Abstract
Free full text

Loss of Extracellular Superoxide Dismutase Leads to Acute Lung Damage in the Presence of Ambient Air
Abstract
The extracellular superoxide dismutase 3 (SOD3) is highly expressed in both blood vessels and lungs. In different models of pulmonary injury, SOD3 is reduced; however, it is unclear whether this contributes to lung injury. To study the role of acute SOD3 reduction in lung injury, the SOD3 gene was deleted in adult mice by using the Cre-Lox technology. Acute reduction of SOD3 led to a fivefold increase in lung superoxide, marked inflammatory cell infiltration, a threefold increase in the arterial-alveolar gradient, respiratory acidosis, histological changes similar to those observed in adult respiratory distress syndrome, and 85% mortality. Treatment with the SOD mimetic MnTBAP and intranasal administration of SOD-containing polyketal microparticles reduced mortality, prevented the histological alterations, and reduced lung superoxide levels. To understand how mice with the SOD3 embryonic deletion survived without lung injury, gene array analysis was performed. These data demonstrated the up-regulation of 37 genes and down-regulation of nine genes, including those involved in cell signaling, inflammation, and gene transcription in SOD3−/− mice compared with either mice with acute SOD3 reduction or wild-type controls. These studies show that SOD3 is essential for survival in the presence of ambient oxygen and that acute loss of this enzyme can lead to severe lung damage. Strategies either to prevent SOD3 inactivation or to augment its levels might prove useful in the treatment of acute lung injury.
The superoxide dismutases (SODs) are major defenses against oxidative damage caused by the superoxide anion (O2).1 There are three isoforms of SOD in mammalian cells. In most tissues, the copper/zinc superoxide dismutase (Cu/ZnSOD or SOD1), a cytoplasmic copper-containing enzyme, is predominant. Mice lacking SOD1 are predisposed to hepatic fat deposition, hepatic tumor development, motor neuron disease, abnormal vascular function, and vascular hypertrophy.2,3,4,5,6 Manganese superoxide dismutase (MnSOD or SOD2) is localized to the mitochondria, where it plays a major role in defending against O2
generated as a co-product of electron transport.7 Mice lacking MnSOD die of a cardiomyopathy within the first 10 days of life.8 The last discovered of the SOD isoforms is extracellular superoxide dismutase, or SOD3. SOD3 is similar to SOD1 in that it is a copper/zinc-containing enzyme; however, it contains a signal sequence that targets it to the Golgi secretory apparatus and it is loaded with copper via two novel proteins, Atox-1 and Menkes.9 SOD3 is secreted via the trans-Golgi network and is bound to components of the extracellular matrix including heparin binding sites, fibullin-5,10 collagen-I, and hyaluronan.11,12,13 While SOD3 represents less than 5% of the total SOD in most cells, it is highly expressed in the lung.14,15
The adult respiratory distress syndrome represents a major cause of morbidity and mortality in hospitalized patients and is observed in the setting of diverse clinical insults, including sepsis, hemorrhage, surgery, trauma, and extensive transfusion. The precise mechanisms whereby these various clinical entities predispose to lung injury remain poorly defined. Of note, several experimental models of lung injury, including hypoxia, asbestos exposure, bleomycin, and hyperoxia, are associated with reduced lung SOD3 protein and activity.16,17,18,19 These studies suggest that extracellular oxidative stress contributes to the pathogenesis of acute lung injury and that SOD3 protects against it.19,20,21,22
In these cases of lung injury, it is unclear whether the loss of SOD3 contributes to or is simply a consequence of lung injury. Of note, lung injury caused by exposure to 100% oxygen is hastened in mice with embryonic deletion of SOD3 (SOD3−/− mice). Embryonic SOD3−/− mice tolerate exposure to ambient oxygen levels without difficulty; however, they have had a life-time to compensate for any deleterious effects of SOD3 deletion. These animals are therefore not informative in determining the role of acute SOD3 reduction in causing lung damage in the presence of normal oxygen concentrations.
The present study was performed to accomplish the following goals. First, we sought to determine whether acute reductions in SOD3, similar to that observed in experimental models of lung damage, could cause lung injury without exposure to hyperoxia. Second, we sought to develop a method of delivering exogenous SOD to prevent lung damage. To this end, we developed a novel polyketal particle that could be delivered intranasally and that could release SOD slowly over several days. Third, we examined potential compensatory mechanisms present in mice with embryonic deletion of SOD3 that could potentially prevent lung damage in these animals.
Materials and Methods
Creation and Study of Tgcre/esr × ecSODloxp/loxp Mice
The targeting vector was created by modifying a 6.12-kb HindIII fragment of the mouse SOD3 gene obtained from the BAC clone 229-C-20 on vector pBeloBAC (Genome Systems, St. Louis, MO). This was extended by cloning an additional 3 kb into the 5′ end. A single loxP site was inserted into intron 1 at the AatII site and a loxP-flanked neomycin resistance cassette was inserted at ApaI site 3′ of exon 2. All three loxP sites were in the same orientation. The targeting vector used for homologous recombination was 10.7 kbp in length and the 5′ and 3′homologous sequences for targeting were 4.43 kb and 1.23 kb, respectively.
Electroporation of the linearized DNA fragment into embryonic stem cells yielded 306 clones resistant to G418. Southern blotting using a probe external to the targeting vector indicated that two clones had successful recombination. The neomycin cassette was removed by transfection of embryonic stem cells with Cre-recombinase containing plasmid, yielding cells that harbored a SOD3 targeted allele (see Supplemental Figure 1 available at http://ajp.amjpathol.org). A high percentage of chimeric mice were generated by blast injection and were bred to C57BL/6 mice to allow germ line transmission of the floxed SOD3 allele. Heterozygous ecSODloxp offspring were backcrossed six generations to C57BL/6 wild-type mice before being crossed to the tamoxifen-Cre transgenic mice. These were then intercrossed a second time to generate Tgcre/esr × ecSODloxp/loxp experimental animals. The control group for experiments was C57Bl/6 mice that were purchased from the Jackson Laboratory (Bar Harbor, Maine). Mice were fed with regular chow diet (LabDiet, rodent diet no. 5001) ad libitum and studied between 10 and 12 weeks of age after CO2 euthanization. The Emory University Animal Care and Use Committee approved the protocol for animal use. Experiments were performed when mice were 3 months of age.
Measurement of Blood Gases, Blood Pressure, and Cardiac Contractility
Mice were anesthetized with 1% to 2% isoflurane in 100% O2, the abdomen opened, and ~150 μl of blood was collected from the abdominal aorta into a heparinized syringe and analyzed on an ABL5 blood gas analyzer (Radiometer Copenhagen). Arterial blood samples were obtained as soon as the animals became ill. Total anesthesia time was held constant in mice from each treatment group. Partial pressures of oxygen, CO2, and pH were measured to calculate arterial-alveolar gradient. Blood pressure was measured noninvasively via the tail cuff method and invasively using radiotelemetry as previously described.23 Cardiac function was evaluated using the Siemens Sequoia Acuson 512 Sonograph (Siemens, Mountain View, CA) and 15L8 linear transducer (14 MHz). Two-dimensional images were acquired at a depth setting of 2 cm.
Tamoxifen Injection and Administration of MnTBAP and SOD Microparticles
Cre-recombinase was activated by the intraperitoneal injection of tamoxifen (3 mg/20 g of body weight) for 5 consecutive days. Control mice were studied in parallel. These animals received injections with an equivalent volume of the vehicle, corn oil, for 5 consecutive days. As another control, C57BL/6 mice were treated for a similar period of time with tamoxifen. In some experiments, the SOD mimetic MnTBAP (Calbiochem catalog no. 475870, purity >95%, 1 mg/20 g of body weight) was injected intraperitoneally daily starting 2 days before tamoxifen injection, during tamoxifen treatment, and for 7 days after tamoxifen treatment. In other experiments, 80 μl of polyketal particles containing SOD (5 mg/ml, described below) were administrated intranasally while mice were briefly anesthetized with isoflurane (2%).
