into an activator (18, 19). CREB is phosphorylated on Ser-133 also in response to Ca2+/calmodulin-dependent and stress-activated signaling pathways (18, 20). Thus, CREB is a nuclear effector of multiple signaling pathways.
CREB mediates transcriptional inducibility of c-fos by cAMP through a CRE located at −60 in the promoter (21). c-fos belongs to the class of immediate-early genes whose expression is rapidly and transiently activated as part of the mitogenic response (22–24). Transcription factors contributing to the growth factor induction of c-fos are SRF and Elk-1, which belongs to the Ets family (25, 26). A dimer of SRF and one molecule of Elk-1 (also known as TCF, ternary complex factor) bind to the serum response element (SRE) centered at −300 in the promoter (27). Phosphorylation of SRF and Elk-1, after activation of the MAPKs pathway, appears essential to elicit c-fos transcriptional induction (28, 29). The specific contribution of CREB to the growth factor-induced expression of c-fos is not fully understood.
Here we document the pattern of CREB phosphorylation and c-fos expression upon EGF stimulation of a fibroblast cell line established from CLS patients. Loss of Rsk-2 function results in a severe reduction of CREB phosphorylation in response to EGF. The effect on CREB is specific as response to other signaling pathways is normal in CLS fibroblasts. Lack of EGF-induced CREB phosphorylation in CLS fibroblasts correlates to a drastic reduction in c-fos induction. Importantly, SRF and Elk-1 phosphorylation in response to EGF is normal in Rsk-2-deficient cells. Thus, Rsk-2-mediated CREB phosphorylation is a prerequisite for c-fos inducibility by EGF. Finally, we demonstrate that the c-fos CRE is sufficient to direct the transcriptional response to Rsk-2.
MATERIALS AND METHODS
Antibodies and Cells.
Fibroblasts from CLS patients were obtained as described (14) and cultured in DMEM with 10% fetal calf serum (FCS). COS cells were maintained in DMEM/5% FCS. Antibodies to phosphorylated and unphosphorylated CREB (Ser-133; anti-P-CREB), MAPKs (Tyr-204; anti-P-MAPK), and Elk-1 (Ser-383; anti-P-Elk-1) were from New England Biolabs as well as antibodies detecting total levels of these proteins. Anti-p90rsk antibodies specific for each isoform were obtained from Santa Cruz Biotechnology, while the anti-pan-p90rsk antibodies were from Transduction Laboratories.
Western Analysis.
Cells were starved for 24 hr before treatments and harvested in boiling SDS sample buffer after washing twice in PBS. Cell lysates were resolved by standard SDS/10% PAGE. Equal loading was confirmed with Coomassie blue staining. Samples were electroblotted onto Protran nitrocellulose (Schleicher & Schuell). Membranes were incubated in PBS/5% low-fat milk and specific antibody for 12 hr at 4°C. Donkey, anti-rabbit-HRP antibodies were used to reveal immunocomplexes by enhanced chemiluminescence.
RNA Analysis.
Total RNA was extracted as described (30). Total RNA (10 μg) was analyzed by RNase protection (31). A pBluescript SK(−) plasmid containing the human c-fos cDNA was linearized at a unique NcoI site (+2530) to prepare an RNA probe (from +2530 to +2676). The antisense probe was generated from the T7 promoter by using an in vitro transcription kit (Promega). Equal amounts of RNA were used in all analyses, and tRNA and histone H4 were used as controls.
DNA-Binding Assay.
Nuclear extracts were prepared as described (32). A 24-bp oligonucleotide (5′-GTCGGATGTCCATATTAGGACATCT-3′) containing the c-fos SRE was 32P end-labeled and used in binding reactions. Three micrograms of nuclear extracts was added to 20 μl DNA binding mixture [20 mM Hepes, pH 7.9/1 mM EDTA/1 mM DTT/10% glycerol/60 mM KCl/1 μg of poly(dI-dC)]. Reactions were preincubated at room temperature for 10 min and continued for 15 min after addition of the probe. Four percent nondenaturing polyacrylamide gels in a 0.25× Trisborate/EDTA buffer were used. Two microliters of anti-P-SRF antibody was added to equilibrium-binding reactions and incubated further for 10 min.
