Abstract
Free full text

The maturity-onset diabetes of the young (MODY1) transcription factor HNF4α regulates expression of genes required for glucose transport and
metabolism
Abstract
Hepatocyte nuclear factor 4α (HNF4α) plays a critical role in regulating the expression of many genes essential for normal functioning of liver, gut, kidney, and pancreatic islets. A nonsense mutation (Q268X) in exon 7 of the HNF4α gene is responsible for an autosomal dominant, early-onset form of non-insulin-dependent diabetes mellitus (maturity-onset diabetes of the young; gene named MODY1). Although this mutation is predicted to delete 187 C-terminal amino acids of the HNF4α protein the molecular mechanism by which it causes diabetes is unknown. To address this, we first studied the functional properties of the MODY1 mutant protein. We show that it has lost its transcriptional transactivation activity, fails to dimerize and bind DNA, implying that the MODY1 phenotype is because of a loss of HNF4α function. The effect of loss of function on HNF4α target gene expression was investigated further in embryonic stem cells, which are amenable to genetic manipulation and can be induced to form visceral endoderm. Because the visceral endoderm shares many properties with the liver and pancreatic β-cells, including expression of genes for glucose transport and metabolism, it offers an ideal system to investigate HNF4-dependent gene regulation in glucose homeostasis. By exploiting this system we have identified several genes encoding components of the glucose-dependent insulin secretion pathway whose expression is dependent upon HNF4α. These include glucose transporter 2, and the glycolytic enzymes aldolase B and glyceraldehyde-3-phosphate dehydrogenase, and liver pyruvate kinase. In addition we have found that expression of the fatty acid binding proteins and cellular retinol binding protein also are down-regulated in the absence of HNF4α. These data provide direct evidence that HNF4α is critical for regulating glucose transport and glycolysis and in doing so is crucial for maintaining glucose homeostasis.
Hepatocyte nuclear factor 4α (HNF4α) belongs to the steroid/thyroid hormone receptor superfamily of transcription factors and first was identified by its interaction with a cis-regulatory sequence of liver specific gene promoters (1). Like other members of the family, HNF4α contains a zinc finger regions and binds DNA as a dimer. The carboxyterminal region contains a large hydrophobic domain (amino acids 133–373) reminiscent of the dimerization and ligand binding domain of other steroid hormone receptors (2). Although HNF4α displays significant sequence similarities to the mammalian retinoic-x receptor α, it does not heterodimerize with any of the other nuclear receptors identified and exists as stable homodimers in solution (3). Targeted disruption of the HNF4α gene results in early embryonic death caused by dysfunction of the visceral endoderm (VE) in which it is expressed (4, 5). In the adult HNF4α is located primarily in the liver, gut, kidney, and pancreatic islets (1, 6). HNF4α interacts with regulatory elements in promoters and enhancers of genes whose products are involved in diverse function, including cholesterol, fatty acid, amino acid, and glucose metabolism, as well as liver development and differentiation (5, 7, 8). Furthermore it has been demonstrated that HNF4α is critical for regulating expression of numerous genes in vivo (9). We recently have shown that a mutation in HNF4α can cause a form of early-onset type 2 diabetes (maturity-onset diabetes of the young; gene named MODY1) (10). The molecular mechanisms by which mutations in HNF4α cause an autosomal dominant form of type 2 diabetes are unknown. Clinical studies suggest that MODY1 is characterized by a defect in glucose-stimulated insulin secretion, suggesting that abnormal gene expression in the pancreatic β-cell is responsible for this disorder (11). The present study was designed to determine the biological function of the MODY1 HNF4(Q268X) mutation and the effect on the transactivation of downstream target genes. We demonstrate that the mutant truncated HNF4α protein has lost its transcriptional transactivation function and does not bind to HNF4 binding sites in vivo. Furthermore, we show that loss of function of HNF4α leads to impaired expression of genes involved in glucose transport and glycolysis, two steps known to be important for glucose uptake from the enterohepatic circulation into hepatocytes and for insulin secretion of pancreatic β-cells.
MATERIALS AND METHODS
Expression of Proteins and Immunoblot Analysis.
cDNAs for HNF4α and the deletion mutants cloned into Bluescript were transcribed by using T3 RNA polymerase, and the transcripts were translated in the rabbit reticulocyte lysate TNT system (Promega) as described (12). The relative amounts of the translated proteins were determined by SDS/PAGE.