Histology and Immunostaining
After CO2 euthanization, a cannula was placed in the left ventricle and the mice were initially perfused at 100 mmHg with saline to remove blood and then with 10% formaldehyde. Lungs and other organs were harvested after 10 minutes of perfusion and preserved in formaldehyde until embedded in paraffin, sectioned, and immunostained for SOD3 using a rabbit polyclonal antibody against SOD3 previously described.24
Flow Cytometry Analysis of Lung Inflammatory Cells
Lungs were cleared of blood by perfusion with phosphate-buffered saline (PBS), excised, and digested using collagenase type IX (125 U/ml), collagenase type IS (450 U/ml), and hyaluronidase IS (60 U/ml) dissolved in 20 mmol/L 4-(2-hydroxyethyl)-1-piperazineethanesulfonic acid-PBS buffer containing calcium. The digested tissue was then passed through a 70-μm sterile cell strainer (Falcon, BD), yielding single cell suspensions. Cell labeling was performed using the following antibodies (all from BD PharMingen, apart from CD49b): fluorescein isothiocyanate anti-CD45 (30-F11); PerCP anti-CD45 (30-F11); PE anti-CD4 (GK1.5); APC anti-CD4 (GK1.5); PerCP anti-CD8 (53-6.7); APC anti-CD3 (145-2C11); fluorescein isothiocyanate anti-I-Ab (AF6-120.1); APC anti-CD11c (HL3); APC CD11b (M1/70); APC CD4 (RM4-5); PerCP CD4 (RM4-5); PE NK1.1 (PK136); PE CD49b (eBioscience). Cells were washed twice with 1% bovine serum albumin-PBS buffer and additionally incubated for 30 minutes in 37°C with complete media (RPMI 1640 with 10% fetal calf serum). Fluorescence-activated cell sorting analysis was performed using a Becton Dickson LSRII flow cytometer. An initial gate was applied to exclude cell debris from further analysis, and CD45 staining was used to identify leukocytes within the aortic cell suspension. Within the CD45+ gate individual subpopulations of leukocytes were identified with antibodies listed above. Data were analyzed using Flowjo software (Treestar) and expressed as an absolute number of cells per two lungs.
Western Blots and SOD Activity
Protein expression was examined using Western blot analysis as previously described. Antibodies used were the following: anti-SOD1 from Biodesign International; anti- SOD2 from Stressgen; and anti-SOD3 as previously described.24 Equal gel loading was determined by blotting for α-actin (Sigma). Activity of SOD isoforms was determined spectrophotometrically by monitoring the inhibition of the rate of xanthine oxidase-mediated reduction of cytochrome c. SOD3 was separated from intracellular SOD with concavalin A as described previously.24
Detection of Extracellular Superoxide Using Electron Spin Resonance
To detect lung O2 production, we used electron spin resonance spectroscopy with the CAT1H spin probe as previously described.25 The SOD-inhibited amplitude of the low-field component of the electron spin resonance spectra of oxidized CAT1H was used to quantify extracellular O2
production. Values were normalized to dry weight.
Formulation of SOD-Loaded Microparticles
SOD-loaded microparticles were formulated from the acid- sensitive polymer poly(cyclohexane-1,4-diyl acetone dimethylene ketal) using a modified water-in-oil-in-water (w/o/w) double-emulsion procedure. As reported previously, SOD-loaded poly(cyclohexane-1,4-diyl acetone dimethylene ketal) microparticles degrade in acidic environments and have been shown to decrease cellular superoxide in vitro.26 Briefly, 15 mg of SOD (Sigma) was dissolved in 75 μl of a 2.5% (w/v) polyvinyl alcohol (Sigma, 31–50 kd) solution prepared from a pH 8.0 sodium phosphate buffer (0.1 mol/L). This aqueous SOD/polyvinyl alcohol solution was dispersed via homogenization (21,000 rpm for 60 seconds.) into 1.0 ml of a 15% (w/v) poly(cyclohexane-1,4-diyl acetone dimethylene ketal)/ CHCl3 solution, generating the w/o primary emulsion. This SOD-containing w/o emulsion was then transferred by pipette to the bottom of a 24-ml vial containing 15 ml of an aqueous 5% (w/v) polyvinyl alcohol solution and homogenized (9500 rpm for 90 seconds.) to produce the w/o/w secondary emulsion. This w/o/w emulsion was then poured into a stirred 5% polyvinyl alcohol solution buffered at pH 8.0 by a 0.1 mol/L sodium phosphate buffer. This mixture was then continuously stirred for 3 hours to allow the CHCl3 to evaporate, leaving the polymer hardened around the SOD to generate SOD microparticles. To remove the excess polyvinyl alcohol, the hardened particles were transferred to 50-ml centrifuged tubes and washed by successively isolating the particles via centrifugation, decanting the supernatant, and then resuspending the particles in distilled water. The particles were washed three times and then freeze-dried, producing approximately 30 mg of SOD microparticles. A scanning electron microscopic image of the SOD microparticles (see Supplemental Figure 2 available at http://ajp. amjpathol.org) shows that these particles have diameters from 1 to 25 μm, with the majority of the particles having diameters in the 5- to 20-μm range.
The amount of SOD loaded into the particles was determined by a commercially available BCA protein assay kit (BioRad) using the following procedure. The encapsulated SOD was recovered from the microparticles by hydrolyzing 5 mg of SOD microparticles in a 1 N HCl solution containing 2% (w/v) sodium dodecyl sulfate to hydrolyze the acid-sensitive poly(cyclohexane-1,4-diyl acetone dimethylene ketal) polymer. This acidic solution was the neutralized with 1 N NaOH and assayed for protein content using the BCA protein assay with various concentrations of SOD in 1% sodium dodecyl sulfate that had been acidified and subsequently neutralized as a standard. The protein loading assays showed that each milligram of SOD microparticles contained 32.1 μg of SOD. Further details regarding these microparticles have been described previously.27
Statistical Analysis
Data are expressed as means ± SEM. Comparisons between groups were performed using analysis of variance and a Bonferroni/Dunn post hoc test for comparison of selected groups.
Results
Conditional Reduction of SOD3
Tgcre/esr × ecSODloxp/loxp mice were phenotypically identical to C57BL/6 mice. Administration of tamoxifen had no effect on C57BL/6 mice or on ecSODloxp/loxp mice not harboring Cre-recombinase. Likewise, administration of vehicle (corn oil) to either C57BL/6 or Tgcre/esr × ecSODloxp/loxp mice had no effect on survival. In contrast, administration of tamoxifen to Tgcre/esr × ecSODloxp/loxp mice led to striking mortality such that 85% of mice died within 7 days (Figure 1). The pattern of death in these animals was characterized by normal behavior without any evidence of illness until a few hours before death, when they became hunched, demonstrated limited motion, had tachypnea, and died shortly thereafter. After recognizing this, we began to sacrifice mice at the time that they became ill and harvest organs for study. The control animals (C57BL/6 receiving vehicle and tamoxifen and Tgcre/esr × ecSODloxp/loxp mice receiving vehicle) were euthanized in parallel at this time. In general, this was between 3 and 7 days following onset of tamoxifen injections. Data in the figures therefore represent these time points.
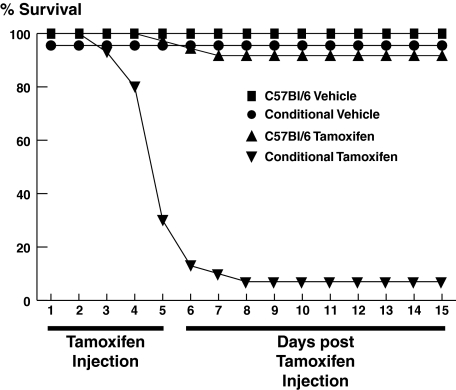
Effect of SOD3 deletion on survival. C57BL/6 and Tgcre/esr × ecSODloxp/loxp mice were injected with tamoxifen (3 mg/20 g of body weight) or vehicle (equivalent volume of corn oil) intraperitoneally for 5 consecutive days. In additional experiments conditional SOD3 deletion, mice lacking the cre/esr transgene (Cre−/−) were injected with tamoxifen.