Transfections.
Cells were plated at 5 × 105 per 6-cm plate and transfected by the calcium phosphate coprecipitation technique with 10 μg of plasmid DNA, by normalizing with pBluescript. Chloramphenicol acetyltransferase (CAT) activity was assayed as described (21). The expression vector for Rsk-2 is based on pSG5 (34). The c-fos reporters FC4 and FC8 have been described (35) and correspond to promoter deletions at −404 and −220, respectively.
RESULTS
Levels of Rsk-1 and Rsk-3 Are Normal in CLS Fibroblasts.
To perform the present study we selected a fibroblastic cell line originated from one CLS patient, as described previously (14). All mutations in Rsk-2 from CLS patients whose activity has been tested have revealed an impaired kinase function (refs. 14 and 36; K. Merienne, S.J., and A.H., unpublished data). Fibroblasts are originated from a CLS patient (DX34; Fig. Fig.1A1A Lower) bearing a Rsk-2 lacking the entire second catalytic domain. This deletion is a result of a splice-site mutation leading to the use of a cryptic acceptor site and premature termination at amino acid 422 (14). A Rsk-2 kinase carrying this deletion is nonfunctional as established by mutational studies and S6 phosphorylation assays (L. Monaco and D. Allis, unpublished data). MAPK binding and subsequent Rsk-2 activation are impaired in the DX34 variant since the ERK binding site is localized within the C-terminal 44 residues of p90rsk (13). Such a deletion results in impaired EGF responsiveness of p90rsk kinase activity (13). The DX34 cells contain Rsk-1 and Rsk-3 protein levels comparable to normal fibroblasts as revealed by specific antibodies (Fig. (Fig.11B). The truncated Rsk-2 protein is not detectable with specific anti-Rsk-2 antibodies as these are directed toward the C-terminal domain missing in DX34 (Fig. (Fig.11B). Using general anti-p90rsk antibodies directed toward the N-terminal domain of the various Rsk proteins, we observed a decrease in the intensity of the p90rsk band in the DX34 cells, as the contribution of Rsk-2 is lacking (Fig. (Fig.11C). Importantly, a putative truncated Rsk-2 product is not observed in DX34 cells, indicating that the mutated protein is unstable (Fig. (Fig.11C).
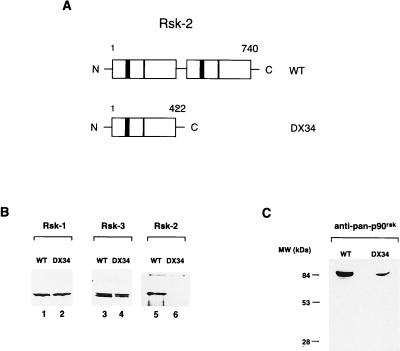
Rsk-2 mutation in DX34 Coffin–Lowry patient. (A Upper) Schematic representation of the wild-type Rsk-2 protein. (Lower) Predicted Rsk-2 protein in fibroblasts from DX34 Coffin–Lowry patient (14). Numbers refer to the amino acid length of the proteins. ATP-binding sites are shown as solid boxes, and APE (A = alanine, P = proline, E = glutamic acid) sites are shown as hatched boxes. (B) Levels of Rsk-1, Rsk-3, and Rsk-2 in normal and CLS fibroblasts. Total cell lysates from cells were analyzed by Western blot by using anti-Rsk-1, Rsk-3, and Rsk-2 specific antibodies (see Materials and Methods). Rsk-2 is not detectable in DX34 cells as the antibody is directed against the C terminus of the protein. (C) Levels of p90rsk using a general anti-p90rsk antibody (see Materials and Methods). The total level of p90rsk is lower in DX34 cells as the contribution of Rsk-2 is missing. There is no evidence for a truncated Rsk-2 protein in DX34 cells.
