Abstract
Objective
Glucagon-like peptide-1 (GLP-1) promotes glucose homeostasis through regulation of islet hormone secretion, as well as hepatic and gastric function. Because GLP-1 is also synthesized in the brain, where it regulates food intake, we hypothesized that the central GLP-1 system regulates glucose tolerance as well.Research design and methods
We used glucose tolerance tests and hyperinsulinemic-euglycemic clamps to assess the role of the central GLP-1 system on glucose tolerance, insulin secretion, and hepatic and peripheral insulin sensitivity. Finally, in situ hybridization was used to examine colocalization of GLP-1 receptors with neuropeptide tyrosine and pro-opiomelanocortin neurons.Results
We found that central, but not peripheral, administration of low doses of a GLP-1 receptor antagonist caused relative hyperglycemia during a glucose tolerance test, suggesting that activation of central GLP-1 receptors regulates key processes involved in the maintenance of glucose homeostasis. Central administration of GLP-1 augmented glucose-stimulated insulin secretion, and direct administration of GLP-1 into the arcuate, but not the paraventricular, nucleus of the hypothalamus reduced hepatic glucose production. Consistent with a role for GLP-1 receptors in the arcuate, GLP-1 receptor mRNA was found to be expressed in 68.1% of arcuate neurons that expressed pro-opiomelanocortin mRNA but was not significantly coexpressed with neuropeptide tyrosine.Conclusions
These data suggest that the arcuate GLP-1 receptors are a key component of the GLP-1 system for improving glucose homeostasis by regulating both insulin secretion and glucose production.Free full text

Arcuate Glucagon-Like Peptide 1 Receptors Regulate Glucose Homeostasis but Not Food Intake
Abstract
OBJECTIVE—Glucagon-like peptide-1 (GLP-1) promotes glucose homeostasis through regulation of islet hormone secretion, as well as hepatic and gastric function. Because GLP-1 is also synthesized in the brain, where it regulates food intake, we hypothesized that the central GLP-1 system regulates glucose tolerance as well.
RESEARCH DESIGN AND METHODS—We used glucose tolerance tests and hyperinsulinemic-euglycemic clamps to assess the role of the central GLP-1 system on glucose tolerance, insulin secretion, and hepatic and peripheral insulin sensitivity. Finally, in situ hybridization was used to examine colocalization of GLP-1 receptors with neuropeptide tyrosine and pro-opiomelanocortin neurons.
RESULTS—We found that central, but not peripheral, administration of low doses of a GLP-1 receptor antagonist caused relative hyperglycemia during a glucose tolerance test, suggesting that activation of central GLP-1 receptors regulates key processes involved in the maintenance of glucose homeostasis. Central administration of GLP-1 augmented glucose-stimulated insulin secretion, and direct administration of GLP-1 into the arcuate, but not the paraventricular, nucleus of the hypothalamus reduced hepatic glucose production. Consistent with a role for GLP-1 receptors in the arcuate, GLP-1 receptor mRNA was found to be expressed in 68.1% of arcuate neurons that expressed pro-opiomelanocortin mRNA but was not significantly coexpressed with neuropeptide tyrosine.
CONCLUSIONS—These data suggest that the arcuate GLP-1 receptors are a key component of the GLP-1 system for improving glucose homeostasis by regulating both insulin secretion and glucose production.
The importance of gastrointestinal hormones signaling gut absorption of carbohydrates and downstream processes involved in glucose disposal has received increasing attention. Prominent among these is glucagon-like peptide-1 (GLP-1), which is produced by L-cells of the ileum and is secreted during meal ingestion. GLP-1 augments nutrient-induced insulin release (1,2), inhibits glucagon release (3), slows gastric emptying (4), and has islet-independent effects to reduce hepatic glucose production (5–8). Studies in animals and humans have demonstrated that GLP-1 signaling is necessary for normal glucose tolerance (9). Moreover, two newly approved therapies for type 2 diabetic patients act through GLP-1 signaling to improve glucose homeostasis.
Most of the evidence demonstrating a role for GLP-1 in glucose homeostasis has focused on actions within the pancreatic islet. However, GLP-1 is also synthesized in a discrete population of neurons in the hindbrain (10–12), and GLP-1 receptors are highly expressed in various regions of the hypothalamus (13) including the arcuate nucleus (ARC) and the paraventricular nucleus (PVN) (14), two areas where immunoreactive GLP-1 fibers terminate (11). Central nervous system (CNS) GLP-1 receptors have been linked to the control of food intake, endocrine and behavioral responses to stress, and visceral illness (15–17). Although there is evidence that circulating GLP-1 agonists can activate CNS neurons (18) and that GLP-1 may cross the blood-brain barrier (19), central and peripheral GLP-1 signaling systems are generally held to be separate.
Compelling recent evidence links a number of CNS systems to the regulation of peripheral glucose levels. While hypothalamic areas such as the PVN and the dorsal medial and the ventromedial hypothalamus may play a role in glucose homeostasis during stress (20–22), there is strong evidence that the ARC plays a key role in maintaining normal glucose levels in response to anorectic peptides or nutrients by regulation of glucose production (23–26). Given this emerging evidence for CNS involvement in the regulation of peripheral metabolism and the broad role that peripheral GLP-1 signaling plays in regulating glucose homeostasis, we hypothesized that CNS GLP-1 receptors would have multiple coordinated effects to improve glucose tolerance. Specifically, we focused on the ARC because GLP-1 receptors are found in this region, and previous studies have shown that neurons in this area regulate glucose production. Thus, a second hypothesis was that ARC GLP-1 receptors regulate glucose output. Finally, using dual in situ hybridization histochemistry, we evaluated ARC GLP-1 receptor expression on orexigenic neuropeptide tyrosine (NPY) and anorexigenic proopiomelanocortin (POMC) neurons.
RESEARCH DESIGN AND METHODS
Male Long-Evans rats (300–350 g) from Harlan (Indianapolis, IN) were housed in the University of Cincinnati Laboratory Animals for Medical Science Facility at the Genome Research Institute under controlled conditions (12:12 light-dark cycle, 50–60% humidity, and 25°C) with free access to rodent food and water. All procedures for animal use were approved by the University of Cincinnati Institutional Animal Care and Use Committee.