Tamoxifen treatment reduced SOD3 protein without altering SOD1 levels in lungs of Tgcre/esr × ecSODloxp/loxp mice while having no effect in C57BL/6 mice (Figure 2A). The decrease in SOD3 protein was paralleled by a reduction in its enzymatic activity, while there was no change in activity of intracellular SOD isoforms (Figure 2B). Polymerase chain reaction, using primers external to the loxP sites flanking exon 3, confirmed deletion of this exon following 5 days of tamoxifen injection (see Supplemental Figure 3 available at http://ajp.amjpathol.org). Immunostaining also showed a marked decrease in lung SOD3 following tamoxifen administration in Tgcre/esr × ecSODloxp/loxp mice with no change in C57BL/6 mice (Figure 2C). This reduction of lung SOD3 was associated with a marked increase in lung O2 levels as measured using electron spin resonance (Figure 2D). Treatment with MnTBAP, while not inhibiting scission of exon 3, prevented this increase in O2
(Figure 2D and Supplemental Figure 3 available at http://ajp.amjpathol.org).
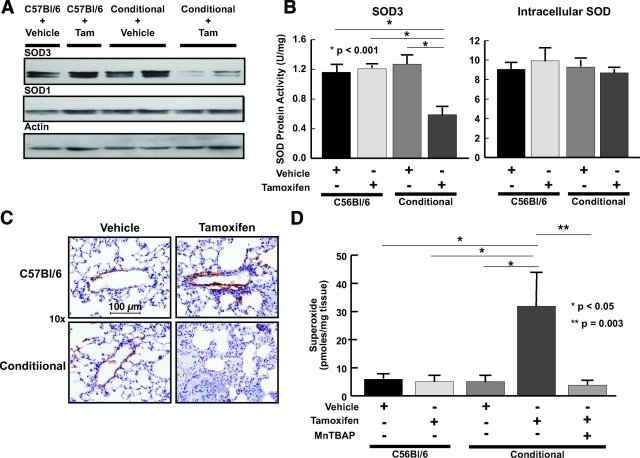
Effect of Cre-recombinase induction on SOD levels and activity and O2 levels in Tgcre/esr × ecSODloxp/loxp mice. A: Western blot showing protein levels of SOD1 and SOD3 in lung tissue of C57BL/6 and Tgcre/esr × ecSODloxp/loxp mice injected with either tamoxifen or vehicle. B: Effect of SOD3 depletion on intracellular SODs and SOD3 enzymatic activity in Tgcre/esr × ecSODloxp/loxp and C57BL/6 mouse lung. Lung tissue was homogenized and SOD isoforms separated as described in the text. SOD activity was determined by measuring the ability to inhibit xanthine/xanthine oxidase cytochrome c reduction. Data are the means of three experiments. C: Immunohistochemistry and hematoxylin and eosin staining for SOD3 in lungs of C57BL/6 and Tgcre/esr × ecSODloxp/loxp mice injected with tamoxifen (magnification, ×40). D: Effect of SOD3 reduction on O2
production in C57BL/6 and Tgcre/esr × ecSODloxp/loxp mice. Three or four 1-mm-thick slices were incubated in buffer containing the CAT1H probe for one hour at 37°C. Parallel slices were incubated with CAT1H and Cu/ZnSOD (100 U/ml). Aliquots of buffer were then examined using electron spin resonance (n = 6 or 7 per group).
SOD3 Reduction Induces Lung Damage
Histological examination of the heart (Figure 3A), aorta (Figure 3B), kidney, and liver (data not shown) revealed no significant pathology in Tgcre/esr × ecSODloxp/loxp mice following tamoxifen injection. Likewise, lung structure was not altered in C57BL/6 mice given either vehicle or tamoxifen or in Tgcre/esr × ecSODloxp/loxp mice given vehicle (Figure 3C). In contrast, administration of tamoxifen to Tgcre/esr × ecSODloxp/loxp mice caused a striking alteration of lung histology, with thickening of the alveolar septa, a marked inflammatory cell infiltrate, hemorrhage into the alveoli, and loss of patent alveoli (Figure 3, C and D).
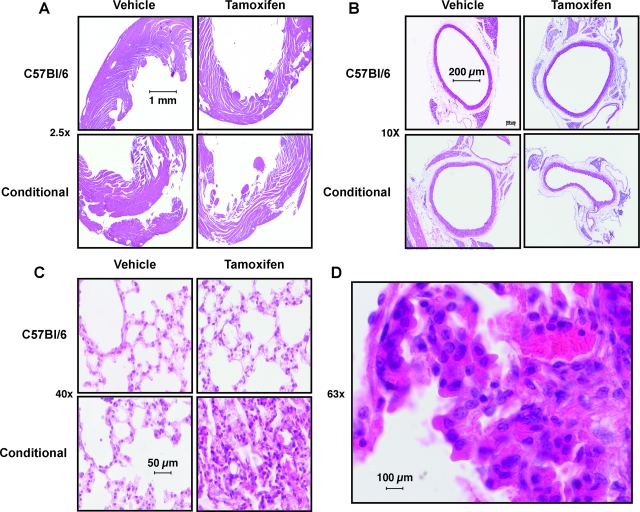
Effect of SOD3 deletion on histology of the heart, aorta, and lungs. Hematoxylin and eosin staining of 5-μm paraffin-embedded slides of myocardium (A, ×10); aorta (B, ×4); and lung (C, ×40) following either tamoxifen or vehicle injection (×10). D shows a higher magnification (×63) of eosin-stained lung with neutrophils, lymphocytes, and other inflammatory cells visible.
Acute reduction of SOD3 was associated with a striking increase in lung levels of granulocytes, T cells, and natural killer cells. Among T cells, CD8 and to a lesser extent CD4+ cells were increased (Figure 4). Reduction of SOD3 did not change lung content of dendritic cells, B cells, or macrophages (data not shown). Injection of tamoxifen in C57BL/6 mice or vehicle in to Tgcre/esr × ecSODloxp/loxp mice had no effect on lung inflammatory cells.
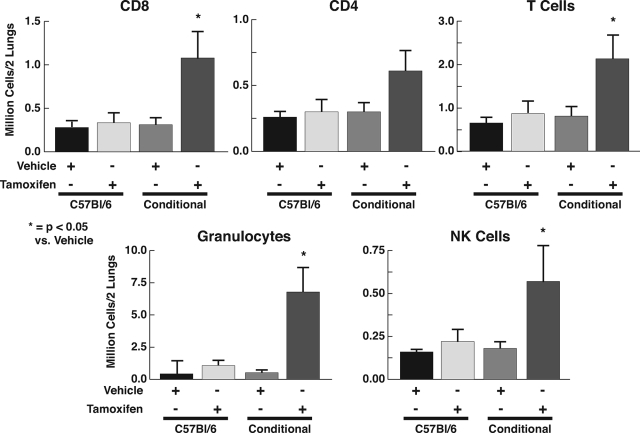
Lung infiltration of inflammatory cells following reduction of SOD3. Lungs were cleared of blood by perfusion with PBS, excised, and digested using collagenase and hyaluronidase as described in Materials and Methods. The homogenates were dissolved in 20 mmol/L 4-(2-hydroxyethyl)-1-piperazineethanesulfonic acid-PBS and passed through a 70-μm sterile cell strainer, yielding single cell suspensions. Cells were immunostained, resuspended in fluorescence-activated cell sorting buffer, and immediately analyzed via flow cytometry.
In additional studies, we measured arterial blood gases while the mice were anesthetized with isoflurane and breathing 100% O2. Deletion of the SOD3 gene resulted in a reduction in pO2 and pH, an increase in pCO2, and a marked widening of the arterial-alveolar oxygen gradient (Table 1). Neither tamoxifen nor vehicle altered these parameters in C57BL/6 mice, and vehicle had no effect on blood gases in Tgcre/esr × ecSODloxp/loxp mice.