Rsk-2 Is Required for EGF-Stimulated Phosphorylation of CREB at Ser-133.
We wished to assess whether the loss of Rsk-2 function resulted in alteration of specific signaling leading to CREB phosphorylation. Rsk-2-deficient cells were stimulated by serum and EGF, and CREB phosphorylation was examined. We also treated cells with forskolin and UV light to monitor the effect of cAMP- and stress-induced signaling pathways. Equivalent treatments were performed on a fibroblast cell line established from a normal individual (RSK2+, Fig. Fig.2).2). P-CREB levels were undetectable in both Rsk-2+ and Rsk-2− untreated fibroblasts (lane 1). CREB was readily phosphorylated after forskolin, UV light, and serum stimulation, both in normal and Rsk-2− fibroblasts (lanes 2–4). In contrast, while CREB phosphorylation is increased within 5 min of EGF treatment in Rsk-2+ cells, strikingly no significant phosphorylation is detectable in CLS cells (lanes 5 and 6). Thus, lack of CREB phosphorylation in CLS cells is specific to EGF induction. An anti-CREB antibody recognizing both phosphorylated and unphosphorylated forms reveals that, as expected (37), protein levels do not change upon treatments.
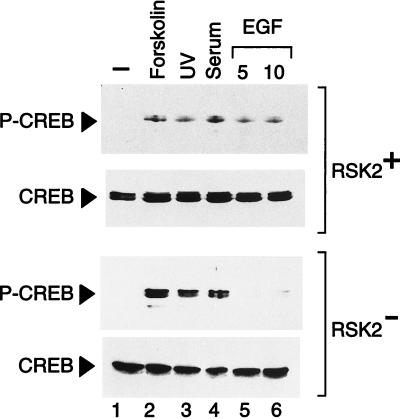
Rsk-2 is necessary for CREB phosphorylation upon activation of EGF signaling pathway. Normal (RSK2+) and CLS (RSK2−) cells were starved 24 hr in the absence of serum before harvesting. Cell extracts were quantified by Coomassie blue staining. Lanes: 2, forskolin was utilized at a concentration of 10 μg/ml for 30-min stimulation; 3, cells were irradiated for 10 sec and harvested 30 min after exposure to UV (as in ref. 20); 4, fetal calf serum was added at 20% final concentration for 10 min; 5 and 6, cells were exposed to 30 ng/ml of EGF for 5 and 10 min (lanes 5 and 6, respectively). CREB phosphorylation levels in Rsk-2− cells upon EGF induction are about 5% of the forskolin-induced level in the same cells and 10% of the corresponding EGF induction in Rsk-2+ cells, after adjusting with the basal levels.
MAPK Response to EGF Is Normal in Rsk-2-Deficient Cells.
p90rsk proteins are activated by ERK-1 and ERK-2 upon triggering of the EGF pathway (13). We wished to analyze the levels of activated ERKs in normal and Rsk-2− cells upon EGF treatment. ERKs occur through phosphorylation of threonines and tyrosines by a single upstream MAPK kinase (MEK) (3, 5, 38). A specific P-MAPK antibody was used to detect catalytically activated ERKs at Tyr-204 (39). Serum and EGF treatments induce ERK phosphorylation in both normal and mutated fibroblasts, indicating that the MAPK response is present in Rsk-2− cells (Fig. (Fig.3,3, lanes 4–6). Activation of the cAMP-dependent pathway did not induce ERK phosphorylation (lane 2). These results also demonstrate that the loss of CREB phosphorylation in CLS fibroblasts is not linked to a possible down-regulation of the EGF receptor in Rsk-2− cells and that the block is downstream of MAPKs. MAPK levels in normal and CLS fibroblasts are comparable as demonstrated by an anti-MAPK antibody (Fig. (Fig.3).3).