One week before the study, under anesthesia (isoflourine), rats had catheters placed in the carotid artery and jugular vein. For some studies, a stainless steel cannula was placed in the third cerebral ventricle (i3vt) (22 gauge, 11 mm, and i3vt; coordinates: 2.2 A/P and 7.5 D/V), the ARC (26 ga and 11 mm; coordinates: 1.8 mol/l/l; 3.0 A/P and 8.6 D/V, at 10°) or the PVN (26 ga and 11 mm; coordinates: 0.2 mol/l/l; 2.2 A/P and 7.5 D/V) of the brain using stereotaxic (ASI Instruments, Warren, MI) coordinates as determined by the atlas of Paxinos and Watson (27). Rats were studied after regaining at least 90% of presurgery body weight (~1 week). An additional set of animals had cannulas placed in the ARC or PVN, as described above, but without catheters. The i3vt cannula placement was verified in rats drinking ≥5 ml water 1 h after CNS injection of angiotensin II. Postmortem cannula placement for rats implanted with the ARC or PVN cannulas was verified after toluidine blue injection into the cannula. The brains were removed, fresh frozen, and subsequently cut with a cryostat set at 30 μm. The dye was then visualized on brain slices using a microscope. Only rats with correct placement of cannulas (Fig. 5) were included in the final analyses.
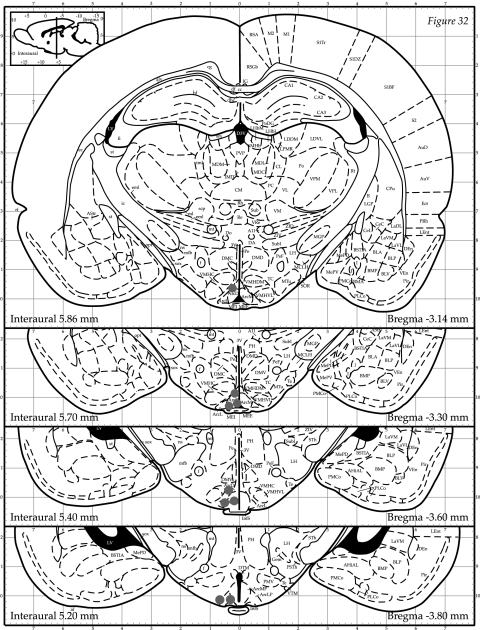
ARC and PVN cannula-placement sites. After completion of the hyperinsulinemic-euglycemic clamps, the animals were killed and toludine blue dye was injected into the cannula. The brain was then sectioned and placement was verified. Black dots represent correct cannula placement. Rats with cannulas not placed in the ARC and PVN were excluded from the analysis (n = 8).
Intraperitoneal glucose tolerance tests.
Eight overnight-fasted rats received a i3vt injection (50 μg; 2 μl/15 s) of a potent GLP-1 receptor antagonist, des-His1,Glu8-exendin-4 (dH-EX) (n = 4 [28,29]) or saline (n = 4). Five minutes later, an intraperitoneal injection of 50% dextrose (2 g/kg) was given. Blood samples were taken from the tail at baseline and at 15, 30, 45, 60, and 120 min and at 0, 15, 30 and 60 min after the intraperitoneal injection for glucose and insulin assessment, respectively.
Intravenous glucose tolerance tests.
Overnight-fasted rats received an i3vt injection of GLP-1 (3 μg in 2 μl/15 s; n = 8) or saline (n = 7), followed 5 min later by an intravenous bolus of 20% dextrose (0.5 mg/kg). Blood samples for glucose and insulin were taken at baseline (−10 and −6 min) and every 2 min from −2 through 10 min and then at 20 and 30 min after the intravenous glucose infusion.
Hyperinsulinemic-euglycemic clamp experiments.
Overnight-fasted rats were conscious and unrestrained during the experimental period. The morning of the study, rats were weighed and the exteriorized catheters were extended for ease of access. To measure glucose kinetics, a primed (30 μCi) constant (0.3 μCi/min) infusion of high-performance liquid chromatography–purified [3-3H]glucose (Perkin Elmer Life Sciences, Boston, MA) was administered via a precalibrated infusion pump (Harvard Apparatus, South Natick, MA) at 0–270 min. At 120–270 min, GLP-1 was infused into either the i3vt (n = 7), the ARC (n = 7), or the PVN (n = 7) at 0.01 μg/min, or saline was infused into either the ARC (n = 7) or PVN (n = 7) at 0.1 μl/min. In another group of animals, GLP-1 plus glibenclamide (200 pmol total intra-ARC dose [25]), an ATP-sensitive K+ channel (KATP channel) blocker, was coinfused into the ARC to determine the role of these channels in GLP-1–induced changes in glucose kinetics. From 150 to 270 min, a primed (30 pmol · kg−1 · min−1), continuous (15 pmol · kg−1 · min−1) infusion of insulin (Eli Lilly, Indianapolis, IN) was administered via a precalibrated infusion pump (Harvard Apparatus, South Natick, MA). This insulin infusion rate was used because our preliminary data showed that it did not completely suppress glucose production, allowing the determination of an effect of CNS GLP-1 on both glucose production and glucose uptake. A variable rate 50% dextrose infusion maintained blood glucose at ~5.6 mmol/l. During the clamp, the [3-3H]glucose infusion rate was increased to 0.6 μCi/min to maintain constant specific activity. During the experimental period, blood was drawn every 5–15 min for measurements of blood glucose, every 10 min during the basal period, and every 15 min during the experimental periods for [3-3H]glucose and at times 120, 150, and 270 min for plasma insulin levels.
Tracer calculations.
Rates of glucose appearance (Ra), glucose production, and glucose utilization were calculated according to previous methods (30,31). Briefly, endogenous glucose production was calculated by determining the total Ra (this comprises both glucose production and any exogenous glucose infused to maintain the desired glycemic levels) and subtracting it from the amount of exogenous glucose infused.
Anatomical localization of ARC GLP-1 receptor–expressing cells.
