Abstract
Free full text

VE-PTP maintains the endothelial barrier via plakoglobin and becomes dissociated from VE-cadherin by leukocytes and by VEGF
Abstract
We have shown recently that vascular endothelial protein tyrosine phosphatase (VE-PTP), an endothelial-specific membrane protein, associates with vascular endothelial (VE)–cadherin and enhances VE-cadherin function in transfected cells (Nawroth, R., G. Poell, A. Ranft, U. Samulowitz, G. Fachinger, M. Golding, D.T. Shima, U. Deutsch, and D. Vestweber. 2002. EMBO J. 21:4885–4895). We show that VE-PTP is indeed required for endothelial cell contact integrity, because down-regulation of its expression enhanced endothelial cell permeability, augmented leukocyte transmigration, and inhibited VE-cadherin–mediated adhesion. Binding of neutrophils as well as lymphocytes to endothelial cells triggered rapid (5 min) dissociation of VE-PTP from VE-cadherin. This dissociation was only seen with tumor necrosis factor α–activated, but not resting, endothelial cells. Besides leukocytes, vascular endothelial growth factor also rapidly dissociated VE-PTP from VE-cadherin, indicative of a more general role of VE-PTP in the regulation of endothelial cell contacts. Dissociation of VE-PTP and VE-cadherin in endothelial cells was accompanied by tyrosine phoshorylation of VE-cadherin, β-catenin, and plakoglobin. Surprisingly, only plakoglobin but not β-catenin was necessary for VE-PTP to support VE-cadherin adhesion in endothelial cells. In addition, inhibiting the expression of VE-PTP preferentially increased tyrosine phosphorylation of plakoglobin but not β-catenin. In conclusion, leukocytes interacting with endothelial cells rapidly dissociate VE-PTP from VE-cadherin, weakening endothelial cell contacts via a mechanism that requires plakoglobin but not β-catenin.
Recruitment of leukocytes into sites of inflammation is very efficiently regulated by the endothelium. It provides a cascade of adhesion and signaling molecules, such as selectins, chemokines, and integrin ligands (1), that attract and capture leukocytes, and it provides well controlled but not yet fully understood mechanisms that open a path for leukocytes through the endothelial cell layer and the underlying basement membrane (2, 3).
Although the molecular mechanisms mediating the capture, activation, and migration of leukocytes are well characterized, the actual diapedesis step, the migration of leukocytes through the blood vessel wall, is less well understood. There is good evidence that leukocytes can use two different pathways, either through the junctions of neighboring endothelial cells or through the body of single endothelial cells (4). The transcellular pathway was documented in vivo (5) as well as in vitro (6, 7); however, quantitative comparison of both pathways showed that, at least in vitro, the junctional route seems to be the major pathway (8), even though the importance of the transcellular pathway may vary depending on the vascular bed from which the endothelial cells originate (7).
The majority of the endothelial membrane proteins that participate in leukocyte diapedesis are found at endothelial cell contacts. Platelet endothelial cell adhesion molecule (PECAM-1) (9), the small family of tight junction–associated junctional adhesion molecules (JAMs) (10), the endothelial cell–selective adhesion molecule (ESAM) (11), CD99 (12, 13), and CD99L2 (14) are all required in vivo for the migration of leukocytes through the vessel wall, as was demonstrated either by blocking antibodies and/or by analyzing gene-deficient mice. Diapedesis was described as a process of sequential steps, with PECAM-1 being important for the entry of leukocytes into the contact area between endothelial cells and CD99 being required for the movement through the endothelial cell layer, as suggested from in vitro transmigration experiments (12, 15). In vivo, it was shown that mice deficient for PECAM-1 accumulate leukocytes between the endothelium and basement membrane, whereas leukocytes become trapped between endothelial contacts in JAM-A–deficient mice (16).
In contrast, vascular endothelial (VE)–cadherin acts as a barrier in leukocyte diapedesis. It is of major importance for the stability of endothelial cell contacts (17–19), and antibodies against VE-cadherin can disrupt endothelial junctions in vivo (20) and can enhance leukocyte recruitment into inflamed peritoneum (21). Thus, it is reasonable to assume that homophilic VE-cadherin trans interactions need to be opened to allow leukocytes to migrate through the endothelial cell barrier. Indeed, it has been described that VE-cadherin moves away from endothelial cell contacts at sites of leukocyte diapedesis, whereas other adhesion molecules such as intercellular adhesion molecule (ICAM) 1 and PECAM-1 form a ringlike structure surrounding leukocytes at such sites (22–24).