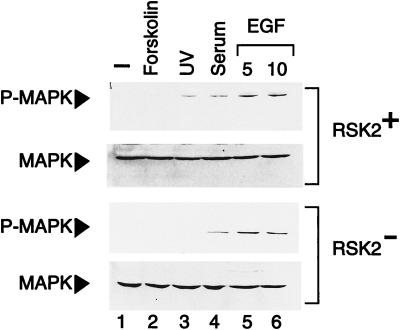
MAPK response is normal in cells from CLS patients. Normal (RSK2+) and CLS (RSK2−) cells were starved 24 hr in the absence of serum before treatment and then harvested. An antibody recognizing the phosphorylated MAPK was used (see Materials and Methods). Lanes: 2, forskolin was added at the concentration of 10 μg/ml for 10 min; 3, cells were exposed for 10 sec to UV rays and harvested 30 min after irradiation (20); 4, samples were stimulated in the presence of 20% fetal calf serum for 10 min; 5 and 6, cells were treated with 30 ng/ml of EGF for the indicated times. Total levels of MAPK are uniform in all samples.
Rsk-2 Activity Is Necessary for EGF Induction of c-fos.
Activation of the c-fos through various signaling pathways is basically ensured by two elements, the SRE and the CRE (21, 41, 42). The CRE is responsive to cAMP and Ca2+ (21, 43, 44) and seems important in the response to nerve growth factor (45). We asked whether the absence of induced CREB phosphorylation in Rsk-2− cells may correlate with an altered c-fos response to mitogenic and growth factor stimulation. We analyzed c-fos transcripts in normal and CLS cells after induction with serum and EGF (Fig. (Fig.4).4). In both lines, a remarkable c-fos induction was detected 15 min after serum stimulation (lanes 2 and 5), peaking at 30 min (lanes 3 and 6) and declining by 60 min (lanes 4 and 7). A similar inducibility profile was observed in normal and CLS fibroblasts (Fig. (Fig.4).4). EGF treatment also led to a robust induction of c-fos expression in normal cells (lanes 5–7). In striking contrast, EGF-induced c-fos transcription is almost completely abolished in Rsk-2− cells (lanes 5–7). This result demonstrates that induction of c-fos by EGF, but not by serum, requires Rsk-2 activity.
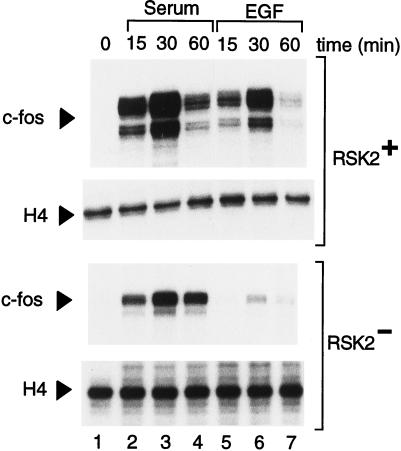
Induction of c-fos transcription by EGF requires Rsk-2 activity. Normal (RSK2+) and CLS (RSK2−) fibroblasts were starved 24 hr before induction with serum and EGF and then harvested at the indicated times. Ten micrograms of total RNA was used per lane, and RNase protection was performed as described (see Materials and Methods). Lanes: 2–4, cells were stimulated with 20% fetal calf serum; 5–7, fibroblasts were exposed to 30 ng/ml of EGF. The peak of c-fos-induced expression by EGF in the Rsk-2− cells is 5% of the signal obtained with serum in the same cells and 2% of the EGF peak in the Rsk-2+ cells (after adjusting with basal levels). Transcript levels of histone H4 were monitored as internal control.
Transcription Factor Elk-1 Is Activated in Rsk-2− Cells.