Brains from male Long-Evans and Sprague-Dawley rats (250–300 g) were frozen and sectioned (12 μm) on a cryostat. The following plasmids were used for probe synthesis: 1) for GLP-1 receptors, the full-length rat sequence including 83 bp of the 3′ untranslated region was inserted into pBSII KS (Stratagene, San Diego, CA); 2) for POMC, a 900-bp fragment was inserted into pGEM4z (Promega, Madison WI); and 3) for NPY, a full-length fragment was inserted into pBSSK (Stratagene). For generating antisense cRNAs, GLP-1 receptors and NPY plamids were linearized with HindIII, and T7 polymerase was used for in vitro transcription. POMC plasmid was linearized with EcoRI, and T3 polymerase was used for in vitro transcription to generate antisense cRNA. Sense and antisense 33P-radiolabeled (GLP-1 receptors) or digoxigenin-labeled (POMC and NPY) cRNAs were generated using standard manufacturer protocols and added to a final concentration of 16 × 106 cpm and 800–1,600 ng/slide, respectively. Double in situ hybridization histochemistry was performed according to previously published protocols (21,32).
Three-dimensional serial section reconstruction and neuron mapping.
Microscopic examinations were made on an Olympys BX51 connected to a digital camera CX9000 (MBF Biosciences) controlled by Stereoinvestigator software (version 7.51.1; MBF Biosciences). Pictures were acquired under 10× and 40× objectives, and anatomical maps were generated using the 40× objective under simultaneous brightfield and lateral darkfield illumination using a Darklite illuminator (Micro Video Instruments, Avon, MA).
Ten sections spanning the retrochiasmatic nucleus and the ARC were used to generate quantitative data as well as three-dimensional anatomical reconstructions from three rats. Both sides of the ARC were analyzed. Each section was separated by 240 μm. Anatomical maps were generated for each rat using Stereoinvestigator. Each section was acquired using the serial section module aligned to previous sections, and colored symbols were used to represent neurons. Single- and double-labeled neurons for POMC and GLP-1 receptors were traced for each section. Cells were counted as positive for GLP-1 receptor mRNA when the number of silver grain accumulated over the cell body was at least 5× above the background (area devoid of cells, e.g., the corpus callosum). For digoxigenin staining, cells were counted only if they displayed a clear cellular morphology and their size was within the range of average size of cell bodies within this area. When a cluster of cells was encountered, only cells with a clearly visualized nucleus were scored. Double-labeled cells were scored when the silver-grain accumulation confined to the digoxigenin labeling was at least 5× above background. Three-dimensional model graphics were generated using the solid-modeling module and rotated using Neurolucida Explorer (version 4.50.4; MBF Biosciences).
Food intake.
Food was removed 3 h before dark onset. Immediately before dark onset, rats with cannulas directed at the ARC (unilateral, n = 35; bilateral, n = 18) or PVN (n = 11) were injected with 0.3 μg · 0.1 μl−1 · 1−1 · min−1 GLP-1 or 0.1 μl/1 min saline using a precalibrated infusion pump (Harvard Apparatus, South Natick, MA). This dose of GLP-1 was chosen because it is less than the lowest effective dose found with i3vt administration of GLP-1 (3 μg [17]) and similar to previous site-specific doses of GLP-1 (33). Food was weighed at baseline and 0.5, 1, and 2 h after dark onset.
Analytical methods.
Blood glucose was measured using a Freestyle (Therasense, Almeda, CA) glucose analyzer. Insulin and glucagon were measured using radioimmunoassay techniques with a standard antiserum method for insulin (34) and a commercial kit for glucagon (Linco, St. Louis, MO). Plasma [3H]glucose radioactivity was measured using the Somogyi procedure, as previously described (31).
Statistical analysis.
The data were analyzed using paired and unpaired t tests and mixed-model ANOVAs where appropriate. Post hoc tests of individual groups were made using Tukey's post hoc tests. Statistical significance was set at P < 0.05 for all analyses.
RESULTS
Effect of CNS GLP-1 receptors on glucose homeostasis.
Rats injected with dH-EX in the i3vt were hyperglycemic for the first 45 min after the intraperitoneal glucose load compared with the saline-injected rats (Fig. 1A) and had a 50% increase in the glycemic response to intraperitoneal glucose, as represented by the area under the curve above baseline (P < 0.05). Despite these marked differences in plasma glucose during the intraperitoneal glucose tolerance test, there were no differences in insulin levels between the groups (Fig. 1B). The same dose of dH-EX that disrupted glucose homeostasis when given in the CNS had no effect when given peripherally (Table 1), and in a separate group of animals, we found that 20 min after an intraperitoneal glucose load, plasma GLP-1 levels did not significantly increase over baseline (n = 4; mean ± SE 43 ± 20 vs. 54 ± 36 pmol/l).
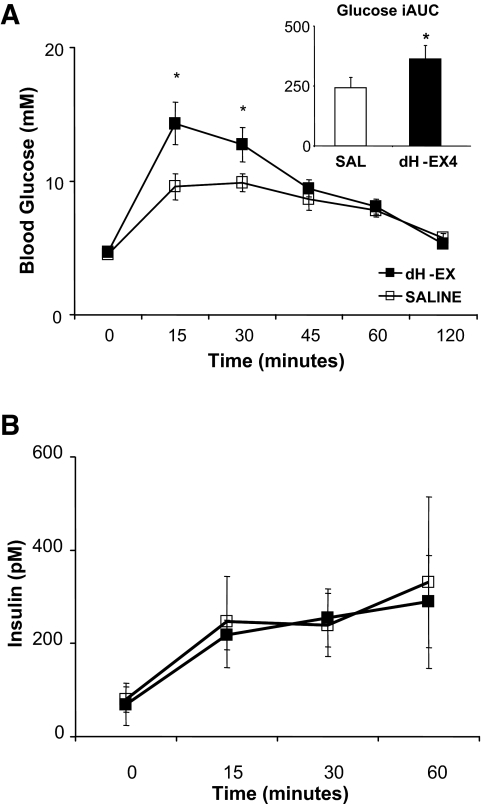
Effect of blocking CNS GLP-1 receptors on glucose (A) and insulin (B) responses to an intraperitoneal glucose injection. A: Glucose response to the intraperitoneal glucose load was significantly greater at 15 and 30 min after the glucose injection with dH-EX versus saline injected into the i3vt. Integrated area under the curve (iAUC) was also significantly greater in dH-EX versus saline (inlay). B: There were no significant differences between groups in the insulin response to the glucose load. *P 0.05 vs. saline.