Table 1
Arterial Blood Gases in C57BL/6 and Tgcre/esr × ecSODloxp/loxp Mice Injected with Tamoxifen or Vehicle
pH | pO2 (mmHg) | pCO2 (mmHg) | Arterial-alveolar gradient (mmHg) | |
---|---|---|---|---|
C57Bl6 vehicle | 7.37 ± 0.01 | 545 ± 40 | 38 ± 1 | 78 ± 20 |
C57Bl6 tamoxifen | 7.36 ± 0.02 | 596 ± 26 | 42 ± 4 | 55 ± 27 |
Conditional vehicle | 7.37 ± 0.01 | 581 ± 19 | 42 ± 1 | 79 ± 19 |
Conditional tamoxifen | 7.23 ± 0.05*† | 409 ± 67† | 53 ± 3† | 237 ± 67*† |
Effect of Systemic and Locally Administered SOD on Survival and Lung Histology
In other experiments we determined if either systemic or local administration of antioxidants could prevent lung injury and mortality caused by deletion of ecSOD. Co-treatment of Tgcre/esr × ecSODloxp/loxp mice with tamoxifen and intraperitoneal MnTBAP markedly reduced mortality and lung injury caused by SOD3 reduction (Figure 5,A and B). To directly demonstrate that pulmonary augmentation of SOD activity could prevent mortality and lung damage in these animals, we administered polyketal microparticles containing SOD1 via an intranasal route. This treatment also reduced mortality and alterations of lung morphology caused by acute SOD3 depletion (Figure 5B). Taken together, these data illustrate that a relatively moderate (50%) reduction of SOD3 in adult animals causes severe lung damage and death and that this can be prevented by either systemic augmentation of superoxide scavenging or by directed pulmonary delivery of SOD.
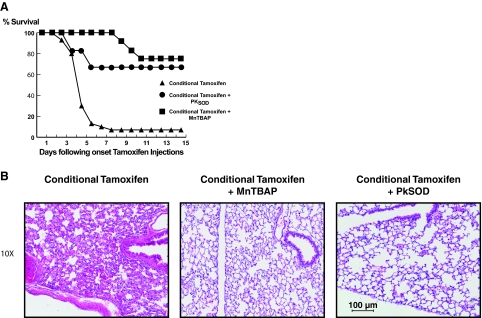
A: Effect of the SOD mimetic MnTBAP and SOD-containing microparticles on survival (A) and lung injury (B) in C57BL/6 and Tgcre/esr × ecSODloxp/loxp mice. MnTBAP (1 mg/20 g of body weight) was dissolved in PBS and injected intraperitoneally for 3 consecutive days before starting the tamoxifen or vehicle injections and for 12 days thereafter. During isoflurane anesthesia, SOD-containing nanoparticles were administered intranasally (1 mg/20 g of body weight) 3 days before starting tamoxifen and vehicle injections, on the third day of treatment, and 3 days after finishing treatment. B: Lungs were fixed with formalin 10% and embedded in paraffin. Five-μm slices were cut and stained with hematoxylin and eosin. Magnification, ×10.
In additional experiments, we examined the effect of acute deletion of SOD3 on hemodynamics. Previous studies have shown that mice with embryonic knockout of SOD3 have normal blood pressure at baseline, but develop augmented hypertension during angiotensin II infusion.25 Moreover, their aortas have higher levels of O2 and abnormal endothelium-dependent vasodilatation at baseline. We sought to determine whether acute deletion of SOD3 would cause an acute increase in blood pressure. Before the day on which the Tgcre/esr × ecSODloxp/loxp mice became ill, blood pressure was not affected by tamoxifen injection as measured either noninvasively by the tail cuff method or invasively by radiotelemetry (Figure 6A). Likewise, cardiac contractility measured by echocardiography was preserved in Tgcre/esr × ecSODloxp/loxp mice even when the animals became ill (Figure 6B).
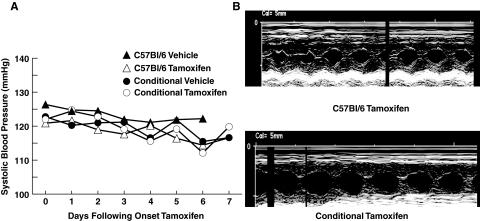
Effect of SOD3 deletion on blood pressure and cardiac function. Blood pressure (mmHg) measured by radiotelemetry in C57BL/6 and Tgcre/esr × ecSODloxp/loxp mice before, during, and after treatment with either tamoxifen or vehicle is shown in A (n = 6–10 per group). B: Examples of echocardiography images obtained in C57BL/6 and Tgcre/esr × ecSODloxp/loxp mice after tamoxifen treatment for 5 days.
To provide an understanding of compensatory mechanisms that might allow mice embryonically deleted SOD3 to survive, we compared mRNAs expressed in the lungs of mice with embryonic deletion of SOD3 to those in either normal wild-type mouse lungs or our Tgcre/esr × ecSODloxp/loxp mice following tamoxifen injection using gene array studies. This analysis revealed 37 genes that were up-regulated in mice with embryonic deletion of SOD3 by more than twofold compared to either of the other groups (Table 2).
Table 2
Gene Array in Lungs from Wild-Type Tgcre/esr × ecSODloxp/loxp/loxp Mice Treated with Tamoxifen and SOD3−/−
Gene name | Fold change SOD3−/− versus conditional knockout | Fold change SOD3−/− versus wild type |
---|---|---|
Igh-VJ558 | 11.06 | 30.39 |
Fosb | 6.84 | 4.97 |
Camk2β | 6.53 | 5.26 |
Nr4a1 | 4.21 | 3.46 |
Egr3 | 4.14 | 5.46 |
Fgfr3 | 3.70 | 2.43 |
Ctse | 3.58 | 9.43 |
Cd59a | 3.49 | 2.65 |
Utrn | 3.23 | 3.02 |
Egr1 | 3.11 | 3.69 |
Zfp236 | 3.00 | 2.10 |
Ndst1 | 2.87 | 4.38 |
Atf3 | 2.70 | 2.92 |
Cyr61 | 2.65 | 2.81 |
Apold1 | 2.55 | 2.75 |
Wdfy1 | 2.52 | 3.35 |
Nr1d2 | 2.43 | 4.78 |
Arf3 | 2.42 | 3.26 |
Cpd | 2.38 | 2.54 |
Rassf3 | 2.03 | 2.98 |
Etv5 | 2.00 | 2.39 |
Ptprj | −2.45 | −2.40 |
Peg3 | −2.54 | −2.06 |
Lrp1 | −2.74 | −2.02 |
Cd163 | −3.42 | −2.25 |
Il22ra2 | −4.13 | −2.34 |
Mid1 | −11.5 | −8.84 |
Discussion
There are several important new findings derived from the current study. First, in the presence of normal oxygen tension, an acute reduction of SOD3 causes severe lung disease. This adds to our current understanding of the role of SOD3, because prior studies of mice with embryonic deletion of SOD3 showed that lung damage only occurred on exposure to 100% oxygen or challenged with other insults. Our findings that acute loss of this protein in adult animals might have implications for other etiologies of lung disease, where SOD3 is also reduced. Second, we developed a novel method of locally delivering exogenous SOD using polyketal particles that was effective in preventing lung disease. Given the similarity between the lung damage observed in our Tgcre/esr × ecSODloxp/loxp mice and other models of acute lung injury, such a therapeutic approach might prove effective in treating additional causes of pulmonary injury. Third, our gene array analysis show a striking change in mRNA expression in the lungs of mice with embryonic SOD deletion, providing some insight into how mammals can compensate for a life-long loss of this gene.