Tyrosine phosphorylation of adherens junction proteins has been correlated with the loosening of cadherin-mediated adhesion in various cell types (25–27). For endothelial cells, soluble factors that increase vascular permeability, such as histamine, thrombin, and vascular endothelial growth factor (VEGF), as well as C5a-activated neutrophils, were shown to affect tyrosine phosphorylation of various components of the VE-cadherin–catenin complex (28–31). In addition, the docking of leukocytes to endothelial cells was found to stimulate tyrosine phosphorylation of cortactin, which was demonstrated to be essential for transmigration (32–34). Very recently, it was shown that the binding of leukocytes to ICAM-1 stimulated tyrosine phosphorylation of VE-cadherin. Intriguingly, VE-cadherin mutant proteins deficient for certain tyrosine residues inhibited leukocyte diapedesis (35, 36).
Vascular endothelial protein tyrosine phosphatase (VE-PTP) is an endothelial-specific membrane protein that can bind to the endothelial tyrosine kinase receptor Tie-2 (37). VE-PTP is essential for vascular remodeling, and gene-disrupted mice die at E9.5 during embryonic development (38, 39). We found that this tyrosine phosphatase associates specifically with VE-cadherin and enhances the adhesive function of VE-cadherin when cotransfected in Chinese hamster ovary (CHO) cells (40). Whether VE-PTP is indeed relevant for the function of VE-cadherin in endothelial cells has not yet been analyzed.
In this paper, we show that VE-PTP is indeed essential for the maintenance of endothelial cell contact integrity and for the adhesive function of VE-cadherin in endothelial cells. Consequently, knocking down VE-PTP expression by RNA interference increased diapedesis of leukocytes. Furthermore, docking of leukocytes to endothelial cells triggered the dissociation of VE-PTP from VE-cadherin within minutes, suggesting a mechanism that allows leukocytes to reduce the adhesive function of VE-cadherin and, thereby, to support the opening of endothelial cell contacts. Interestingly, VEGF triggers the dissociation of VE-PTP and VE-cadherin with similar kinetics. Searching for adherens junction proteins in endothelial cells, which could mediate the effect of VE-PTP on VE-cadherin, we found that it is plakoglobin but not β-catenin that is required for VE-PTP to support VE-cadherin–mediated adhesion.
RESULTS
Redistribution of VE-PTP to endothelial cell contacts and increased VE-cadherin association with higher cell density
Because we had shown previously that VE-PTP supports VE-cadherin function when exogenously expressed in transfected cells (40), we expected it to be expressed at endothelial cell contacts. Surprisingly, VE-PTP was hardly detectable at cell contacts of mouse bEnd.3 endothelioma cells when recently confluent cells were analyzed. Instead, VE-PTP was found at an intracellular compartment located close to the nucleus (Fig. 1 A). Staining of this compartment for VE-PTP was gradually lost with increasing confluence, whereas cell contact staining increased (Fig. 1 A). At 8 d after plating (cells had been plated at a 12.5-fold lower density than normal; see Materials and methods), VE-PTP was much less visible at intracellular sites and colocalized with VE-cadherin at cell contacts (Fig. 1, A and B). Immunoprecipitates of VE-PTP contained considerably more VE-cadherin when obtained from cells analyzed 8 d after plating compared with cells at 5 d after plating, although similar amounts of VE-PTP and VE-cadherin (standardized to the protein content of cell lysates) were expressed in both cell samples (Fig. 2 A). A similar result was obtained for human umbilical vein endothelial cells (HUVECs) plated at the same cell density but grown for 3, 4, and 5 d (Fig. 2 B). Thus, without affecting overall cellular expression levels, increasing cell density is accompanied by increasing amounts of VE-PTP at cell contacts and more VE-PTP associated with VE-cadherin.
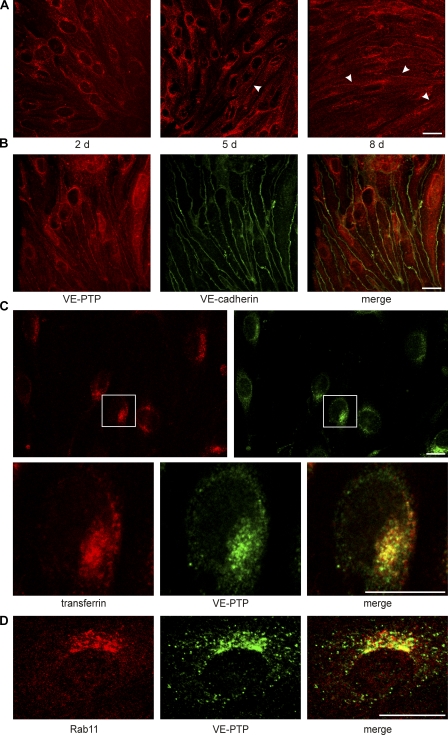
VE-PTP redistributes from an endosomal recycling compartment to endothelial cell contacts with increasing cell confluence. (A) Indirect immunofluorescence staining of mouse bEnd.3 endothelioma of increasing cell confluence (days after seeding are indicated) with an mAb against VE-PTP. VE-PTP was found in cell contacts with increasing cell confluence (arrowheads). (B) Fully confluent bEnd.3 cells were doublestained for VE-PTP (red) and VE-cadherin (green). Merged image is shown on the right. (C) Subconfluent bEnd.3 cells were allowed to endocytose Texas red–conjugated mouse transferrin for 30 min. Subsequently, fixed cells were stained for VE-PTP (green). The boxed cell is shown below at higher magnification. (D) Subconfluent bEnd.3 cells were doublestained for the recycling endosome marker Rab11 (red) and VE-PTP (green). Merged image is shown on the right. Visualization for all micrographs was done by confocal laser scanning microscopy. Each experiment is representative for three (A, B, and D) and two (C) independent experiments. Bars, 20 μm.