Electrophoretic Mobility-Shift Assay (EMSA).
In vitro- translated receptor protein was incubated with the 32P-labeled oligonucleotide in a 15-μl reaction mixture containing 10 mM Hepes buffer, 50 mM KCl, 1.0 mM DTT, 2.5 mM MgCl2, 10% glycerol, and 1 μg of poly(dI-dC) at 25°C for 20 min. The reaction mixture then was loaded on a 6% nondenaturing polyacrylamide gel containing 0.25× TBE buffer (0.023 M Trisborate 0.5 mM EDTA) and run at 4°C. Supershift analysis was performed by incubating the reaction mix with antiserum at room temperature for 20 min.
Tissue Culture, Transient Transfection, and Luciferase Assay.
Cos-7 and HepG2 cells were grown in DMEM supplemented with 10% and 15% fetal calf serum, respectively. A modified calcium phosphate precipitation procedure was used for transient transfections as described elsewhere (13). Luciferase was normalized for transfection efficiency by the corresponding β-galactosidase activity (13).
In Situ Hybridization.
In situ hybridizations were performed as described previously (5). Sense and antisense aldolase B (aldoB) and HNF4α, [33P]-UTP-labeled RNA probes were synthesized in vitro from plasmids pBS-aldoB and pa8–41. Probes were hybridized to paraffin sections of day 7 and 14 J1 embryonic stem (ES) cell aggregates and exposed to photographic emulsion for 14 days, before developing and counterstaining with hematoxylin and eosin.
Growth and Differentiation of ES Cells in Vitro.
ES cells were maintained in ES cell medium supplemented with 1,000 units of leucemia inhibitory factor on a primary embryonic fibroblast feeder layer as described (14). ES cells were induced to differentiate to form embryoid bodies (EBs) in vitro by growing them in tissue culture dishes in the absence of feeder fibroblasts and leucemia inhibitory factor for the specified duration.
Reverse Transcriptase-PCRs.
Total RNA was extracted from ES cells by using TRIzol reagent and following the manufacturer’s instructions (GIBCO/BRL). Contaminating genomic DNA was removed by using 1 μl of RNase-free DNase-I (Boehringer)/10 μg total RNA. cDNA was synthesized by using moloney murine leucemia virus-reverse transcriptase with dNTPs and random hexamer primers (Stratagene). The cDNAs provided templates for PCRs by using specific primers in the presence of dNTPs, [α-32P]dCTP, and Taq DNA polymerase. The primer sequences used are available upon request.
RESULTS
The MODY1 HNF4(Q268X) Is a Loss of Function Mutation.
The HNF4(Q268X) mutation found at the MODY1 locus was predicted to delete the carboxyl-terminal 187 amino acids of HNF4α (10, 15). This raised the possibility that the truncated mutant protein could act dominantly by affecting the function of the endogenous wild-type HNF4α or by exhibiting an altered transactivation activity. We therefore compared the DNA binding, dimerization, and transcriptional transactivation activities of mutant and wild-type HNF4α proteins.
HNF4α and the MODY1 mutant (Q268X) as well as a control dominant-negative mutant (E360X) were cloned into the mammalian expression vector cytomegalovirus (CMV)-β (16). All three constructs were tagged with a FLAG epitope at their respective C-termini to allow identification (17). The various constructs were cotransfected into cos7 and HepG2 cells along with a reporter plasmid pZLHIV-A1-4, which contains four HNF4 binding sites of the apolipoprotein AI promotor upstream of the HIV-long terminal repeat basal promotor and confers HNF4α responsiveness to a luciferase reporter gene. The expression levels and integrity of the wild-type HNF4α and its deletion mutants were found to be similar by Western blot analysis (data not shown). Fig. Fig.11A shows that the HNF4α deletion mutants did not activate the transcription of the reporter gene, whereas the wild-type HNF4α increased its expression by ≈120-fold. To investigate the possibility that the MODY1 HNF4(Q268X) mutation acts as a dominant-negative regulator, we cotransfected the deletion mutants with wild-type HNF4α and the reporter plasmid ApoAI.HIV-Luc. No effect on transcriptional activity of wild-type HNF4α was detected in cotransfections with increasing amounts of the HNF4(Q268X) mutation (Fig. (Fig.11B). In contrast, potent inhibition of wild-type activity was seen in cotransfections with the HNF4(E360X), which recently has been shown to act as a dominant-negative regulator, most likely as a result of heterodimerization with wild-type HNF4α (Figs. (Figs.11C and and22D) (18).