The earth formed approximately 4.5 billion years ago, and it has been estimated that it was anoxic until about 2.3 billion years ago.28 At that time, the first photosynthetic organisms, cyanobacteria, became capable of photosynthesis, leading to a sudden increase in oxygen in the oceans and atmosphere. This triggered the development of complex multicellular organisms that depended on oxygen;29 however, a very complex biology arose as a result of this involving utilization of oxygen and protection against oxygen metabolites. Prokaryotic and eukaryotic cells adapted small molecules as antioxidants and developed enzymes that produce, scavenge, and use reactive oxygen species and other enzymes that can prevent and repair oxidative damage. Interestingly, some of these enzymes are highly preserved from bacteria to humans, supporting the concept that the coexistence with oxygen metabolites has been essential for millions of years. One such adaptation that developed in mammals was expression of SOD3 in high amounts in specific organs such as blood vessels30 and the lung.9 The present study shows that SOD3 is essential for survival in the presence of ambient oxygen and that acute deletion of this gene leads to severe acute lung disease. This is associated with a widening of the arterial-alveolar oxygen gradient, a reduction in blood oxygen partial pressure, an increase in plasma CO2, and ultimately death. Systemic treatment with the SOD mimetic MnTBAP or local administration of microparticles containing SOD reduced mortality in these animals and preserved lung architecture. We speculate that these novel SOD carrier microparticles could reduce lung damage and mortality in human lung injury. It has been proposed that SOD mimetics might have a protective role against smoking-induced lung injury and COPD in humans.31 Specific SOD3 polymorphisms have also been associated with varying courses of idiopathic pulmonary fibrosis in humans, further supporting the role of this protein in protecting against lung disease.32 We propose that loss of SOD3 might underlie the progression of lung disease observed following diverse stimuli that would impose an initial oxidant stress, such as infection, radiation, hemorrhage, or trauma.
The highest oxygen tension encountered in vivo is at the alveolar surface, and these high levels of oxygen could serve as a substrate for reactive oxygen species-generating enzymes such as NADPH oxidases within macrophages or in alveolar epithelial cells,33 mitochondria in epithelial cells,34 or xanthine oxidase in epithelial cells.35 The presence of O2-scavenging enzymes would therefore seem to be critical at this site. Indeed, previous studies have shown that the alveolar surface is the major site of lung SOD3.11,36 In keeping with this, we observed a striking increase in superoxide production as measured by electron spin resonance in mice following deletion of SOD3.
Mice with embryonic deletion of SOD3 are predisposed to lung damage when given 100% oxygen for prolonged periods20 or when exposed to other oxidant injuries such as hypoxia or ozone.18,37 The histological appearance of the lungs in these animals following exposure to high oxygen has been reported to be similar to that observed in the current study following acute deletion of the gene in normoxic conditions.20 Surprisingly, mice with embryonic SOD3 deletion tolerate exposure to ambient oxygen, although in preliminary studies, we have found that they have minor degrees of alveolar septal thickening and inflammatory infiltrates that denote a moderate degree of lung injury (data not shown). It is likely that embryonic deletion of SOD3 leads to compensatory mechanisms that allow survival despite structural changes we observed on acute deletion of this gene.
Previous studies have supported a role for reactive oxygen species such as superoxide in inducing chemotaxis of inflammatory cells38 and that SOD3 prevents inflammation.39,40 Our fluorescence cell sorting analysis showed that acute deletion of SOD3 leads to a striking pulmonary infiltration of various inflammatory cells. Among T cell subtypes, the increase in CD8+ cells was greater than CD4+ cells. In keeping with these findings, Hashimoto et al have shown that bleomycin-induced lung injury is associated with a lymphocytic infiltrate in mice.41 These investigators have also shown high levels of mRNA for several products of CD8+ T cells, including granzymes A and B, perforin, Fas, and Fas ligand in the bronchoalveolar fluid of patients during the acute phase of lung injury.42 These molecules participate in apoptotic cytotoxicity induced by CD8 cells and could contribute to lung injury. The large accumulation of granulocytes occurs in numerous types in acute lung injury.43,44
As seen in Figure 4, tamoxifen injection caused a small increase in granulocytes in wild-type mice. We considered the possibility that tamoxifen could induce an inflammatory response in the absence of SOD3 and that the acute reduction in SOD3 caused by tamoxifen was not the cause of lung damage and death. To address this, we treated SOD3−/− mice for 5 days with tamoxifen (n = 6). This caused no death or change in lung histology (data not shown). We therefore do not believe that tamoxifen nonspecifically caused lung damage in the absence of SOD3 but that its effect was due to acute reduction of SOD3.
In Tgcre/esr × ecSODloxp/loxp mice, induction of Cre-recombinase using tamoxifen did not completely eliminate SOD3 but reduced it by 50 to 60%, presumably due to an incomplete deletion of the gene in the target tissues. It is possible that SOD3 levels would have decreased further; however, mice became ill generally between 3 and 7 days following the initial tamoxifen injection, and we harvested organs at that time, such that it was impossible to determine the efficacy of Cre-recombinase induction on SOD3 levels in these animals at latter times. In Tgcre/esr × ecSODloxp/loxp mice that survived tamoxifen injections, we found minimal (<30%) reduction of SOD3 in preliminary studies, suggesting that these animals might have survived simply because of persistent SOD3 expression. The reason why tamoxifen had minimal effect in these animals is unclear but might be due to technical problems due to intraperitoneal injection or variability of Cre-recombinase induction. Our data can also be interpreted as showing that as lung SOD3 levels fall, when they reach approximately 50% of normal levels, acute lung injury occurs. Despite this modest reduction of SOD3, there was a striking increase in lung O2 production as estimated by electron spin resonance, in keeping with the rapid kinetics of the reaction between SOD and O2
. It is unlikely that the lung damage occurred as a consequence of tamoxifen or the vehicle used, as tamoxifen had no effect on survival or lung histology in C57BL/6 mice or ecSODloxp/loxp mice lacking Cre-recombinase. The fact that MnTBAP and SOD1 locally released from microparticles could prevent mortality in these mice strongly supports a role for O2
in the genesis of the lung damage. The role of MnTBAP as antioxidant has been already demonstrated in prior studies, in which MnTBAP has prolonged survival in SOD2-deficient mice and reduced injury in Paraquat-induced lung injury.45,46
Several prior studies have shown that diverse injuries such as hyperoxia, asbestos injury, and hypoxia are associated with a decrease in lung activity and protein levels of SOD3 ranging from 30% to 50%.16,17,18 In these studies, it was not clear whether this decrease in SOD3 was the cause of lung injury or was simply a reflection of destruction of cells that produce this enzyme. Our present experiments indicate that a 50% decrease in SOD3 can cause lung injury even in the absence of an initiating insult. In addition to changes in protein levels, hydrogen peroxide can lead to inactivation of the Cu/ZnSODs via a reaction with the copper catalytic center of these enzymes.47,48,49 It is therefore possible that hydrogen peroxide generated in response to stimuli such as infection or trauma could lead to inactivation of SOD3 without changing its protein levels.
To gain insight into mechanisms that allow survival of mice with embryonic deletion of SOD3 that are not present in the Tgcre/esr × ecSODloxp/loxp mice, we performed gene array studies analyzing 47,000 mRNAs in these animals. Thirty-seven genes were up-regulated by more than twofold in the SOD3−/− lungs compared to either wild-type mice or the Tgcre/esr × ecSODloxp/loxp mice after tamoxifen treatment. These encoded several factors involved in cell signaling, including the transcription factors FosB, Nr4a1, Erg1, and Etv5. FosB interacts with the DNA-binding complex AP1 and modulates nuclear gene transcription.50 The transcription factor early growth response (Erg-1) has been shown to be involved in the tissue factor gene regulation,51 and Etv5 is a member of the Ets transcription factor family.44 Other cell signaling genes that are highly expressed in the SOD3−/− lungs include the FGF receptor 3, which has been implicated in lymphatic vessel development52; the Ras effector family member Rassf3, which can function as a tumor suppressor53; and ARf3, a member of the small G-protein family that is involved in epithelial cell proliferation.54 The extracellular matrix encoding mRNAs, utrophin, and Ndst were also increased in the SOD3−/− mice compared to the other animals. Of particular interest, CD59, a surface protein that conveys resistance to compliment attack, was up-regulated about threefold in mice with embryonic deletion of ecSOD. Another striking finding was an increase in immunoglobulin heavy chain VJ558 by 30-fold in the SOD3−/− mice and by threefold in the Tgcre/esr × ecSODloxp/loxp mice compared to wild-type mice. This might implicate inappropriate expression of heavy chain in nonimmunological tissues in response to oxidant stress.