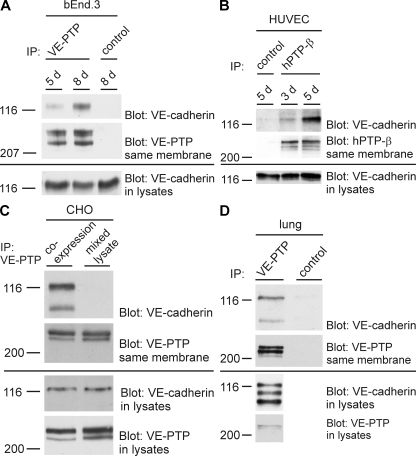
VE-PTP association with VE-cadherin increases with endothelial cell confluence and association occurs in vivo. bEnd.3 cells (A) and HUVECs (B) of increasing cell confluence (days after seeding are indicated above) were subjected to immunoprecipitations for VE-PTP (or human homologue hPTPβ), followed by immunoblotting for VE-cadherin and VE-PTP, as indicated on the right. Aliquots of cell lysates with identical protein content were immunoblotted directly for VE-cadherin (bottom). Molecular weight markers are indicated (left). Preimmune serum was used for negative controls. (C) CHO coexpressing VE-PTP and VE-cadherin (coexpression) or mixed lysates of CHO expressing either only VE-PTP or only VE-cadherin (mixed lysate) were subjected to immunoprecipitations for VE-PTP and subsequent immunoblotting, as described in A. (D) Lungs of mice were lysed and subjected to immunoprecipitation for VE-PTP and subsequent immunoblotting, as described in A. Each experiment is representative for three (A, B, and C) and five (D) independent experiments.
Association of VE-PTP and VE-cadherin could also be verified in vivo, as shown by coimmunoprecipitations from lysates of mouse lung homogenates (Fig. 2 D). We could demonstrate that VE-PTP–VE-cadherin association occurs in intact cells and not just subsequently to the lysis procedure, because both proteins were coprecipitated from lysates of CHO cells that coexpressed the proteins but not from mixed lysates of two CHO cell lines, each expressing only one of the two proteins (Fig. 2 C).
To characterize the intracellular compartment that accumulates VE-PTP at low cell density, we allowed endothelial cells to endocytose Texas red–labeled transferrin for 30 min and costained the cells with antibodies against VE-PTP. As shown in Fig. 1 C, a large fraction of the VE-PTP–containing vesicles colocalized with endocytic vesicles. Comparison with Rab11, a marker for the endocytic recycling compartment, again showed a partial overlap with the staining for VE-PTP (Fig. 1 D). Thus, VE-PTP seems to reside in an endocytic compartment until it is translocated to endothelial cell contacts once the junctions have matured.
VE-PTP is required for endothelial cell contact integrity and VE-cadherin function
To investigate the relevance of VE-PTP for endothelial cell contact integrity, we inhibited the expression of VE-PTP in endothelial cells by small interfering RNA (siRNA). As shown in immunoblots (Fig. 3 A), with this technique we could inhibit the expression of VE-PTP in bEnd.5 mouse endothelioma cells by up to 95% and in HUVECs by up to 90% when compared with negative control siRNA (Fig. 3 A). VE-PTP staining was undetectable in endothelioma cells after siRNA (not depicted). Silencing of VE-PTP did not cause loss of confluence of endothelial cells, and immunofluorescence staining for VE-cadherin, JAM-A, ESAM, and PECAM-1 at cell contacts was essentially unchanged (Fig. S1, available at http://www.jem.org/cgi/content/full/jem.20080406/DC1). Using this technique, we tested whether inhibition of VE-PTP expression would affect the permeability of a monolayer of mouse endothelioma cells for the diffusion of 250 kD FITC-dextran. bEnd.5 cells transfected with the VE-PTP–specific siRNA or with a negative control siRNA were plated on Transwell filters for 24 h before the assay. As shown in Fig. 3 B, inhibition of VE-PTP expression by siRNA increased the cell layer permeability by 60 ± 15% as compared with the control. Thus, VE-PTP is required for the maintenance of cell contact integrity in endothelial cells.