A ternary complex of Elk-1 and SRF is essential for serum and growth factor induction of c-fos (25, 26, 46). Elk-1 is phosphorylated after activation of the MAPK pathway at a C-terminal cluster of Ser/Thr. Direct phosphorylation by ERKs at these sites, and particularly at Ser-383, is critical for Elk-1 function (28, 47). Using specific anti-P-Elk-1 antibodies we tested whether Elk-1 phosphorylation in response to EGF treatment occurred in Rsk-2− cells. Elk-1 is readily phosphorylated upon serum and EGF treatment both in normal and CLS fibroblasts (Fig. (Fig.5,5, lanes 4 and 5, respectively), confirming that ERKs are active in Rsk-2− cells. No P-Elk-1 was detected in untreated cells (lane 1) or upon induction of the cAMP pathway by forskolin (lane 2). In contrast, UV irradiation of cells led to a robust Elk-1 phosphorylation (lane 3), as already reported (48). This result confirms that the UV-induced stress-activated pathway is unaltered in Rsk-2− fibroblasts.
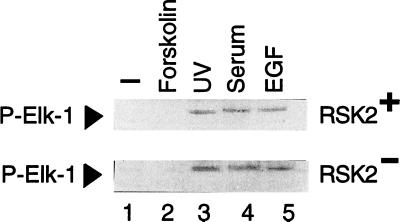
Rsk-2 is not necessary for TCF/Elk-1 phosphorylation. Normal (RSK2+) and CLS (RSK2−) fibroblasts were deprived of serum for 24 hr before treatment. Lanes: 2, 10 μg/ml of forskolin were used for 30-min stimulation; 3, cells were UV-irradiated for 10 sec and harvested 20 min later (19); 4, fetal calf serum was added at a 20% concentration; 5, EGF was used at the concentration of 30 ng/ml for 10-min stimulation.
SRF Is Normally Phosphorylated in CLS Fibroblasts.
SRF is differentially phosphorylated at Ser-103 (49). Upon growth factor stimulation of quiescent fibroblasts, phosphorylation of Ser-103 is thought to regulate SRF DNA-binding activity (49). p90rsk has been shown to phosphorylate SRF on Ser-103, at least in vitro, although the specific member of the p90rsk family responsible for this phosphorylation has not been identified (49). Anti-P-SRF antibodies were used to investigate whether serum and growth factor stimulation of quiescent Rsk-2− fibroblasts led to induced SRF phosphorylation at Ser-103. Phosphorylation of SRF in nuclear extracts was assayed in a DNA mobility shift assay, as originally reported (ref. 49; Fig. Fig.6).6). It has been demonstrated that addition of the anti-P-SRF antibodies to the DNA-binding reaction results in a characteristic retardation in the mobility of the SRF–SRE complex (49). While only a small proportion of SRF in quiescent cells was recognized by the anti-P-SRF antibody (lanes 2 and 6), a significant proportion of the SRF–SRE complex was super-shifted in serum and EGF-stimulated fibroblasts (lanes 3 and 4 and 7 and 8, respectively). The super-shift was specific as tested by the use of preimmune (lane 9) and unrelated antibodies (not shown). The results are qualitatively and quantitatively identical for control and Rsk-2− fibroblasts (compare lanes 3 and 4 with lanes 7 and 8). These results demonstrate that SRF is similarly phosphorylated in normal and Rsk-2− fibroblasts upon EGF induction.

Rsk-2 is not necessary for SRF phosphorylation. Normal (RSK2+) and CLS (RSK2−) cells that had been starved for 24 hr were treated with 20% fetal calf serum and 30 ng/ml of EGF for 10 min before preparing nuclear extracts. Three micrograms of nuclear proteins was used per lane. α-Phospho-SRF (α-P-SRF) antibodies were added to the equilibrium-binding reactions (lanes 3–5 and 6–8) for 10 min. The specific SRF/SRE complex is indicated by an arrow. The SRF/SRE/α-P-SRF super-shifted complex is indicated by an arrowhead. Lane 9: equilibrium-binding reaction was incubated with preimmune serum for 10 min before loading onto the polyacrylamide gel.