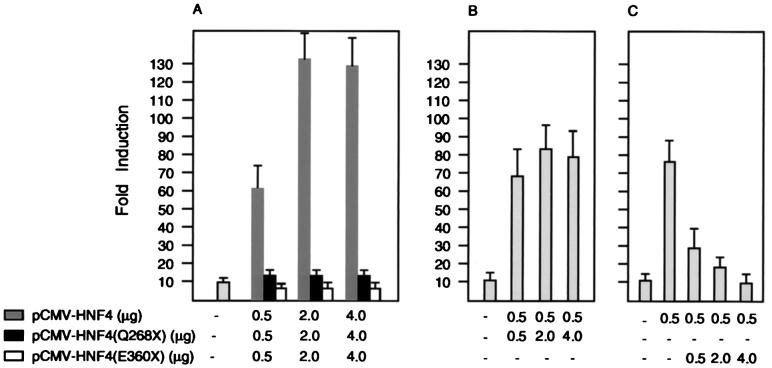
Transient expression and transcriptional activity of HNF4α and the C-terminal deletion mutants (Q268X) and (E360X) in HepG2 cells. (A) The reporter plasmid pZLHIV-A1-4 was cotransfected into HepG2 cells with CMV-LacZ plasmid and effector plasmids expressing HNF4α and the indicated deletion mutants. Cells were harvested 48 hr later and assayed for luciferase and β-galactosidase activities. The average fold inductions from two independent transfections done in duplicate and normalized to β-galactosidase activity are shown. (B and C) Cotransfections as described for A but with a constant amount of vectors CMV-HNF4 and an increasing amount of CMV-HNF4(Q268X) (B) or CMV-HNF4(E360X) (C) as indicated. Error bars indicate range.
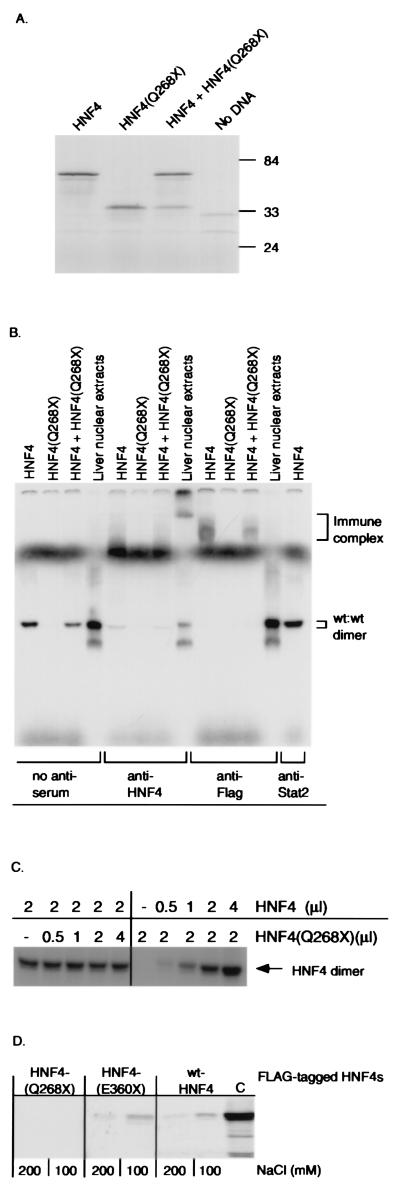
Expression, DNA binding, and dimerization properties of HNF4α and deletion mutant (Q268X). (A) SDS/PAGE analysis of in vitro-translated wild-type HNF4α and mutant HNF4(Q268X), followed by autoradiography. Numbers indicate molecular mass protein markers in kDa. (B) EMSA analysis of DNA binding activity of in vitro-translated HNF4α and deletion mutant (Q268X) (Top) by using the 32P-labeled double-stranded oligonucleotide LF-A1 as a probe. Supershift analysis was carried out with a polyclonal anti-HNF4 antiserum, an anti-Flag mAb, or an anti-STAT-2 control antiserum. (C) EMSA analysis of DNA binding activity of in vitro-translated wild-type HNF4α with increasing amounts of HNF4(Q268X) protein. (D) Both in vitro-translated wild-type HNF4 and HNF4(E360X), but not HNF4(Q268X), dimerize with HNF4. FLAG-tagged HNF4(Q268X), HNF4(E360X), or wild-type HNF4α bound to a anti-FLAG mAb attached to agarose was incubated with [35S]-methionine-labeled HNF4α protein. Immunoprecipitates were run on a 10% SDS/PAGE and bound HNF4α was detected by autoradiography. Each reaction was performed in buffers containing 100 and 200 mM NaCl, respectively (32). (C) 35S-methionine-labeled HNF4α.