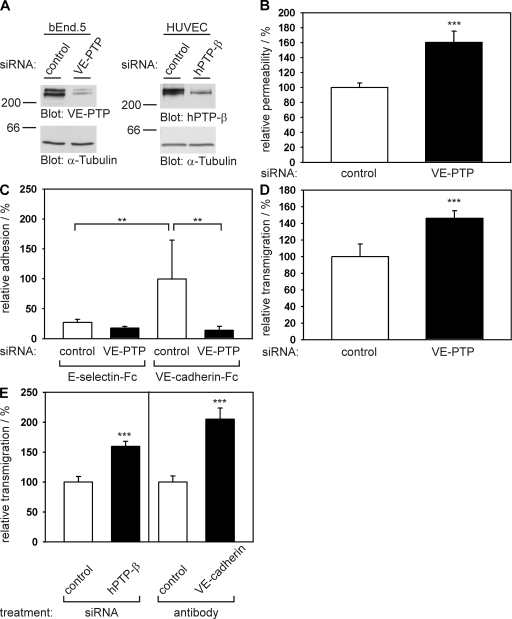
Knocking down VE-PTP expression in endothelial cells by siRNA decreases cell contact integrity and VE-cadherin–mediated adhesion and increases neutrophil transmigration. (A) Mouse bEnd.5 endothelioma cells and HUVECs were transfected with control siRNAs (control) or with siRNAs directed against VE-PTP or hPTP-β, respectively. 24 h later, cell lysates were immunoblotted with pAbs against mouse VE-PTP that cross react with human hPTP-β. Equal loading was controlled by blotting for tubulin (bottom). Molecular weights are shown. (B) Paracellular permeability for 250 kD FITC-dextran was determined for bEnd.5 cells, transfected either with control siRNAs or with siRNAs directed against VE-PTP and cultured on Transwell filters. Permeability of control-transfected bEnd.5 cells was set to 100% (n = 6 for each group; control = 1.44 × 105 ± 8.7 × 103 fluorescence units). (C) bEnd.5 cells were transfected with control siRNA or with siRNA directed against VE-PTP, and adhesion to immobilized VE-cadherin–Fc and E-selectin–Fc was determined by counting adherent cells under a microscope (n = 6 for each group). Control binding to VE-cadherin–Fc was 188 ± 111 cells/area and was set as 100%. (D) Mouse neutrophils were allowed to transmigrate toward the chemokine KC through a monolayer of bEnd.5 cells on Transwell filters transfected either with control siRNA or with siRNA directed against VE-PTP. The number of neutrophils migrated across control-transfected cells was set to 100% (n = 6 for each group; control = 3.6 × 104 ± 5.5 × 103 transmigrated cells). (E) Transmigration of human neutrophils through monolayers of HUVECs on coverslips that were either transfected with control siRNAs or with siRNAs directed against hPTP-β (left), or that were pretreated with a control antibody or an adhesion blocking mAb against human VE-cadherin (right; n = 8–10 for the various groups). In each case, the number of neutrophils migrating under negative control conditions was set to 100%. **, P < 0.01; ***. P < 0.001. Transmigrated cells per area were 23.8 ± 2.2 for control (left) and 17.2 ± 1.8 for control (right). Each experiment is representative of eight (B), five (C and D), and 3 (E) independent experiments. Analysis as in A was done for each siRNA experiment in the paper and was performed at least 40 times. Results are shown as means ± SEM.
To determine whether VE-PTP affects endothelial cell contacts directly via acting on VE-cadherin, we tested whether inhibiting the expression of VE-PTP in endothelial cells would affect the binding of these cells to immobilized VE-cadherin–Fc fusion protein. For negative controls we immobilized E-selectin–Fc. No specific binding of endothelial cells to E-selectin–Fc was observed, whereas strong binding was seen to VE-cadherin–Fc and this binding was reduced to background levels by siRNA for VE-PTP (Fig. 3 C).
Because we know that VE-cadherin represents a barrier for extravasating neutrophils (21), we tested whether the inhibition of VE-PTP expression would enhance the migration of neutrophils across a monolayer of endothelial cells. At 24 h before the transmigration assay, endothelial cells were transfected with VE-PTP or control siRNAs. Inhibition of VE-PTP in mouse endothelioma cells increased transendothelial migration of mouse neutrophils by 44 ± 9% when compared with control transfected cells (Fig. 3 D). Similar results were obtained for HUVECs. hPTP-β (the human homologue of VE-PTP) siRNA increased transmigration of human neutrophils by 60 ± 8% compared with negative control siRNA (Fig. 3 E). This was quite a substantial increase, because an adhesion-blocking mAb against human VE-cadherin, known to disrupt endothelial cell junctions, increased transmigration of neutrophils by 105 ± 19% (Fig. 3 E).