In contrast to the rather large number of genes up-regulated in the ecSOD−/− mouse lungs, a relatively small number were down-regulated. One notable gene that was reduced by two- to 2.5-fold in mice with both embryonic and acute deletion of SOD3 is Peg 3, which is involved in tumor necrosis factor-α signaling and promotes p53-mediated apoptosis.55 In addition, the interleukin-22 receptor was reduced by fourfold in the mice with embryonic deletion. Interleukin-22 is a product of CD4+ T cells and is involved in autoimmune and proinflammatory diseases. Down-regulation of its receptor might convey protection. Another striking finding was a ninefold down-regulation of MID1, a microtubule-associated protein, in SOD3−/− mice. This protein is involved in formation of midline structures; however, its role in inflammation has not been defined.56
The roles of these various genes in protection against oxygen toxicity remain undefined, and a precise definition of their roles is beyond the scope of this study. It is of interest, however, that virtually none of these are obviously involved in scavenging of reactive oxygen species or responses to oxidant stress, while many seem to be involved in protection against inflammatory reactions. It might have been expected that alternate reactive oxygen species-scavenging enzymes would be up-regulated in SOD3−/− mouse lungs, such as one of the other superoxide dismutases or other molecules that could react with superoxide. This analysis emphasizes the unique aspects of the superoxide dismutases in that they seem to be the only enzymes that catalytically remove the superoxide radical and the unique extracellular location of SOD3, which prohibits compensation by intracellular superoxide dismutases. Thus the organism is unable to provide an alternate mode for removing extracellular superoxide dismutase and therefore compensates for the life-long loss of SOD3 by reducing inflammation. These studies therefore emphasize the link between oxidation and inflammation and provide insight into how mammals respond to a loss of a particular reactive oxygen species-scavenging enzyme.
In a prior study, we showed that mice with embryonic deletion of SOD3 develop augmented hypertension in response to angiotensin II infusion but had normal hemodynamics at baseline.25 Given the large amount of SOD3 in vessels, we initially thought that the normal hemodynamics at baseline was due to compensatory mechanisms that could have developed in the SOD3−/− mice during development and that acute reduction of SOD3 might lead to hypertension. The limited data we obtained in mice before death failed to reveal any increase in blood pressure. It is difficult to exclude a role of SOD3 in modulation of basal blood pressure based on these studies because of the limited time before pulmonary pathology developed. A cross of our SOD3loxp/loxp mice with a vascular smooth muscle specific Cre-recombinase could be helpful in this regard. In preliminary studies, we found that blood pressure does not increase in mice given SOD-containing microparticles intranasally, suggesting that this modest decrease in SOD3 in organs other than the lung does not alter baseline blood pressure.
In summary, our current studies show that SOD3 is essential for survival in the presence of ambient oxygen, and even a modest reduction of this enzyme leads to profound lung injury and mortality. These findings have relevance to several causes of lung injury that are associated with decreased SOD3 protein levels and activity, and it is interesting to speculate that in these conditions, the loss of SOD3 is a perpetuating factor. Moreover, in the present study, we show that systemic treatment with MnTBAP or local administration of SOD using novel microparticles can reduce lung damage and reduce mortality. Therapies such as these might prove useful in treating acute lung injury in humans.
Footnotes
Address reprint requests to David G. Harrison, Division of Cardiology, Emory University School of Medicine, 1639 Pierce Drive, Room 319 WMB, Atlanta, GA 30322. E-mail: ude.yrome@20rrahd.
Supported by National Institutes of Health grants HL390006, HL59248, and R21 EB006418; National Institutes of Health Program Project grant HL58000; Nanotechnology Center grant UO1 HL80711-01; a Department of Veterans Affairs merit grant; Georgia Tech/Emory Center for the Engineering of Living Tissues funded by the National Science Foundation grant EEC-9731643; and National Science Foundation Career Award BES-0546962.
M.C.G. and H.E.L. contributed equally to this work.
Supplemental material for this article can be found on http://ajp. amjpathol.org.
Current address of U.L.: Institute of Physiology, Cardiovascular Research Group, University Hospital Zurich, Zurich, Switzerland.
References
- Beyer W, Imlay J, Fridovich I. Superoxide dismutases. Prog Nucleic Acid Res Mol Biol. 1991;40:221–253. [Abstract] [Google Scholar]
- Didion SP, Ryan MJ, Didion LA, Fegan PE, Sigmund CD, Faraci FM. Increased superoxide and vascular dysfunction in CuZnSOD-deficient mice. Circ Res. 2002;91:938–944. [Abstract] [Google Scholar]
- Uchiyama S, Shimizu T, Shirasawa T. CuZn-SOD deficiency causes ApoB degradation and induces hepatic lipid accumulation by impaired lipoprotein secretion in mice. J Biol Chem. 2006;281:31713–31719. [Abstract] [Google Scholar]
- Kessova IG, Ho YS, Thung S, Cederbaum AI. Alcohol-induced liver injury in mice lacking Cu Zn-superoxide dismutase. Hepatology. 2003;38:1136–1145. [Abstract] [Google Scholar]
- Elchuri S, Oberley TD, Qi W, Eisenstein RS, Jackson Roberts L, Van Remmen H, Epstein CJ, Huang TT. CuZnSOD deficiency leads to persistent and widespread oxidative damage and hepatocarcinogenesis later in life. Oncogene. 2005;24:367–380. [Abstract] [Google Scholar]
- Baumbach GL, Didion SP, Faraci FM. Hypertrophy of cerebral arterioles in mice deficient in expression of the gene for CuZn superoxide dismutase. Stroke. 2006;37:1850–1855. [Abstract] [Google Scholar]
- Macmillan-Crow LA, Cruthirds DL. Invited review: manganese superoxide dismutase in disease. Free Radic Res. 2001;34:325–336. [Abstract] [Google Scholar]
- Li Y, Huang TT, Carlson EJ, Melov S, Ursell PC, Olson JL, Noble LJ, Yoshimura MP, Berger C, Chan PH, Wallace DC, Epstein CJ. Dilated cardiomyopathy and neonatal lethality in mutant mice lacking manganese superoxide dismutase. Nat Genet. 1995;11:376–381. [Abstract] [Google Scholar]
- Fukai T, Folz RJ, Landmesser U, Harrison DG. Extracellular superoxide dismutase and cardiovascular disease. Cardiovasc Res. 2002;55:239–249. [Abstract] [Google Scholar]
- Nguyen AD, Itoh S, Jeney V, Yanagisawa H, Fujimoto M, Ushio-Fukai M, Fukai T. Fibulin-5 is a novel binding protein for extracellular superoxide dismutase. Circ Res. 2004;95:1067–1074. [Abstract] [Google Scholar]
- Oury TD, Chang LY, Marklund SL, Day BJ, Crapo JD. Immunocytochemical localization of extracellular superoxide dismutase in human lung. Lab Invest. 1994;70:889–898. [Abstract] [Google Scholar]