To determine if the loss of transcriptional activity was caused by an inability to bind DNA, we compared the ability of wild-type and mutant HNF4α proteins to interact with a high-affinity HNF4 binding site (LF-A1) by EMSA analysis (1). Wild-type and mutant proteins, epitope-tagged at their C-termini, were produced in vitro by using the Promega TNT transcription/translation system (Fig. (Fig.22A). Fig. Fig.22B shows that wild-type HNF4α binds strongly to DNA, whereas the mutant HNF4α(Q268X) has lost its DNA binding activity. Because endogenous HNF4α is known to bind to DNA as a homodimer (3) EMSA analysis was performed to detect any heterodimer formation between wild-type and mutant proteins. Equal molar amounts of in vitro translated HNF4α and HNF4(Q268X) proteins were mixed and assayed for binding to the probe. The only complex detected migrated with an electrophoretic mobility identical to the wild-type HNF4α homodimer (Fig. (Fig.22B). Furthermore, excess amounts of HNF4(Q268X) protein did not reduce the DNA binding activity of wild-type HNF4α homodimers (Fig. (Fig.22C). The presence of HNF4α protein in the DNA binding complex was confirmed by supershift analysis by using anti-HNF4 and anti-Flag antibodies (Fig. (Fig.22B) (17). The inability of the HNF4(Q268X) protein to interact with wild-type HNF4α also was confirmed by coimmunoprecipitation experiments. FLAG-tagged HNF4(Q268X) did not bind to wild-type [35S]-methionine-labeled HNF4α, whereas both FLAG-tagged HNF4 and HNF4(E360X) did (Fig. (Fig.22D). The lack of binding activity, loss of transcriptional activation, and the inability to heterodimerize with wild-type HNF4α in vivo strongly suggest that the MODY1 HNF4(Q268X) mutation leads to a loss of HNF4α function. This implies that a reduction in the amount of HNF4α per se is responsible for the MODY1 phenotype.
Loss of Function of HNF4α Leads to Impaired Expression of Genes Involved in Glucose Transport and Glycolysis.
The molecular mechanisms by which a reduction of HNF4α activity results in an autosomal dominant form of type 2 diabetes are unknown. Glucose-stimulated insulin secretion (11) is impaired in MODY1 patients, suggesting that gene regulation in the pancreatic β-cell is responsible for their hyperglycemia. HNF4α null mice die during development before formation of the pancreas and so cannot be used to identify HNF4-regulated genes in the pancreatic β-cells (4). However, the VE, which expresses HNF4α (ref. 5; Fig. Fig.33 A and B), displays many characteristics of both the liver and endocrine pancreas and can be used to analyze HNF4α regulated gene expression (9). When ES cells are grown in suspension in the absence of lymphocyte inhibitory factor they differentiate to form EBs, which contain VE (Fig. (Fig.3,3, arrow). Moreover the VE expresses many genes that regulate glucose metabolism, including glucose transporter 2 (GLUT2) and aldoB (refs. 12, 19, and 20; Fig. Fig.33 C and D). Because ES cells are amenable to genetic manipulation this allowed us to test if loss of function of HNF4α leads to an impairment of gene expression of key components of the glucose-stimulated insulin secretion signaling pathway in vitro. Gene expression in differentiated wild-type, heterozygous. and null HNF4α (HNF4 +/+, +/−, −/−) EBs was assayed by reverse transcriptase–PCR (9). Each sample used similar amounts of mRNA as shown by the equal amplification of hypoxanthine phosphoribosyltransferase (HPRT) mRNA. EBs produced from the HNF4α null ES cell lines, A8, B9 and B13, did not express HNF4α mRNA (Fig. (Fig.44A). Steady-state mRNA levels of VE markers Gata-4 (21) and vHNF-1 also were measured to demonstrate that each EB preparation had similar amounts of VE (Fig. (Fig.44A; data not shown). To identify downstream target genes of HNF4α we studied the expression of 60 genes that are known to have essential functions in insulin secretion in the pancreatic islet and glucose metabolism in the liver. Genes tested in the expression screen included glucose transporters, enzymes of glucose and fatty acid metabolism, intracellular storage proteins, ion channels, and G-protein coupled receptors of the seven transmembrane spanning superfamily. Analysis of gene expression that gave the most striking results are shown in Fig. Fig.4.4. Four genes involved in glucose transport and metabolism, glucose transporter 2 (GLUT2), aldoB, glyceraldehyde-3-phosphate dehydrogenase (1,3 BGD), and liver pyruvate kinase (L-PK) were significantly reduced or absent. In addition, the liver and intestinal specific fatty acid binding proteins (FABP-L, FABP-I), and the cellular retinol binding protein 2 (CREBII) also were down-regulated relative to HNF4 +/+ or +/− EBs. To determine if the dysregulation of these genes in the absence of HNF4α in EBs also holds true in vivo, we collected E.8.5 embryos from crosses of HNF4+/− mice and assayed VE gene expression in normal or HNF4 mutant mice. Again, HPRT expression showed that equivalent amounts of starting material were used in each sample. Gata-4 mRNA levels were found at higher levels in HNF4−/− embryos than in embryos expressing HNF4α, reflecting the fact that the ratio of VE cells to cells of other embryonic lineages is greater in HNF4−/− embryos as has been described previously (4, 9). Expression analyses of target genes in HNF4α-deficient embryos confirmed the results obtained from the ES cell lines and suggests that down-regulation of these genes in vivo may be even more dramatic than in vitro (Fig. (Fig.44B).
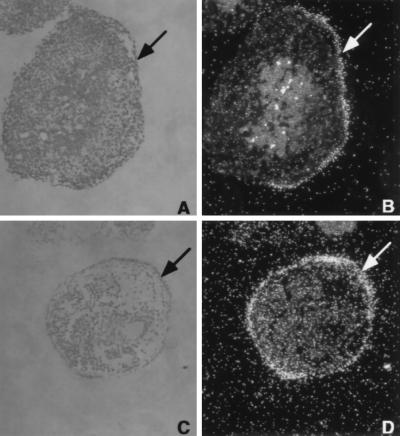
VE-specific expression of HNF4α and aldoB. Morphological section of HNF4+/+ EBs and in situ hybridization with antisense RNA of HNF4α (B) and aldoB (D) probe. Phase contrast micrograph of 5-μm sections through 14 days ES cell EBs, stained with hematoxylin and eosin (A and C), and the corresponding dark field on the right (B and D). The cuboidal epithelium of the VE is identified by an arrow.
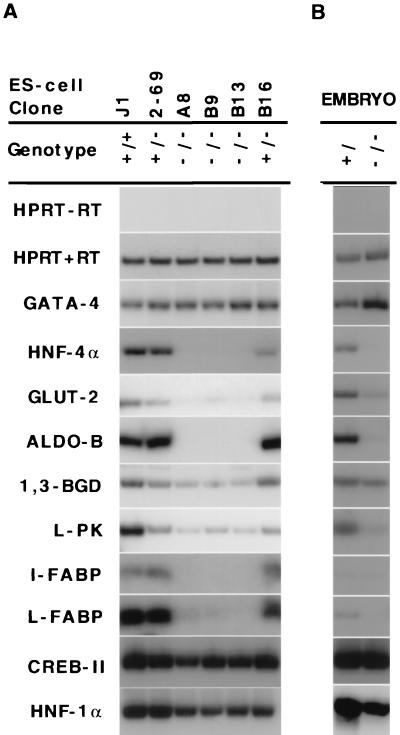
HNF4 regulates gene expression of genes involved in glucose transport and glucose metabolism in vitro and in vivo. (A) HNF4+/+ (J1), HNF4 +/− (2–69 and B16), and HNF4−/− (A8, B9, B13) ES-cell EBs were assayed for the presence of mRNAs derived from genes encoding glucose transporters, enzymes of glycolysis, and intracellular storage proteins. (B) Steady-state mRNA levels of HNF4α target genes were measured in E8.5 HNF4+ and HNF4−/− embryos by reverse transcriptase-PCR. Because HNF4−/− embryos have a block in gastrulation, a greater proportion of the starting material in HNF4−/− embryos is VE tissue, as confirmed by the greater levels of Gata-4 mRNA.