Docking of neutrophils and lymphocytes to the surface of endothelial cells triggers the dissociation of VE-PTP from VE-cadherin
The results described above led us to conclude that VE-PTP supports the function of endothelial cells as a barrier for neutrophils, most likely by interacting with VE-cadherin and thereby enhancing its adhesive function. This raised the idea that neutrophils might interfere with the association of VE-PTP with VE-cadherin to open endothelial cell contacts. We looked into this by testing whether the coprecipitation of VE-cadherin with anti–VE-PTP antibodies would be affected by the binding of neutrophils to the surface of cultured mouse endothelioma cells. Neutrophils were allowed to bind to the monolayer of TNF-α–activated endothelial cells for 5 min. Then the neutrophils were carefully and completely removed by washing the cells with PBS before lysing endothelial cells for immunoprecipitations, because we showed previously that VE-cadherin is easily degraded during experimentation by neutrophil proteases if neutrophils are not removed before analyzing the endothelial cells (41). Endothelial cell lysates were subjected to immunoprecipitations for VE-PTP, and the amount of VE-cadherin coprecipitated with VE-PTP was analyzed in immunoblots. As shown in Fig. 4 A, the amount of coprecipiated VE-cadherin was reduced by 80% upon docking of neutrophils to the endothelium, whereas the amount of precipitated VE-PTP and the expression levels of VE-cadherin in the cell lysates were unaffected. Of note, aliquots of cell lysates for subsequent VE-cadherin immunoblots were set aside and handled in the same way as the aliquots that were subjected to immunoprecipitations. Similar experiments were performed with ovalbumin-specific antigen-stimulated T cells, resulting in a 75% reduction of coprecipitated VE-cadherin upon 5 min of binding of T cells to the endothelium (Fig. 4 B). Thus, neutrophils as well as lymphocytes reduce the association of VE-cadherin with VE-PTP within minutes.
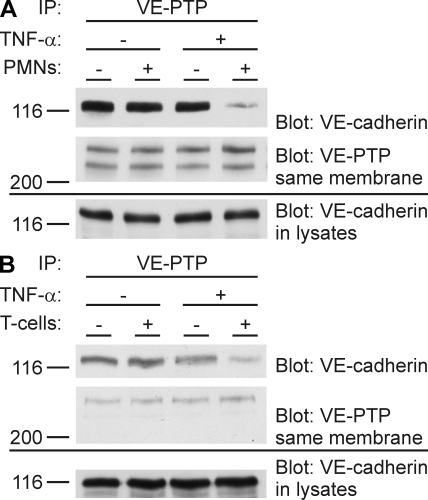
Leukocytes binding to endothelial cells rapidly dissociate VE-cadherin from VE-PTP in a TNF-α–dependent manner. Mouse neutrophils (A) or antigen-stimulated ovalbumin-specific mouse T cells (B) were added to 6-h TNF-α–treated or untreated, highly confluent bEnd.5 cells, and no leukocytes were added to negative control cells. After 5 min, leukocytes were removed by washing with PBS, and VE-PTP was immunoprecipitated from endothelial cells and analyzed by immunoblotting for coprecipitated VE-cadherin or VE-PTP (as indicated on the right). To exclude proteolysis as a potential reason for the weakening of the VE-cadherin signal, aliquots of cell lysates were set aside and kept under identical conditions as lysates subjected to immunoprecipitations before direct analysis by immunoblotting (bottom). Each experiment is representative of at least 15 (A) and 3 (B) independent experiments. Molecular weights are shown.
Leukocyte-triggered dissociation of VE-PTP and VE-cadherin is only seen in TNF-α–activated endothelial cells
We tested whether leukocyte-triggered dissociation of the VE-PTP–VE-cadherin complex would be detectable for resting as well as for TNF-α–activated endothelial cells. To this end, either resting or 6-h TNF-α–activated endothelial cells were incubated with mouse neutrophils for 5 min, and VE-cadherin coprecipitation was probed as described above. Dissociation of VE-PTP from VE-cadherin was only observed for activated endothelial cells but not for resting endothelium (Fig. 4 A). Similar results were observed with lymphocytes (Fig. 4 B). TNF-α was carefully removed from endothelial cells by washing the cells before adding the leukocytes. Our results show that leukocytes trigger the dissociation of VE-PTP from VE-cadherin and, thereby, the weakening of VE-cadherin function only in endothelial cells that are actively supporting adhesion.
None of the known endothelial adhesion receptors for leukocytes are required for leukocyte-triggered dissociation of the VE-PTP–VE-cadherin complex
To test whether any of the known endothelial adhesion receptors for leukocytes would be required for leukocytes to dissociate the VE-PTP–VE-cadherin complex, we analyzed endothelioma cells that had been established from mice deficient for both E- and P-selectin, for both ICAM-1 and -2, or for PECAM-1. We found that neutrophils or lymphocytes still triggered dissociation of the complex despite the lack of these adhesion molecules (Fig. 5 A). Similarly, leukocyte-triggered dissociation of VE-PTP from VE-cadherin was also observed with endothelial cells deficient for only ICAM-1 or only ICAM-2 (not depicted). In addition, antibodies against CD99 that block neutrophil and lymphocyte extravasation in vivo and transendothelial migration of leukocytes in vitro (13, 14) were unable to inhibit the leukocyte-induced dissociation of the VE-PTP–VE-cadherin complex (Fig. 5 B). Thus, either different endothelial cell–surface receptors are involved in this process, or soluble mediators released by leukocytes may trigger the complex dissociation. Oxygen radicals potentially released by neutrophils could be excluded as relevant in this assay because incubating neutrophils with endothelial cells in the presence of superoxide dismutase and catalase did not inhibit leukocyte-triggered dissociation of VE-cadherin and VE-PTP (not depicted). Furthermore, “conditioned” medium taken from endothelial cells that had been incubated with neutrophils, when transferred to new endothelial cell layers, did not have any dissociation activity, arguing against a soluble factor that would trigger the effect (not depicted). Thus, it is likely that endothelial receptors different from the ones known to mediate adhesion of leukocytes are required for leukocytes to dissociate VE-PTP from VE-cadherin.