TABLE 1
Percentage of double-labeled POMC and GLP-1 receptor neurons expressed in rat retrochiasmatic nucleus (RCh) and different areas of the ARC
Percent POMC neurons expressing GLP-1 receptor | Percent GLP-1 receptor neurons expressing POMC | |
---|---|---|
RCh | 40.0 ± 10.7 | 34.8 ± 14.9 |
Rostral ARC | 61.8 ± 4.5 | 50.6 ± 3.2 |
Mid-ARC | 68.5 ± 4.3 | 45.1 ± 3.5 |
Caudal ARC | 71.8 ± 3.7 | 35.8 ± 1.9 |
Total ARC | 68.1 ± 2.4 | 43.1 ± 2.1 |
Data are means ± SE. Ten sections per animals were used, and both sides of the retrochiasmatic nucleus and ARC for three animals were analyzed to generate these data.
CNS GLP-1 and insulin secretion.
To test whether the comparable plasma insulin concentrations during an intraperitoneal glucose load in dH-EX–and saline-treated rats in the presence of substantially different levels of glucose were due to CNS GLP-1 regulation of the β-cell, we administered GLP-1 into the i3vt before an intravenous glucose tolerance test (IVGTT). We found that despite similar glucose levels (Table 1), i3vt GLP-1 versus saline significantly increased insulin levels and insulin area under the curve (AUC) during the IVGTT (Fig. 2A), whereas when the same dose of GLP-1 was combined with intravenous saline, there was no significant effect on blood glucose (Table 1) or insulin levels (Fig. 2B). We used an IVGTT because in response to a single bolus of intravenous glucose, plasma glycemia in the first ~10 min is primarily a function of body compartment distribution rather than insulin action, and the rapid return to baseline (within 20 min) allowed us to isolate the effects of CNS GLP-1 on insulin secretion independently without the potential confounding influence of insulin-mediated glucose disposal. Furthermore, the fact that insulin levels increase over 10-fold more than baseline and return to baseline within 20 min suggests that a change in insulin clearance is an unlikely explanation for the increased insulin levels.
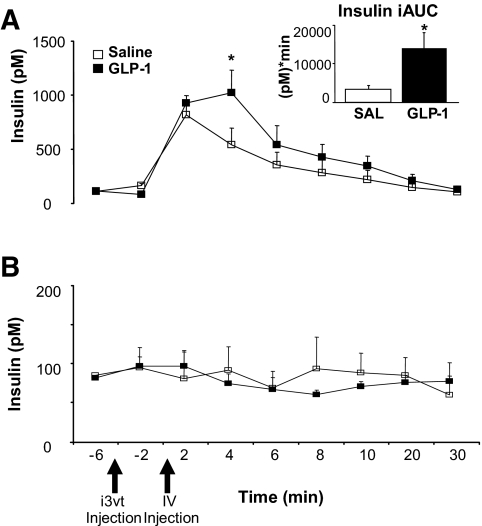
Insulin response to an intravenous glucose or saline bolus 5 min after i3vt GLP-1 or saline injection. A: Insulin levels were significantly greater at 4 min, and area under the curve for insulin response was significantly greater after GLP-1 (inset). B: Insulin levels did not significantly change with an intravenous saline bolus after i3vt GLP-1 and saline injections. *P < 0.05 vs. saline.
Expression of ARC GLP-1 receptor mRNA in NPY and POMC neurons.
As previously shown (14), we found numerous cells expressing GLP-1 receptors in the ARC (Fig. 3). Many of the GLP-1 receptor–containing cells also appeared to coexpress POMC mRNA. However, there was virtually no colocalization of GLP-1 receptor with NPY neurons, which were generally more medial and ventral compared with GLP-1 receptor mRNAs containing cells (Fig. 3A–C).
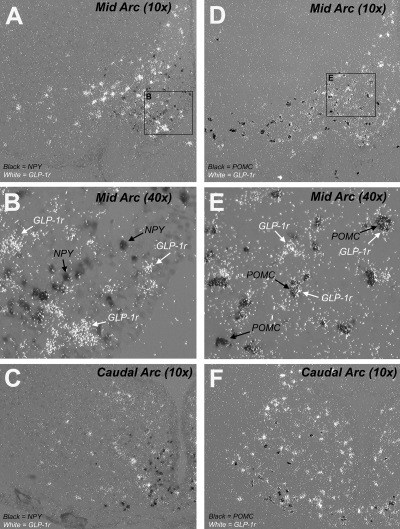
Photomicrographs illustrating the expression of GLP-1r mRNAs in NPY and POMC neurons in rat mid- and caudal ARC as detected by double in situ hybridization histochemistry. Neurons expressing GLP-1 receptor are visualized by a white, dense, silver-grain accumulation, while either NPY (A–C) or POMC (D–F) mRNAs appeared as a black precipitate in cell bodies. In the mid-ARC at low magnification (A and D), many GLP-1 receptor–expressing cells do not express NPY mRNA and are localized more laterally (A). Neurons expressing only GLP-1r are also visualized in the periventricular area posterior to both NPY and POMC neurons (A and D). A high number of POMC-expressing cells are found expressing GLP-1r mRNA characterized by strong silver-grain accumulation over the digoxigenin black precipitate and are clearly seen at higher magnification (F). In the caudal ARC (B and E), many neurons expressing the GLP-1 receptor but not NPY or POMC are found mostly in the posterior and periventricular part (B and E). C and F are photomicrographs at higher magnification of inserts represented by a black square in A and D.
In the retrochiasmatic nucleus, an average of 40.0 ± 10.7% of the POMC neurons also coexpressed GLP-1 receptor mRNA, whereas in the ARC, an average of 68.1 ± 2.7% of the POMC neurons located in the medio-lateral part of the nucleus expressed GLP-1 receptor as well (Table 2 and Fig. 3D–F). Overall, about half of the GLP-1 receptor–expressing cells did not express either NPY or POMC mRNA. These neurons were located mostly in the dorsal part of the ARC around the i3vt and, to a lesser extent, at the lateral edge of the nucleus (Figs. 3 and and4).4). These cells also seem to be more abundant in the caudal part of the ARC (Fig. 4).