The CRE Is Sufficient for Induction of c-fos Expression by Rsk-2.
The above results strongly suggested that phosphorylation of CREB by Rsk-2 is a critical event leading to c-fos transcriptional activation upon EGF induction. In addition, SRF- and Elk-1-regulated phosphorylation does not require Rsk-2 (Figs. (Figs.55 and and6).6). To test whether the c-fos CRE is sufficient to direct Rsk-2-dependent transcriptional activation, we transiently transfected COS cells with c-fos promoter constructs FC4 and FC8 (ref. 21; Fig. Fig.77A). While FC4 contains both SRE and CRE (up to position −404), the SRE is deleted in FC8 (deletion at −220). The responsiveness of these reporter plasmids to various stimuli has been studied extensively (35, 50). Here we show that both FC4 and FC8 transcription is stimulated significantly, although to a different extent, upon cotransfection with a Rsk-2 expression vector (Fig. (Fig.77B). Additional experiments including various control and mutated reporters, different expression vectors, and cell types confirmed the extent and specificity of this effect (D.D.C., unpublished data). Thus, the CRE is sufficient to direct responsiveness to Rsk-2.
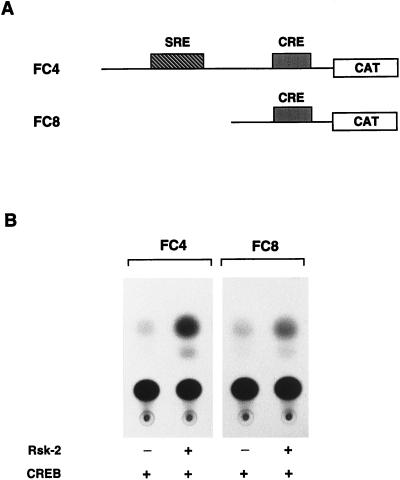
The CRE is sufficient to mediate induction of c-fos expression by Rsk-2. (A) Schematic representation of FC4 and FC8 reporter plasmids (35); binding sites for Elk-1/SRF (SRE) and CREB (CRE) are indicated. (B) COS cells were transfected with 0.5 μg of FC4 and FC8 reporter plasmids in combination with 0.2 μg of CREB expression vector and 2 μg of Rsk-2 expression vector when indicated. Cells were harvested 36 hr after addition of calcium phosphate precipitate. Rsk-2-mediated activation is 7.5 (±0.5)-fold for FC4 and 4.5 (±0.5)-fold for FC8, as established by photometric scanning of the autoradiograms. The results presented are representative of three independent experiments.
DISCUSSION
We have used a fibroblast cell line established from patients affected by the Coffin–Lowry syndrome to demonstrate that activity of the p90rsk isoform Rsk-2 is necessary for EGF-triggered phosphorylation of CREB at Ser-133 in vivo. In this cell line the Rsk-2 gene is disrupted by a splice-site mutation leading to a truncated protein lacking the C-terminal catalytic domain (14). In drastic contrast to normal fibroblasts, CREB phosphorylation is severely and specifically impaired upon EGF stimulation in these CLS cells (Fig. (Fig.22).