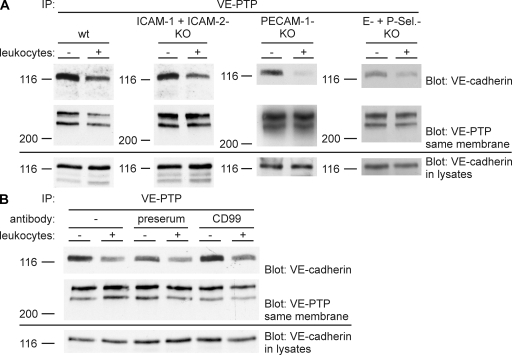
Neither E- and P-selectin, ICAM-1 and -2, or PECAM-1 nor CD99 are required for leukocyte-triggered dissociation of the VE-PTP–VE-cadherin complex. (A) Highly confluent mouse endothelioma cells either double deficient for ICAM-1 and -2, or E- and P-selectin, or deficient for PECAM-1 (as indicated above) were treated for 6 h with TNF-α before adding either T cells (left two panels) or neutrophils (right two panels). After 5 min, leukocytes were removed, and VE-PTP immunoprecipitates and cell lysates were analyzed by immunoblotting for VE-cadherin and VE-PTP or for VE-cadherin, respectively, as described in Fig. 4. (B) TNF-α–treated highly confluent bEnd.5 cells were treated for 30 min either without antibody (−), preimmune antibodies (preserum), or anti-CD99 antibodies (CD99) before the addition of T lymphocytes for 10 min. Lymphocytes were removed, and VE-PTP precipitates and cell lysates were analyzed as described in A. Each experiment is representative of three independent experiments. Molecular weights are shown.
Leukocytes stimulate catenin phosphorylation, and plakoglobin is a substrate for VE-PTP
We tested whether the binding of lymphocytes to TNF-α–activated mouse endothelioma cells would affect tyrosine phosphorylation levels of the components of the VE-cadherin–catenin complex. TNF-α had been washed away before adding the lymphocytes. As shown in Fig. 6 A, we found that VE-cadherin as well as β-catenin and plakoglobin showed increased tyrosine phosphorylation levels upon lymphocyte incubation. In agreement with a recent report (36), tyrosine phosphorylation of β-catenin and plakoglobin were not enhanced if lymphocytes were incubated with resting endothelial cells (not depicted).
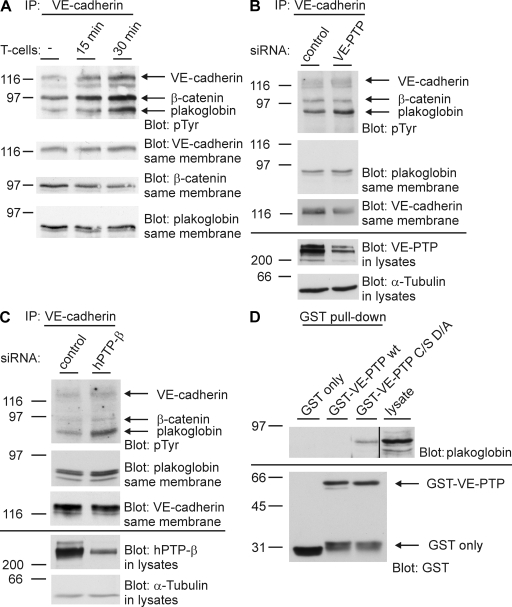
Leukocytes trigger tyrosine phosphorylation of the VE-cadherin–catenin complex, and plakoglobin serves as substrate for VE-PTP. (A) Antigen-stimulated mouse T lymphocytes were allowed to adhere for the indicated times to highly confluent TNF-α–activated bEnd.5 cells. After removal of T cells by washing with PBS, VE-cadherin was immunoprecipitated, and isolated VE-cadherin–catenin complexes were analyzed by immunoblotting with antiphosphotyrosine antibodies (pTyr) and antibodies against VE-cadherin, β-catenin, and plakoglobin (as indicated). (B) bEnd.5 cells were either transfected with control siRNAs or with siRNAs directed against VE-PTP. 24 h later, VE-cadherin was immunoprecipitated, and immunocomplexes (top two panels) and cell lysates (bottom two panels) were analyzed by immunoblotting with antibodies against phosphotyrosine (pTyr), plakoglobin, VE-cadherin, VE-PTP, and tubulin (as indicated). (C) HUVECs, instead of bEnd.5 cells, were analyzed as in B. (D) Mouse L929 fibroblasts, pretreated with 1 mM pervanadate for 30 min and 10 μM of proteasome inhibitor for 2–3 h (to increase plakoglobin expression), were subjected to substrate trapping pull downs with GST (GST only), GST–VE-PTP WT, or GST–VE-PTP C/S D/A, followed by immunoblotting for plakoglobin and GST, as indicated on the right. The black line indicates that intervening lanes have been spliced out. Each experiment is representative for five (A and B), three (C), and two (D) independent experiments. Molecular weights are shown.