- Petersen SV, Oury TD, Ostergaard L, Valnickova Z, Wegrzyn J, Thogersen IB, Jacobsen C, Bowler RP, Fattman CL, Crapo JD, Enghild JJ. Extracellular superoxide dismutase (EC-SOD) binds to type i collagen and protects against oxidative fragmentation. J Biol Chem. 2004;279:13705–13710. [Abstract] [Google Scholar]
- Gao F, Koenitzer JR, Tobolewski JM, Jiang D, Liang J, Noble PW, Oury TD. Extracellular superoxide dismutase inhibits inflammation by preventing oxidative fragmentation of hyaluronan. J Biol Chem. 2008;283:6058–6066. [Europe PMC free article] [Abstract] [Google Scholar]
- Su WY, Folz R, Chen JS, Crapo JD, Chang LY. Extracellular superoxide dismutase mRNA expressions in the human lung by in situ hybridization. Am J Respir Cell Mol Biol. 1997;16:162–170. [Abstract] [Google Scholar]
- Marklund SL. Human copper-containing superoxide dismutase of high molecular weight. Proc Natl Acad Sci U S A. 1982;79:7634–7638. [Europe PMC free article] [Abstract] [Google Scholar]
- Oury TD, Schaefer LM, Fattman CL, Choi A, Weck KE, Watkins SC. Depletion of pulmonary EC-SOD after exposure to hyperoxia. Am J Physiol Lung Cell Mol Physiol. 2002;283:L777–L784. [Abstract] [Google Scholar]
- Tan RJ, Fattman CL, Watkins SC, Oury TD. Redistribution of pulmonary EC-SOD after exposure to asbestos. J Appl Physiol. 2004;97:2006–2013. [Abstract] [Google Scholar]
- Giles BL, Suliman H, Mamo LB, Piantadosi CA, Oury TD, Nozik-Grayck E. Prenatal hypoxia decreases lung extracellular superoxide dismutase expression and activity. Am J Physiol Lung Cell Mol Physiol. 2002;283:L549–554. [Abstract] [Google Scholar]
- Fattman CL, Chang LY, Termin TA, Petersen L, Enghild JJ, Oury TD. Enhanced bleomycin-induced pulmonary damage in mice lacking extracellular superoxide dismutase. Free Radic Biol Med. 2003;35:763–771. [Abstract] [Google Scholar]
- Carlsson LM, Jonsson J, Edlund T, Marklund SL. Mice lacking extracellular superoxide dismutase are more sensitive to hyperoxia. Proc Natl Acad Sci USA. 1995;92:6264–6268. [Europe PMC free article] [Abstract] [Google Scholar]
- Fattman CL, Tan RJ, Tobolewski JM, Oury TD. Increased sensitivity to asbestos-induced lung injury in mice lacking extracellular superoxide dismutase. Free Radic Biol Med. 2006;40:601–607. [Europe PMC free article] [Abstract] [Google Scholar]
- Bowler RP, Nicks M, Warnick K, Crapo JD. Role of extracellular superoxide dismutase in bleomycin-induced pulmonary fibrosis. Am J Physiol Lung Cell Mol Physiol. 2002;282:L719–726. [Abstract] [Google Scholar]
- Laude K, Cai H, Fink B, Hoch N, Weber DS, McCann L, Kojda G, Fukai T, Schmidt HH, Dikalov S, Ramasamy S, Gamez G, Griendling KK, Harrison DG. Hemodynamic and biochemical adaptations to vascular smooth muscle overexpression of p22phox in mice. Am J Physiol Heart Circ Physiol. 2005;288:H7–H12. [Abstract] [Google Scholar]
- Fukai T, Galis ZS, Meng XP, Parthasarathy S, Harrison DG. Vascular expression of extracellular superoxide dismutase in atherosclerosis. J Clin Invest. 1998;101:2101–2111. [Europe PMC free article] [Abstract] [Google Scholar]
- Gongora MC, Qin Z, Laude K, Kim HW, McCann L, Folz JR, Dikalov S, Fukai T, Harrison DG. Role of extracellular superoxide dismutase in hypertension. Hypertension. 2006;48:473–481. [Abstract] [Google Scholar]
- Lee S, Yang SC, Heffernan MJ, Taylor WR, Murthy N. Polyketal microparticles: a new delivery vehicle for superoxide dismutase. Bioconjug Chem. 2007;18:4–7. [Abstract] [Google Scholar]
- Heffernan MJ, Murthy N. Polyketal nanoparticles: a new pH-sensitive biodegradable drug delivery vehicle. Bioconjug Chem. 2005;16:1340–1342. [Abstract] [Google Scholar]
- Raven JA, Johnston AM, Kubler JE, Korb R, McInroy SG, Handley LL, Scrimgeour CM, Walker DI, Beardall J, Clayton MN, Vanderklift M, Fredriksen S, Dunton KH. Seaweeds in cold seas: evolution and carbon acquisition. Ann Bot (Lond) 2002;90:525–536. [Abstract] [Google Scholar]
- Hedges SB, Blair JE, Venturi ML, Shoe JL. A molecular timescale of eukaryote evolution and the rise of complex multicellular life. BMC Evol Biol. 2004;4:2. [Europe PMC free article] [Abstract] [Google Scholar]
- Stralin P, Karlsson K, Johansson BO, Marklund SL. The interstitium of the human arterial wall contains very large amounts of extracellular superoxide dismutase. Arterioscler Thromb Vasc Biol. 1995;15:2032–2036. [Abstract] [Google Scholar]
- Rahman I, Kilty I. Antioxidant therapeutic targets in COPD. Curr Drug Targets. 2006;7:707–720. [Abstract] [Google Scholar]
- Gao F, Kinnula VL, Myllarniemi M, Oury TD. Extracellular superoxide dismutase in pulmonary fibrosis. Antioxid Redox Signal. 2008;10:343–354. [Europe PMC free article] [Abstract] [Google Scholar]
- Papaiahgari S, Kleeberger SR, Cho HY, Kalvakolanu DV, Reddy SP. NADPH oxidase and ERK signaling regulates hyperoxia-induced Nrf2-ARE transcriptional response in pulmonary epithelial cells. J Biol Chem. 2004;279:42302–42312. [Abstract] [Google Scholar]
- Powell CS, Jackson RM. Mitochondrial complex I, aconitase, and succinate dehydrogenase during hypoxia-reoxygenation: modulation of enzyme activities by MnSOD. Am J Physiol Lung Cell Mol Physiol. 2003;285:L189–198. [Abstract] [Google Scholar]
- Kurosaki M, Li Calzi M, Scanziani E, Garattini E, Terao M. Tissue- and cell-specific expression of mouse xanthine oxidoreductase gene in vivo: regulation by bacterial lipopolysaccharide, Biochem J. 1995;306(Pt 1):225–234. [Europe PMC free article] [Abstract] [Google Scholar]
- Fattman CL, Enghild JJ, Crapo JD, Schaefer LM, Valnickova Z, Oury TD. Purification and characterization of extracellular superoxide dismutase in mouse lung. Biochem Biophys Res Commun. 2000;275:542–548. [Abstract] [Google Scholar]
- Jonsson LM, Edlund T, Marklund SL, Sandstrom T. Increased ozone-induced airway neutrophilic inflammation in extracellular-superoxide dismutase null mice. Respir Med. 2002;96:209–214. [Abstract] [Google Scholar]
- Gaboury JP, Anderson DC, Kubes P. Molecular mechanisms involved in superoxide-induced leukocyte-endothelial cell interactions in vivo. Am J Physiol. 1994;266:H637–H642. [Abstract] [Google Scholar]
- Olsen DA, Petersen SV, Oury TD, Valnickova Z, Thogersen IB, Kristensen T, Bowler RP, Crapo JD, Enghild JJ. The intracellular proteolytic processing of extracellular superoxide dismutase (EC-SOD) is a two-step event. J Biol Chem. 2004;279:22152–22157. [Abstract] [Google Scholar]
- Kliment CR, Tobolewski JM, Manni ML, Tan RJ, Enghild J, Oury TD. Extracellular superoxide dismutase protects against matrix degradation of heparan sulfate in the lung. Antioxid Redox Signal. 2008;10:261–268. [Europe PMC free article] [Abstract] [Google Scholar]
- Hagimoto N, Kuwano K, Nomoto Y, Kunitake R, Hara N. Apoptosis and expression of Fas/Fas ligand mRNA in bleomycin-induced pulmonary fibrosis in mice. Am J Respir Cell Mol Biol. 1997;16:91–101. [Abstract] [Google Scholar]
- Hashimoto S, Kobayashi A, Kooguchi K, Kitamura Y, Onodera H, Nakajima H. Up-regulation of two death pathways of perforin/granzyme and FasL/Fas in septic acute respiratory distress syndrome. Am J Respir Crit Care Med. 2000;161:237–243. [Abstract] [Google Scholar]