In mammalian cells, p90rsk is activated in response to a broad range of stimuli, including oncogenic transformation (51) and exposure to growth factors and phorbol esters (52). CREB is readily phosphorylated upon forskolin treatment and UV irradiation in CLS fibroblasts (Fig. (Fig.2),2), underscoring that lack of active Rsk-2 does not result in a global perturbation of other signaling cascades. Also, serum-induced CREB phosphorylation appears unaltered in Rsk-2− cells (Fig. (Fig.2).2). Serum factors induce a number of kinases, including p70S6K, a distinct member of the ribosomal S6 kinase family. Interestingly, activated p70S6K has been demonstrated to phosphorylate the CREM/CREB transcription factors upon serum stimulation (53). However, pretreatment of CLS fibroblasts with rapamycin, a specific p70S6K inhibitor, did not interfere with the serum-induced phosphorylation of CREB (data not shown). Thus, we speculate the existence of a different kinase or a yet unidentified p90rsk isoform in fibroblasts that phosphorylates CREB in response to serum. In this regard, it should be noted that all three members of the p90rsk family have been shown to be activated, although to various degrees, by serum and EGF, using immunoprecipitated kinases from treated cells (13). Recently, all p90rsk members have been shown to be able to phosphorylate CREB in response to nerve growth factor in PC12 cells (54). Our results, however, demonstrate a specifically impaired response to EGF in CLS fibroblasts, which strongly implies that the other p90rsk members cannot compensate for the loss of Rsk-2 function (Figs. (Figs.22 and and4).4). Thus, a specificity of the different p90rsk members with respect to a given stimulus and/or a particular substrate seems to exist in vivo. Another possibility is that the mutated form of Rsk-2 in CLS cells has a dominant-negative effect on Rsk-1 and Rsk-3.
The rapid and transient transcriptional activation of immediate-early genes is a key event leading to cell proliferation in response to growth factors (24). Here we have shown that the lack of EGF-induced phosphorylation of CREB in CLS cells correlates with an impaired c-fos induction. The elements involved in the response to growth factors and serum within the c-fos promoter are well characterized (26, 55, 56), as well as the involvement of Elk-1 and SRF (26, 57). Elk-1 is directly phosphorylated by activated ERKs (28, 29), while SRF phosphorylation in response to mitogenic stimuli at a site that regulates its DNA-binding activity is thought to involve p90rsk, although no direct in vivo evidence is available (49). In this report, we show that both SRF and Elk-1 are readily phosphorylated in the Rsk-2− fibroblasts in response to both serum and EGF (Figs. (Figs.55 and and66).
Induction of c-fos transcription in response to serum and growth factors is known to require SRF and Elk-1 (26, 42, 47). Our experiments indicate that the presence of active Elk-1 and SRF is not sufficient for efficient regulation of c-fos expression in response to EGF, whereas phosphorylation of CREB at Ser-133 appears to be essential for the normal response. In CLS cells we have observed a drastically impaired induction of c-fos expression in response to EGF (Fig. (Fig.4)4) and we have demonstrated that the CRE within the promoter is sufficient to direct the response to Rsk-2 (Fig. (Fig.7).7). These results indicate that the c-fos CRE plays a key role in the response to growth factors. We believe that SRE and CRE operate in concert to ensure full growth-factor responsiveness of the c-fos gene. Consistently, mutations within the CRE effectively diminish nerve growth factor induction of c-fos transcription (39, 42, 45). In addition, experiments in transgenic mice have established a role for the c-fos CRE as a basal transcription element (58).
We have investigated the role of a distinct p90rsk isoform in modulating the activity of transcription factors and gene expression in response to a specific stimulus. Our findings might help to shed light on the physiological basis of CLS. c-fos has been implicated directly in regulating differentiation and the activity of specific bone cell populations during development (59), a process in which EGF plays an essential role (60, 61). On this basis, it could be anticipated that the loss of normal c-fos regulation in CLS patients may account for some of the developmental abnormalities that involve EGF-regulated processes. In support of this notion, the discovery of tissue-specific differences in gene expression of the p90rsk family members has been used to predict distinct physiological roles for the various members (10). In this regard, it will be interesting to investigate how, at the level of the whole organism, the loss of Rsk-2 function affects phosphorylation of transcription factors and expression of target genes in different tissues. The development of mice by homologous recombination carrying targeted mutations at the Rsk-2 locus will represent a powerful tool to study Rsk-2 function and to elucidate the molecular mechanisms generating the CLS phenotype.