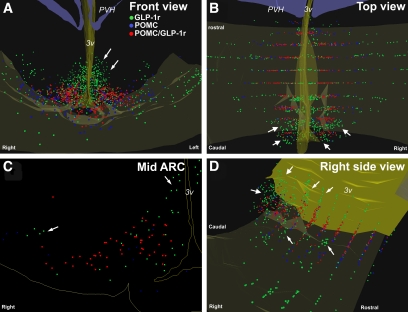
Illustrations of computer-assisted anatomical mapping and three-dimensional serial section reconstruction of POMC, GLP-1 receptors, and POMC/GLP-1 receptor–expressing cells in rat retrochiasmatic and arcuate nuclei. Anatomical maps were generated by drawing colored symbols over each cell type during examination of microscopic slides under a 40× objective. Green dots or squares, cells expressing GLP-1 receptor alone. Blue dots or triangles, cells expressing POMC alone. Red dots, cells expressing both GLP-1 receptor and POMC. 3v, third ventricle; PVH, paraventricular nucleus of the hypothalamus. (Please see http://dx.doi.org/10.2337/db07-1824 for a high-quality digital representation of this figure.)
TABLE 2
Blood glucose levels during an intraperitoneal glucose tolerance test (IPGTT) with intraperitoneally administered dH-EX or saline, after an IVGTT with i3vt GLP-1 or saline, and after intravenously administered saline plus i3vt GLP-1 or saline
GLP-1 | Saline | dH-EX | |
---|---|---|---|
IPGTT (min) | |||
![]() ![]() ![]() ![]() | 87 ± 3 | 90 ± 4 | |
![]() ![]() ![]() ![]() | 303 ± 22 | 269 ± 28 | |
![]() ![]() ![]() ![]() | 281 ± 19 | 248 ± 17 | |
![]() ![]() ![]() ![]() | 253 ± 16 | 211 ± 17 | |
![]() ![]() ![]() ![]() | 228 ± 16 | 185 ± 13 | |
IVGTT (min) | |||
![]() ![]() ![]() ![]() | 94 ± 4 | 96 ± 4 | |
![]() ![]() ![]() ![]() | 279 ± 20 | 245 ± 29 | |
![]() ![]() ![]() ![]() | 241 ± 10 | 224 ± 17 | |
![]() ![]() ![]() ![]() | 169 ± 16 | 161 ± 17 | |
![]() ![]() ![]() ![]() | 122 ± 13 | 115 ± 10 | |
Intravenous saline (min) | |||
![]() ![]() ![]() ![]() | 94 ± 7 | 92 ± 4 | |
![]() ![]() ![]() ![]() | 89 ± 8 | 95 ± 4 | |
![]() ![]() ![]() ![]() | 94 ± 7 | 93 ± 6 | |
![]() ![]() ![]() ![]() | 97 ± 10 | 91 ± 6 | |
![]() ![]() ![]() ![]() | 107 ± 12 | 91 ± 7 |
There were no statistical differences in glucose levels between intraperitoneal dH-EX and saline during the intraperitoneal glucose tolerance test or between i3vt GLP-1 versus saline with either intravenous glucose or saline.
The graphical three-dimensional representation of the ARC allowed us to clearly identify two distinct populations of POMC neurons. One population, found predominantly in the medio-lateral part of the ARC, coexpressed GLP-1 receptor mRNA. The other population was found at the lateral edge of the nucleus and largely did not coexpress GLP-1 receptors (Fig. 4A, B, and D). Note the abundance of GLP-1 receptor–expressing cells (green dots) in the periventricular and lateral edge of the ARC (Fig. 4A, C, and D). GLP-1 receptor–expressing cells are also more numerous in the caudal ARC (large arrows in Fig. 4B and D), specifically in its medial posterior part. While the in vivo experiments were performed in Long-Evans rats and the anatomical data were performed on brains of Sprague-Dawley rats, we have found similar patterns of distribution in GLP-1 receptors between the two strains (data not shown).
CNS GLP-1 and hyperinsulinemic-euglycemic clamps.
Basal insulin and corticosterone (Table 3) levels were similar among groups and did not change with intracerebroventricular infusions. Based on relatively unchanging plasma glucose concentrations and glucose specific activity (coefficients of variation 5.4 ± 0.6 and 5.7 ± 0.8% for basal and final 30 min, respectively), basal glucose production and glucose uptake were not significantly affected by CNS GLP-1 (37 ± 4, 42 ± 6, and 41 ± 4 μmol · kg−1 · min−1 for saline, ARC, and i3vt GLP-1, respectively).
TABLE 3
Plasma glucose, insulin, and corticosterone levels prior to and during the hyperinsulinemic-euglycemic clamp
ARC saline | ARC GLP-1 | i3vt GLP-1 | |
---|---|---|---|
Glucose (mmol/l) | |||
![]() ![]() ![]() ![]() | 4.9 ± 0.1 | 4.6 ± 0.1 | 4.9 ± 0.2 |
![]() ![]() ![]() ![]() | 4.6 ± 0.1 | 4.4 ± 0.5 | 4.9 ± 0.3 |
![]() ![]() ![]() ![]() | 5.2 ± 0.2 | 5.4 ± 0.3 | 5.3 ± 0.2 |
Insulin (pmol/l) | |||
![]() ![]() ![]() ![]() | 67 ± 21 | 69 ± 16 | 66 ± 13 |
![]() ![]() ![]() ![]() | 48 ± 10 | 60 ± 17 | 52 ± 11 |
![]() ![]() ![]() ![]() | 285 ± 59 | 295 ± 48 | 246 ± 37 |
Corticosterone (nmol/l) | |||
![]() ![]() ![]() ![]() | 13 ± 3 | 15 ± 3 | 13 ± 3 |
![]() ![]() ![]() ![]() | 17 ± 3 | 15 ± 3 | 13 ± 2 |
![]() ![]() ![]() ![]() | 13 ± 3 | 18 ± 2 | 16 ± 2 |
Data are means ± SE. Neither insulin nor corticosterone levels significantly changed over time in any group during the hyperinsulinemic-euglycemic clamp. ICV, intracerebroventricular.