- Downey GP, Dong Q, Kruger J, Dedhar S, Cherapanov V. Regulation of neutrophil activation in acute lung injury. Chest. 1999;116:46S–54S. [Abstract] [Google Scholar]
- Kobberup S, Nyeng P, Juhl K, Hutton J, Jensen J. ETS-family genes in pancreatic development. Dev Dyn. 2007;236:3100–3110. [Abstract] [Google Scholar]
- Melov S, Schneider JA, Day BJ, Hinerfeld D, Coskun P, Mirra SS, Crapo JD, Wallace DC. A novel neurological phenotype in mice lacking mitochondrial manganese superoxide dismutase. Nat Genet. 1998;18:159–163. [Abstract] [Google Scholar]
- Day BJ, Crapo JD. A metalloporphyrin superoxide dismutase mimetic protects against paraquat-induced lung injury in vivo. Toxicol Appl Pharmacol. 1996;140:94–100. [Abstract] [Google Scholar]
- Liochev SI, Fridovich I. On the role of bicarbonate in peroxidations catalyzed by Cu,Zn superoxide dismutase. Free Radic Biol Med. 1999;27:1444–1447. [Abstract] [Google Scholar]
- Zhang H, Joseph J, Gurney M, Becker D, Kalyanaraman B. Bicarbonate enhances peroxidase activity of Cu, Zn-superoxide dismutase. Role of carbonate anion radical and scavenging of carbonate anion radical by metalloporphyrin antioxidant enzyme mimetics. J Biol Chem. 2002;277:1013–1020. [Abstract] [Google Scholar]
- Hink HU, Santanam N, Dikalov S, McCann L, Nguyen AD, Parthasarathy S, Harrison DG, Fukai T. Peroxidase properties of extracellular superoxide dismutase: role of uric acid in modulating in vivo activity. Arterioscler Thromb Vasc Biol. 2002;22:1402–1408. [Abstract] [Google Scholar]
- Inoue K, Kuramoto N, Sugiyama C, Taniura H, Sakata K, Fujinami Y, Ogita K, Yoneda Y. Fos-B expression is required for polyamine-induced increase in nuclear activator protein-1 DNA binding in discrete structures of murine brain. J Neurosci Res. 2003;74:199–209. [Abstract] [Google Scholar]
- Schabbauer G, Schweighofer B, Mechtcheriakova D, Lucerna M, Binder BR, Hofer E. Nuclear factor of activated T cells and early growth response-1 cooperate to mediate tissue factor gene induction by vascular endothelial growth factor in endothelial cells. Thromb Haemost. 2007;97:988–997. [Europe PMC free article] [Abstract] [Google Scholar]
- Shin JW, Min M, Larrieu-Lahargue F, Canron X, Kunstfeld R, Nguyen L, Henderson JE, Bikfalvi A, Detmar M, Hong YK. Prox1 promotes lineage-specific expression of fibroblast growth factor (FGF) receptor-3 in lymphatic endothelium: a role for FGF signaling in lymphangiogenesis. Mol Biol Cell. 2006;17:576–584. [Europe PMC free article] [Abstract] [Google Scholar]
- Dammann R, Yang G, Pfeifer GP. Hypermethylation of the cpG island of Ras association domain family 1A (RASSF1A), a putative tumor suppressor gene from the 3p21.3 locus, occurs in a large percentage of human breast cancers. Cancer Res. 2001;61:3105–3109. [Abstract] [Google Scholar]
- Farooqui R, Zhu S, Fenteany G. Glycogen synthase kinase-3 acts upstream of ADP-ribosylation factor 6 and Rac1 to regulate epithelial cell migration. Exp Cell Res. 2006;312:1514–1525. [Abstract] [Google Scholar]
- Dowdy SC, Gostout BS, Shridhar V, Wu X, Smith DI, Podratz KC, Jiang SW. Biallelic methylation and silencing of paternally expressed gene 3 (PEG3) in gynecologic cancer cell lines. Gynecol Oncol. 2005;99:126–134. [Abstract] [Google Scholar]
- Pinson L, Auge J, Audollent S, Mattei G, Etchevers H, Gigarel N, Razavi F, Lacombe D, Odent S, Le Merrer M, Amiel J, Munnich A, Meroni G, Lyonnet S, Vekemans M, Attie-Bitach T. Embryonic expression of the human MID1 gene and its mutations in Opitz syndrome. J Med Genet. 2004;41:381–386. [Europe PMC free article] [Abstract] [Google Scholar]
Articles from The American Journal of Pathology are provided here courtesy of American Society for Investigative Pathology
Full text links
Read article at publisher's site: https://doi.org/10.2353/ajpath.2008.080119
Read article for free, from open access legal sources, via Unpaywall:
https://europepmc.org/articles/pmc2543061?pdf=render
Free after 12 months at ajp.amjpathol.org
http://ajp.amjpathol.org/cgi/content/full/173/4/915
Free after 12 months at ajp.amjpathol.org
http://ajp.amjpathol.org/cgi/reprint/173/4/915.pdf
Free to read at ajp.amjpathol.org
http://ajp.amjpathol.org/cgi/content/abstract/173/4/915
Citations & impact
Impact metrics
Citations of article over time
Alternative metrics
Smart citations by scite.ai
Explore citation contexts and check if this article has been
supported or disputed.
https://scite.ai/reports/10.2353/ajpath.2008.080119
Article citations
Inside the genome: understanding genetic influences on oxidative stress.
Front Genet, 15:1397352, 25 Jun 2024
Cited by: 0 articles | PMID: 38983269 | PMCID: PMC11231378
Review Free full text in Europe PMC
Longitudinal analysis of the lung proteome reveals persistent repair months after mild to moderate COVID-19.
Cell Rep Med, 5(7):101642, 08 Jul 2024
Cited by: 2 articles | PMID: 38981485 | PMCID: PMC11293333
Measurement of superoxide dismutase: clinical usefulness for patients with anti-neutrophil cytoplasmic antibody-associated vasculitis.
Adv Rheumatol, 63(1):28, 28 Jun 2023
Cited by: 0 articles | PMID: 37381048
Cross-tissue omics analysis discovers ten adipose genes encoding secreted proteins in obesity-related non-alcoholic fatty liver disease.
EBioMedicine, 92:104620, 22 May 2023
Cited by: 8 articles | PMID: 37224770 | PMCID: PMC10277924
A Review of Bioactive Compounds and Antioxidant Activity Properties of Piper Species.
Molecules, 27(19):6774, 10 Oct 2022
Cited by: 6 articles | PMID: 36235309 | PMCID: PMC9573611
Review Free full text in Europe PMC
Go to all (79) article citations
Other citations
Data
Similar Articles
To arrive at the top five similar articles we use a word-weighted algorithm to compare words from the Title and Abstract of each citation.
Extracellular superoxide dismutase in the airways of transgenic mice reduces inflammation and attenuates lung toxicity following hyperoxia.
J Clin Invest, 103(7):1055-1066, 01 Apr 1999
Cited by: 208 articles | PMID: 10194479 | PMCID: PMC408251
Extracellular superoxide dismutase protects against pulmonary emphysema by attenuating oxidative fragmentation of ECM.
Proc Natl Acad Sci U S A, 107(35):15571-15576, 16 Aug 2010
Cited by: 131 articles | PMID: 20713693 | PMCID: PMC2932580
Extracellular superoxide dismutase increased the therapeutic potential of human mesenchymal stromal cells in radiation pulmonary fibrosis.
Cytotherapy, 19(5):586-602, 15 Mar 2017
Cited by: 26 articles | PMID: 28314668
Extracellular Superoxide Dismutase: Growth Promoter or Tumor Suppressor?
Oxid Med Cell Longev, 2016:3612589, 12 May 2016
Cited by: 30 articles | PMID: 27293512 | PMCID: PMC4880707
Review Free full text in Europe PMC
Funding
Funders who supported this work.
NHLBI NIH HHS (6)
Grant ID: HL390006
Grant ID: P01 HL058000
Grant ID: HL58000
Grant ID: U01 HL080711
Grant ID: HL59248
Grant ID: UO1 HL80711-01
NIBIB NIH HHS (1)
Grant ID: R21 EB006418