Acknowledgments
We thank M. E. Greenberg for the gift of the anti-phospho-SRF antibodies. We thank L. Monaco, G. M. Fimia, N. S. Foulkes, A. R. Saltiel, T. Leff, J. L. Mandel, C. Mizzen, and D. C. Allis for discussions. The technical help of E. Heitz is appreciated. D.D.C. is supported by a long-term fellowship from the European Community. This study was funded by grants from Centre National de la Recherche Scientifique, Institut National de la Santé et de la Recherche Médicale, Centre Hospitalier Universitaire Régional, Fondation de la Recherche Médicale, and Association pour Recherche sur le Cancer.
ABBREVIATIONS
CLS | Coffin–Lowry syndrome |
EGF | epidermal growth factor |
RSK | ribosomal S6 kinase |
MAPK | mitogen-activated protein kinase |
ERK | extracellular regulated kinase |
SRE | serum response element |
SRF | serum response factor |
CRE | cAMP response element |
CREB | CRE-binding protein |
References
Articles from Proceedings of the National Academy of Sciences of the United States of America are provided here courtesy of National Academy of Sciences
Full text links
Read article at publisher's site: https://doi.org/10.1073/pnas.95.21.12202
Read article for free, from open access legal sources, via Unpaywall:
https://europepmc.org/articles/pmc22809?pdf=render
Citations & impact
Impact metrics
Citations of article over time
Alternative metrics
Article citations
RSK2-mediated cGAS phosphorylation induces cGAS chromatin-incorporation-mediated cell transformation and cancer cell colony growth.
Cell Death Discov, 10(1):442, 18 Oct 2024
Cited by: 0 articles | PMID: 39424777 | PMCID: PMC11492232
Multi-faceted regulation of CREB family transcription factors.
Front Mol Neurosci, 17:1408949, 06 Aug 2024
Cited by: 0 articles | PMID: 39165717 | PMCID: PMC11333461
Review Free full text in Europe PMC
Resolving the role of podoplanin in the motility of papillary thyroid carcinoma-derived cells using RNA sequencing.
Comput Struct Biotechnol J, 21:3810-3826, 26 Jul 2023
Cited by: 0 articles | PMID: 37560122 | PMCID: PMC10407544
A short peptide LINC00665_18aa encoded by lncRNA LINC00665 suppresses the proliferation and migration of osteosarcoma cells through the regulation of the CREB1/RPS6KA3 interaction.
PLoS One, 18(6):e0286422, 07 Jun 2023
Cited by: 4 articles | PMID: 37285335 | PMCID: PMC10246827
Go to all (181) article citations
Data
Similar Articles
To arrive at the top five similar articles we use a word-weighted algorithm to compare words from the Title and Abstract of each citation.
Ribosomal subunit kinase-2 is required for growth factor-stimulated transcription of the c-Fos gene.
Proc Natl Acad Sci U S A, 97(6):2462-2467, 01 Mar 2000
Cited by: 46 articles | PMID: 10716983 | PMCID: PMC15951
Phosphorylation of p90 ribosomal S6 kinase (RSK) regulates extracellular signal-regulated kinase docking and RSK activity.
Mol Cell Biol, 23(14):4796-4804, 01 Jul 2003
Cited by: 124 articles | PMID: 12832467 | PMCID: PMC162206
Glutamate induces phosphorylation of Elk-1 and CREB, along with c-fos activation, via an extracellular signal-regulated kinase-dependent pathway in brain slices.
Mol Cell Biol, 19(1):136-146, 01 Jan 1999
Cited by: 217 articles | PMID: 9858538 | PMCID: PMC83872
P90 RSK arranges Chk1 in the nucleus for monitoring of genomic integrity during cell proliferation.
Mol Biol Cell, 23(8):1582-1592, 22 Feb 2012
Cited by: 36 articles | PMID: 22357623 | PMCID: PMC3327324