Glucose and insulin levels were well matched among the groups during the clamps (Table 3). Insulin levels were comparable with those measured during the intraperitoneal glucose tolerance test (Fig. 1B), indicating that the levels of insulin were within physiological range. Basal glucose kinetics were similar among the groups (Table 4). During the clamp, the amount of glucose infused to maintain glycemic levels did not differ among the saline-, ARC-, and i3vt GLP-1–treated rats, indicative of similar rates of whole-body insulin sensitivity (Fig. 6A). However, ARC GLP-1 significantly inhibited glucose production (Fig. 6B) and glucose uptake (Fig. 6C) by the end of the clamp period. While the glucose production rates between saline and i3vt GLP-1 groups did not differ, the same low dose of GLP-1 infused into the i3vt as that infused into the ARC also inhibited glucose uptake. However, the same rate and concentration of GLP-1 (vs. saline) infused into the PVN did not alter glucose production or glucose uptake (Fig. 7A–C). Thus, these data indicate that increased GLP-1 signaling, specifically in the ARC, can produce significant changes in both glucose production and uptake. While the effects of central GLP-1 to lower glucose production appear to be specific for the ARC, the comparable reduction in glucose uptake with i3vt and ARC GLP-1 raises the possibility that other brain areas could mediate this process.
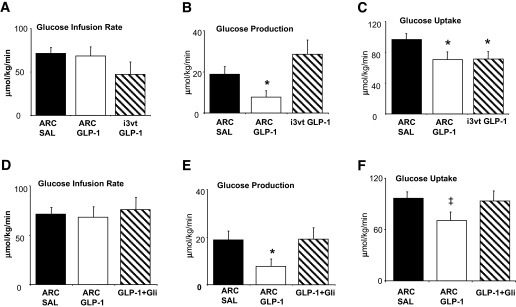
Effect of ARC GLP-1 on peripheral glucose homeostasis during a hyperinsulinemic-euglycemic clamp. A: Glucose infusion rate during the glucose clamp was similar between the three groups. B: Glucose production was significantly reduced in ARC GLP-1 versus both saline and i3vt GLP-1 groups. C: Glucose uptake was significantly reduced in ARC and i3vt GLP-1 vs. saline. D: Glucose infusion rate during the hyperinsulinemic-eulgycemic clamp was similar between saline, ARC GLP-1, and ARC GLP-1 plus glibenclamide. E: Coinfusion of glibenclamide into the ARC prevented GLP-1–induced inhibition of glucose production. F: Coinfusion of glibenclamide into the ARC prevented GLP-1–induced inhibition of glucose uptake. *P < 0.05 vs. saline. †P < 0.05 vs. saline and i3vt. ‡P < 0.05 vs. saline and GLP-1 plus glibenclamide.
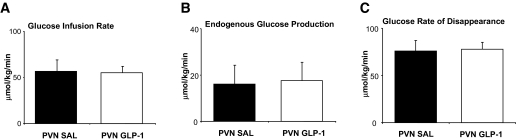
Effect of infusion of GLP-1 or saline into the PVN on glucose kinetics. A: Glucose infusion rate during the glucose clamp was similar between PVN saline and GLP-1 groups. B: Glucose production was similar between the PVN GLP-1 and saline groups. C: Glucose uptake was similar between the PVN GLP-1 and saline groups.
TABLE 4
Effect of ARC, PVN, and i3vt GLP-1 on basal glucose production and uptake
Saline | ARC GLP-1 | i3vt GLP-1 | GLP-1 plus glibenclamide | |
---|---|---|---|---|
Glucose production (μmol · kg−1 · min−1) | ||||
![]() ![]() ![]() ![]() | 37 ± 4 | 42 ± 6 | 41 ± 4 | 33 ± 4 |
![]() ![]() ![]() ![]() | 34 ± 3 | 35 ± 3 | 40 ± 4 | 30 ± 3 |
Glucose uptake (μmol · kg−1 · min−1) | ||||
![]() ![]() ![]() ![]() | 35 ± 5 | 42 ± 6 | 38 ± 2 | 33 ± 4 |
![]() ![]() ![]() ![]() | 31 ± 2 | 35 ± 3 | 36 ± 2 | 39 ± 3 |
Data are means ± SE. There were no significant effects of intra-ARC or i3vt GLP-1 on glucose production or uptake under basal conditions.
We infused glibenclamide, at a dose that has previously been shown to block insulin- and nutrient-induced decreases in glucose production (24,25), together with GLP-1 into the ARC during the hyperinsulinemic-euglycemic clamp. Glibenclamide had no effect on basal glucose and insulin levels, basal glucose kinetics (data not shown), or glucose infusion rate during the clamp (Fig. 6D) but, consistent with previous data, did block both effects of ARC GLP-1 on glucose production and glucose uptake (Fig. 6E and F).
Effect of ARC GLP-1 on food intake.
Neither unilateral nor bilateral injection of GLP-1 into the ARC significantly affected food intake (Fig. 8). However, as shown previously (33), unilateral administration of GLP-1 directly into the PVN significantly reduced food intake (Fig. 8).
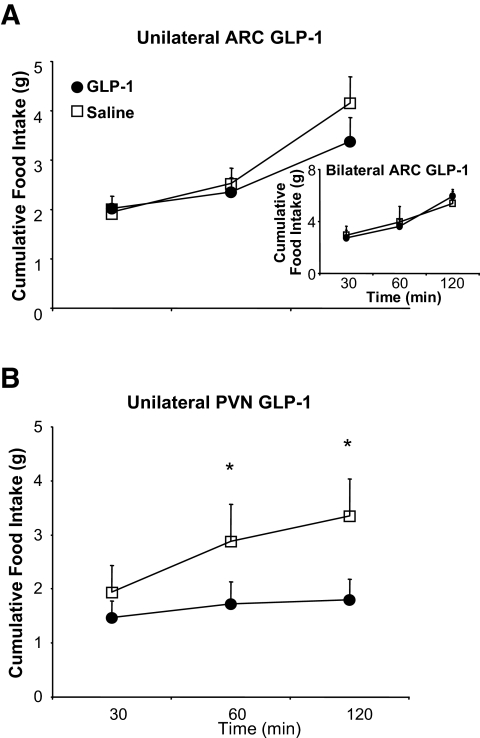
Effect of site-specific injections of GLP-1 on food intake. A: Cumulative food intake was similar after unilateral or bilateral (inset) ARC injection of GLP-1 or saline. B: Cumulative food intake was significantly reduced after 60 and 120 min of GLP-1 injection compared with an equal volume of saline injected directly into the PVN. *P < 0.05 vs. GLP-1.