To test whether VE-PTP could affect the tyrosine phosphorylation levels of components of the VE-cadherin–catenin complexes, we knocked down the expression of VE-PTP by siRNA in mouse endothelioma cells. As shown in Fig. 6 B, this treatment enhanced the tyrosine phosphorylation of plakoglobin by 40% when compared with the control siRNA treatment, whereas no effect was seen for VE-cadherin and β-catenin. Similar results were obtained with HUVECs, except that the increase in plakoglobin tyrosine phosphorylation was even stronger (80%) and a very slight increase in the phosphorylation of VE-cadherin and β-catenin was seen (Fig. 6 C). Thus, plakoglobin is the major component of the VE-cadherin–catenin complex whose tyrosine phosphorylation level can be affected by the lack of VE-PTP, even in the absence of exogenous stimuli such as leukocytes, that could actively trigger tyrosine phosphorylation by activating kinases.
We tested whether plakoglobin is indeed a direct substrate for VE-PTP in glutathione S-transferase (GST) pull-down experiments using a VE-PTP “substrate-trapping” mutant carrying the two point mutations C/S and D/A. Tyrosine phosphatase domains carrying these mutations in their active center lack catalytic activity but have preserved substrate binding activity (42). Indeed, we were able to pull down plakoglobin from lysates of L929 mouse fibroblasts, whereas no signal was detected with the WT form of VE-PTP or with GST alone (Fig. 6 D). L929 cells do not express cadherins, excluding the possibility that plakoglobin was possibly pulled down indirectly in the context of a cadherin–catenin complex.
VE-PTP–mediated support of VE-cadherin function requires plakoglobin but not β-catenin
To investigate which molecular entities of the VE-cadherin–catenin complex are required for VE-PTP to enhance VE-cadherin function, we generated three different types of triple-transfected CHO cell clones that stably expressed VE-PTP under a mifepristone-inducible promoter, and a mutant of VE-cadherin together with VEGFR-2 under constitutive promoters. VEGFR-2 was required for tyrosine phosphorylation of VE-cadherin (39). The three different mutant proteins comprised a form of VE-cadherin in which all eight tyrosine residues within the cytoplasmic tail were replaced by phenylalanines (VE-YallF) and two truncated forms of VE-cadherin lacking either the last 83 (VE-Δ83) or the last 42 (VE-Δ42) amino acids of the intracellular domain, thus deleting the β-catenin/plakoglobin binding site. As read out for VE-cadherin function, we analyzed cell aggregation of the transfected CHO cells. We have shown previously that induction of VE-PTP in such triple-transfected CHO cells leads to a decrease of cell monolayer permeability if WT VE-cadherin is coexpressed (40). In agreement with this, we found now that induction of VE-PTP clearly increased cell aggregation of cells that expressed the WT form of VE-cadherin (Fig. 7 A). Interestingly, a similar effect was seen in cells that expressed the VE-YallF mutant (Fig. 7 A), demonstrating that the tyrosine residues in VE-cadherin are not essential for the effect of VE-PTP on VE-cadherin. CHO cells expressing truncated VE-cadherin aggregated with lower efficiency, but were still able to aggregate in a VE-cadherin–dependent manner when compared with nontransfected cells (Fig. 7 B). However, the enhancing effect of VE-PTP was lost in CHO cells that expressed the VE-Δ42 or the VE-Δ83 truncation mutants (Fig. 7 B). This suggests that VE-cadherin anchoring to the catenins is required for VE-PTP–mediated enhancement of VE-cadherin–adhesive function.