Expression of Genes Encoding Glycolytic Enzymes in the VE Is Glucose Responsive.
Hormones and dietary factors, such as glucose, play an important role in aldoB and L-PK gene expression in the liver. We have shown that the VE is a powerful system to study gene regulation of metabolic enzymes. To test if gene regulation in the VE also is modulated by glucose, we incubated EBs of HNF4+/+ and HNF4−/− cells in increasing concentrations of glucose for 6 hr. Steady-state mRNA levels were assayed for HPRT, Gata-4, aldoB, and L-PK. Gene expression of aldoB and L-PK was induced by high glucose concentrations in the culture medium, whereas expression of HPRT and Gata-4 was unchanged. As before, L-PK and aldoB gene expression was significantly reduced in the absence of HNF4α regardless of glucose concentrations (Fig. (Fig.5).5). These data show that gene transcription of aldoB and L-PK in the VE is physiological and can be modulated by nutrient supply analogous to what has been described in the adult liver (22).
DISCUSSION
Genetic studies have shown that mutations in HNF4α result in an autosomal dominant form of non-insulin-dependent diabetes mellitus characterized by a defect in glucose-stimulated insulin release. The mechanism by which a nonsense mutation in HNF4α, which results in the synthesis of a protein of 267 amino acids with an intact DNA-binding domain but a truncated dimerization and putative ligand domain, causes pancreatic β-cell dysfunction is not known. HNF4α exists as a homodimer in solution and can bind to its recognition site only after dimerization. To study if the HNF4α mutation causes diabetes by a loss-of-function or a dominant-negative mechanism, we expressed mutant and wild-type HNF4α and studied DNA binding and transcriptional activation of these proteins. Our results show that the mutant HNF4α protein, although stable, does not bind to HNF4 binding sites as a homodimer or heterodimer. Furthermore, the mutant protein exhibits no transcriptional transactivation activity and does not interact with the wild-type HNF4α in a dominant-negative fashion. We therefore conclude that the HNF4(Q268X) mutation is likely to result in a complete loss of function.
The loss of function of the mutant MODY1 HNF4 protein implies that a haploinsufficiency of HNF4α results in decreased expression of essential genes of pancreatic islet and liver function. The secretion of insulin is controlled by the rate of glucose entry into the β-cells and the rate of glucose metabolism of which glycolysis represents the major pathway in pancreatic β-cells (23, 24). In contrast to humans, HNF4+/− mice show no signs of diabetes and exhibit normal glucose tolerance (M.S., unpublished work). Furthermore, the embryonic lethality associated with HNF4−/− embryos prevented an analysis of HNF4α regulation of liver and pancreatic β-cell function. However, we have demonstrated that the VE provides a physiological system for the genetic dissection of metabolic pathways. By using this we identified four genes acting on different levels in the insulin secretion signaling pathway that are down-regulated in the absence of HNF4α. The mechanisms by which HNF4α regulates expression of these genes are unknown but are likely to be both direct and indirect. Some of these genes are known to contain HNF4α binding sites and therefore may be directly regulated by HNF4α (e.g., L-PK) (2, 25). It has been postulated that HNF4α may mediate its regulation through a transcriptional cascade involving HNF1α (26–28), however, we have found that HNF1α mRNA levels are at most only moderately affected by the complete absence of HNF4α. Our data also suggests that the defect in transcriptional activation in MODY1 patients is not mediated entirely through HNF1α because it has been reported that aldoB expression is not altered in the absence of HNF1α (29). Moreover, we did not detect transcriptional down-regulation of genes known to contain HNF1α binding sites such as the sodium/glucose cotransporter of the kidney and phenylalanine-hydroxylase (30).