DISCUSSION
Many current models hold that peripheral and CNS GLP-1 signaling operate as distinct systems, i.e., CNS GLP-1 to regulate food intake and circulating GLP-1 to control glucose homeostasis. Our data challenge this conventional model by demonstrating that CNS GLP-1 also plays an important role in regulating glucose tolerance through coordinate actions on the pancreas and liver.
Although it has long been known that the CNS is vital in the response to hypoglycemia, there has been less appreciation for the role of the brain to maintain homeostasis during glucose challenges. However, recent studies using insulin/glucose clamps have demonstrated that i3vt insulin administration increased peripheral insulin sensitivity (35) and inhibited glucose production (36). More specifically, restoration of ARC leptin receptors in leptin receptor–deficient mice improves insulin sensitivity independent of changes in food intake (37,38), and insulin, glucose, lactate, or long-chain fatty acid administration directly into the ARC each inhibits glucose production by ~30% more than vehicle during a somatostatin glucose clamp (26,36,39–41). Importantly, during clamped hyperglycemia/hyperinsulinemia, central GLP-1 signaling promoted hepatic glucose storage and insulin secretion while reducing muscle glucose uptake (42), the latter being an effect that was dependent on neural innervation of the muscle. These studies establish that CNS centers can affect key processes involved in glucose metabolism but do not address regulation of glucose homeostasis because they used experimental conditions (e.g., glucose clamps) in the absence of physiological elevations of glucose.
We chose an alternative approach. In our first experiment, we demonstrated that the composite actions initiated by GLP-1 receptor signaling in the brain promote glucose tolerance. Moreover, because we used a GLP-1 receptor antagonist to elicit this effect, our results indicate that this is a physiologic action of endogenous GLP-1. Interestingly, the degree of impairment of glucose tolerance with CNS GLP-1 receptor antagonism was comparable with that seen in mice with a whole-body targeted disruption of the GLP-1 receptor (9). Further, with an insulin infusion that produces postprandial levels, we found that ARC GLP-1, compared with saline, inhibited glucose production by ~30% more. Thus, our data extend previous observations concerning the importance of the ARC in CNS regulation of glucose levels to include an important role for GLP-1 signaling and also suggest that GLP-1 acting specifically in the ARC, but not in the PVN, acts similarly to insulin and nutrients to regulate glucose production (23–25,36,43). Furthermore, our data are suggestive that GLP-1 receptors on POMC or other non-NPY neurons play an important role in mediating this effect. Taken together, these findings suggest that GLP-1 signaling in the brain and the periphery has a role in normal glucose metabolism.
Little is known regarding GLP-1 signaling in the brain. In pancreatic β-cells, glucose inhibits ATP-sensitive K+ (KATP) channels, which causes cellular depolarization and insulin release. Similarly, glucose and insulin reduce KATP channel currents in lateral ARC neurons (44), and the effects of CNS administration of insulin (25) and nutrients (23,24) on glucose production can be abolished by glibenclamide, a KATP channel inhibitor. Our data add to this finding, as we found that coinfusion of glibenclamide blocked the effect of GLP-1 on both glucose production and glucose uptake. Thus, the KATP channels within the ARC are important for regulating overall glucose homeostasis.
Using in situ hybridization, we found a subset of ARC GLP-1 receptor–expressing neurons that are highly colocalized with POMC but not NPY neurons and a second subset that does not coexpress with either NPY or POMC. Although our data raise the possibility that POMC neurons within the ARC play a role in mediating the effects of GLP-1 on glucose homeostasis, the role of the melanocortin system in mediating CNS regulation of glucose homeostasis is not clear. Although 1 week of i3vt administration of α-MSH (α-melanocyte–stimulating hormone) has been found to decrease glucose production (45), acute administration of α-MSH increases glucose production. Furthermore, coadministration of a melanocortin antagonist failed to block centrally administered insulin and leptin-induced decreases in glucose production (40). Such observations point to a potential role for the GLP-1 receptors that are not localized to either POMC or NPY neurons in the ARC.
Although glucose excitatory neurons have been identified in the lateral ARC, controversy arises over their glucose-sensing properties. For example, in electrophysiological studies, POMC neuronal firing rate decreased with decreasing levels of glucose (46). However, brain hyperglycemia failed to induce Fos or to increase the firing rate of POMC neurons (47). Our finding that POMC neurons are not homogenous and that they are composed of at least two distinct cell types either coexpressing or lacking GLP-1 receptors within the ARC might help explain these discrepancies. Another possibility is that glucose may not be the primary stimulus for POMC neurons. Instead, GLP-1 either directly, as recently demonstrated in vitro (48), or indirectly, via release in response to gastric distension (49), increased blood glucose in the portal system—or, by neurotransmitter activation in the gastrointestinal tract (50), might provide stimulation of POMC neurons via GLP-1 receptors in the ARC.
Despite an overall effect to promote glucose disposition, ARC GLP-1 inhibited glucose uptake in peripheral tissues. This seemingly discordant finding is actually consistent with previous data that lateral ventricular infusion of a long-acting GLP-1 analog inhibited skeletal muscle glycogen formation (41). That study suggested that the mechanism was insulin independent and was instead due to direct neural innervation. An alternative hypothesis is there is an indirect effect of CNS GLP-1 on glucose uptake. i3vt administration of GLP-1 increased the proportion of energy derived from fat over carbohydrate in lean and obese Zucker rats (51). Increased fat oxidation could indirectly reduce glucose uptake in muscle (52). Whatever the mechanism, it is important to note that our data indicate that with a physiological glucose load, the overall effect of CNS GLP-1 is to improve glucose homeostasis; i.e., the pancreatic and hepatic effects override any effect on skeletal muscle, leading to overall improved glucose tolerance.
The focus of most studies of the CNS GLP-1 system has been effects on food intake, and the ability of CNS GLP-1 to cause anorexia is mediated at several cerebral sites, e.g., lateral (16), 3rd and 4th ventricular (16,53), and PVN (Fig. 8) (33); in addition, administration of GLP-1 inhibits food intake. However, only when administered into the 4th ventricle (16) or PVN (33) does GLP-1 administration reduce food intake without also causing visceral illness. This is because GLP-1 receptors in the central nucleus of the amygdala initiate visceral illness (16), and lateral and i3vt CNS administration of GLP-1 increases GLP-1 signaling in this nucleus and in brain areas that cause anorexia, indicating that GLP-1 receptors in specific nuclei have discrete effects. Our data extend these findings by revealing that GLP-1 receptors within the ARC regulate blood glucose without affecting food intake while GLP-1 receptors within the PVN regulate food intake without affecting blood glucose.
Our data indicate a common effect of both peripheral and central GLP-1 receptor signaling to promote glucose tolerance, raising the possibility for cross-talk between circulating GLP-1 and the brain. While our data do not test this possibility directly, they do indicate that endogenous CNS GLP-1 receptors are also important mediators of glucose tolerance. Further, since circulating GLP-1 is not increased during glucose tolerance tests, peripheral GLP-1 may not be the important source of GLP-1 activating the CNS receptors that regulate islet and hepatic function. Long-acting GLP-1 analogs improve glucose homeostasis in type 2 diabetic patients. The extent to which these effects are central versus peripheral is unknown, but the data presented here open up the possibility that some of the improvement in glucose levels may be the result of activating ARC GLP-1 receptors.
In conclusion, our data illustrate a novel role for CNS GLP-1 signaling, specifically on ARC neurons, to regulate glucose homeostasis but not food intake. We hypothesize that, after a meal, the coordinated effects of the CNS GLP-1 system are to stimulate the pancreas to secrete insulin and inhibit glucose production but also to inhibit glucose uptake in a KATP channel–dependent manner. Thus, the CNS makes a number of key contributions to the overall effects of GLP-1 to limit postprandial glucose fluctuations.
Acknowledgments
This work was supported by grants from the National Institutes of Health (DK75365 to D.S., DK57900 to D.D., and DK54890 to R.J.S.) and research funding from Amylin Pharmaceuticals.
We thank Mouhamadoul Toure, Joyce Sorrell, Kay Ellis, Eileen Elfers, Helen Mondala, and Justin Kerr for their technical assistance; Dr. Chen Liaw for generating constructs for the rat POMC, NPY, and GLP-1 receptor; and Dr. Jim Leonard and Dan Connolly for their comments and suggestions during the preparation of this manuscript.
Notes
Published ahead of print at http://diabetes.diabetesjournals.org on 16 May 2008.
The costs of publication of this article were defrayed in part by the payment of page charges. This article must therefore be hereby marked “advertisement” in accordance with 18 U.S.C. Section 1734 solely to indicate this fact.
REFERENCES
Articles from Diabetes are provided here courtesy of American Diabetes Association
Full text links
Read article at publisher's site: https://doi.org/10.2337/db07-1824
Read article for free, from open access legal sources, via Unpaywall:
https://diabetesjournals.org/diabetes/article-pdf/57/8/2046/391620/zdb00808002046.pdf
Free to read at intl-diabetes.diabetesjournals.org
http://intl-diabetes.diabetesjournals.org/cgi/content/abstract/57/8/2046
Free after 6 months at intl-diabetes.diabetesjournals.org
http://intl-diabetes.diabetesjournals.org/cgi/content/full/57/8/2046
Free after 6 months at intl-diabetes.diabetesjournals.org
http://intl-diabetes.diabetesjournals.org/cgi/reprint/57/8/2046.pdf
Citations & impact
Impact metrics
Article citations
GLP-1R-positive neurons in the lateral septum mediate the anorectic and weight-lowering effects of liraglutide in mice.
J Clin Invest, 134(17):e178239, 03 Sep 2024
Cited by: 0 articles | PMID: 39225090 | PMCID: PMC11364389
Regulation of Energy and Glucose Homeostasis by the Nucleus of the Solitary Tract and the Area Postrema.
Endocrinol Metab (Seoul), 39(4):559-568, 01 Aug 2024
Cited by: 0 articles | PMID: 39086274 | PMCID: PMC11377841
Review Free full text in Europe PMC
Stem Cell Theory of Cancer: Clinical Implications for Cellular Metabolism and Anti-Cancer Metabolomics.
Cancers (Basel), 16(3):624, 31 Jan 2024
Cited by: 0 articles | PMID: 38339375 | PMCID: PMC10854810
Satiety: a gut-brain-relationship.
J Physiol Sci, 74(1):11, 17 Feb 2024
Cited by: 5 articles | PMID: 38368346 | PMCID: PMC10874559
Review Free full text in Europe PMC
GLP-1 Analogs, SGLT-2, and DPP-4 Inhibitors: A Triad of Hope for Alzheimer's Disease Therapy.
Biomedicines, 11(11):3035, 12 Nov 2023
Cited by: 5 articles | PMID: 38002034 | PMCID: PMC10669527
Review Free full text in Europe PMC
Go to all (208) article citations
Similar Articles
To arrive at the top five similar articles we use a word-weighted algorithm to compare words from the Title and Abstract of each citation.
Central Nervous System GLP-1 Receptors Regulate Islet Hormone Secretion and Glucose Homeostasis in Male Rats.
Endocrinology, 158(7):2124-2133, 01 Jul 2017
Cited by: 20 articles | PMID: 28430981 | PMCID: PMC5505222
A physiological role of glucagon-like peptide-1 receptors in the central nervous system of Suncus murinus (house musk shrew).
Eur J Pharmacol, 668(1-2):340-346, 06 Jul 2011
Cited by: 11 articles | PMID: 21756894
Glucagon-like peptide-1 (GLP-1) receptors expressed on nerve terminals in the portal vein mediate the effects of endogenous GLP-1 on glucose tolerance in rats.
Endocrinology, 148(10):4965-4973, 21 Jun 2007
Cited by: 199 articles | PMID: 17584962
Minireview: finding the sweet spot: peripheral versus central glucagon-like peptide 1 action in feeding and glucose homeostasis.
Endocrinology, 150(7):2997-3001, 23 Apr 2009
Cited by: 53 articles | PMID: 19389830 | PMCID: PMC2703557
Review Free full text in Europe PMC
Funding
Funders who supported this work.
NIDDK NIH HHS (8)
Grant ID: K01 DK075365
Grant ID: DK75365
Grant ID: R01 DK054890
Grant ID: K01 DK075365-01
Grant ID: DK54890
Grant ID: DK57900
Grant ID: R01 DK057900
Grant ID: K01 DK075365-02