In summary, we have shown that HNF4α regulates the transcription of genes involved in glucose entry and glycolysis in the VE cells both in vitro and in vivo. This pathway has been shown to be impaired in another form of early-onset type 2 diabetes (MODY2), which has mutations in the enzyme encoded by the glucokinase gene (31). Glycolytic flux in pancreatic β-cells constitutes the major pathway for the generation of metabolic signals that determine insulin secretion. Our data indicate that HNF4α is a pleiotropic regulator of the glycolytic pathway and glucose transport that offers a pathomolecular mechanism for the development of MODY1. Moreover, the availability of HNF4−/− VE allows the identification of additional HNF4α regulated-genes that may have important roles in diabetes. Identified genes can be tested in the future in islet cells and hepatocytes in vivo once suitable animal models such as tissue-specific knockout mice have been developed.
Acknowledgments
We thank G. I. Bell for his guidance and mentorship over many years and for encouraging us to work on early-onset type 2 diabetes; J. E. Darnell, Jr. for comments and for sharing the environment to initiate this work, and F. Sladek for providing reagents. M.S. is an Irma Hirschl Scholar, Pew Scholar, and Robert and Harriet Heilbrunn Professor, and S.A.D. is a Naomi Judd American Liver Scholar and an Alexandrine and Alexander Sinsheimer Scholar. This work was supported by the American Diabetes Association.
Footnotes
This paper was submitted directly (Track II) to the Proceedings Office.
Abbreviations: MODY, maturity-onset diabetes of the young; HNF, hepatocyte nuclear factor; EMSA, electrophoretic mobility-shift assay; ES, embryonic stem; CMV, cytomegalovirus; VE, visceral endoderm; EB, embryoid body; HPRT, hypoxanthine phosphoribosyltransferase; L-PK, liver pyruvate kinase; aldoB, aldolase B.
References
Articles from Proceedings of the National Academy of Sciences of the United States of America are provided here courtesy of National Academy of Sciences
Full text links
Read article at publisher's site: https://doi.org/10.1073/pnas.94.24.13209
Read article for free, from open access legal sources, via Unpaywall:
https://europepmc.org/articles/pmc24288?pdf=render
Citations & impact
Impact metrics
Citations of article over time
Alternative metrics
Article citations
DNA methylation and type 2 diabetes: a systematic review.
Clin Epigenetics, 16(1):67, 16 May 2024
Cited by: 1 article | PMID: 38755631 | PMCID: PMC11100087
Review Free full text in Europe PMC
Functional characterization of HNF4A gene variants identify promoter and cell line specific transactivation effects.
Hum Mol Genet, 33(10):894-904, 01 May 2024
Cited by: 0 articles | PMID: 38433330 | PMCID: PMC11070132
FoxK1 associated gene regulatory network in hepatic insulin action and its relationship to FoxO1 and insulin receptor mediated transcriptional regulation.
Mol Metab, 78:101825, 16 Oct 2023
Cited by: 0 articles | PMID: 37852413 | PMCID: PMC10641274
Transcriptional regulation of metabolism in the intestinal epithelium.
Am J Physiol Gastrointest Liver Physiol, 325(6):G501-G507, 03 Oct 2023
Cited by: 0 articles | PMID: 37786942
Review
Multiple roles and regulatory mechanisms of the transcription factor HNF4 in the intestine.
Front Endocrinol (Lausanne), 14:1232569, 10 Aug 2023
Cited by: 5 articles | PMID: 37635981 | PMCID: PMC10450339
Review Free full text in Europe PMC
Go to all (223) article citations
Other citations
Data
Similar Articles
To arrive at the top five similar articles we use a word-weighted algorithm to compare words from the Title and Abstract of each citation.
Hepatocyte nuclear factor 4alpha regulates the expression of pancreatic beta -cell genes implicated in glucose metabolism and nutrient-induced insulin secretion.
J Biol Chem, 275(46):35953-35959, 01 Nov 2000
Cited by: 128 articles | PMID: 10967120
Hepatocyte nuclear factor-4alpha involved in type 1 maturity-onset diabetes of the young is a novel target of AMP-activated protein kinase.
Diabetes, 50(7):1515-1521, 01 Jul 2001
Cited by: 101 articles | PMID: 11423471
Functional characterization of the HNF4alpha isoform (HNF4alpha8) expressed in pancreatic beta-cells.
Biochem Biophys Res Commun, 329(3):984-990, 01 Apr 2005
Cited by: 28 articles | PMID: 15752752
Roles of HNF1α and HNF4α in pancreatic β-cells: lessons from a monogenic form of diabetes (MODY).
Vitam Horm, 95:407-423, 01 Jan 2014
Cited by: 31 articles | PMID: 24559927
Review