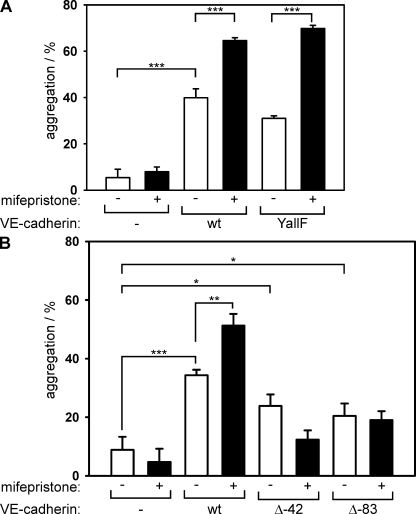
VE-cadherin–catenin anchoring but no VE-cadherin tyrosine residues are required for VE-PTP support of adhesion in transfected CHO cells. (A) Untransfected CHO cells (−); triple-transfected CHO cells stably expressing WT–VE-cadherin, VEGFR-2, and inducible VE-PTP (WT); and CHO cells stably transfected with VE-cadherin YallF mutant (all tyrosines mutated to phenylalanines), VEGFR-2, and inducible VE-PTP (YallF) were stimulated for 10 h with mifepristone to induce VE-PTP expression (+) or left unstimulated (−). Subsequently, harvested cells were allowed to aggregate, and the number of cell aggregates was determined in a cell counter (n = 3 for each group). (B) In addition to untransfected and triple-transfected WT controls (as in A), triple-transfected CHO cells expressing, in addition to VEGFR-2 and inducible VE-PTP, either the VE-cadherin Δ42 mutant (lacking the C-terminal 42 amino acids) or the VE-cadherin Δ83 mutant (lacking the C-terminal 83 amino acids) were analyzed in cell aggregation assays under mifepristone-induced (+) and noninduced (−) conditions (n = 3 for each group). *, P < 0.05; **, P < 0.01; ***, P < 0.001. Each experiment is representative of four (A) and two (B) independent experiments. Results are shown as means ± SEM.
This requirement for the catenin-binding domain of VE-cadherin prompted us to test whether plakoglobin and/or β-catenin would be necessary in endothelial cells for the influence of VE-PTP on VE-cadherin function. To this end, we established mouse endothelioma cells from gene-targeted mice lacking either plakoglobin or β-catenin. These cells expressed endothelial-specific markers such as Tie-2, VEGFR-2, PECAM-1, and VE-cadherin (not depicted). With these cells, we tested whether down-regulation of the expression of VE-PTP by siRNA would still affect cell binding to immobilized VE-cadherin–Fc. Surprisingly, we found that reduction of VE-PTP expression in β-catenin–deficient endothelioma cells had a similar inhibitory effect on VE-cadherin–mediated adhesion as in normal endothelioma cells expressing all catenins (Fig. 8 A). In contrast, a strongly reduced inhibitory effect was seen in plakoglobin-deficient cells (Fig. 8 A). Thus, plakoglobin but, surprisingly, not β-catenin is required for VE-PTP to enhance VE-cadherin function. Similarly, plakoglobin was also required for the effect of VE-PTP on endothelial cell permeability, because silencing of VE-PTP increased permeability less dramatically in endothelial cells deficient for plakoglobin than in cells expressing plakoglobin (Fig. 8 B).
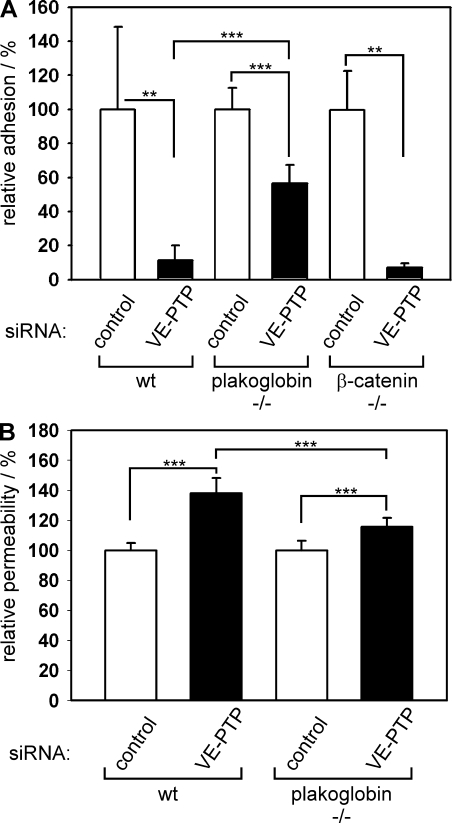
VE-PTP supports VE-cadherin function via plakoglobin, but not β-catenin, and affects endothelial cell permeability via plakoglobin. (A) WT mouse endothelioma cells and endothelioma cells lacking plakoglobin (plakoglobin−/−) or β-catenin (β-catenin−/−) were transfected either with control siRNAs or with siRNAs directed against VE-PTP and were allowed to adhere to immobilized VE-cadherin–Fc. Absolute numbers of controls in cells per area were 577 ± 256 (WT), 1,186 ± 138 (plakoglobin−/−), and 862 ± 179 (β-catenin−/−). (B) Paracellular permeability for 250 kD FITC-dextran was determined for mouse endothelioma cells, transfected as described in A and cultured on Transwell filters. Absolute numbers of fluorescence units for controls were 2.43 × 104 ± 1.16 × 103 (WT) and 4.17 × 104 ± 2.65 × 103 (plakoglobin−/−). Adhesion and permeability, respectively, of control-transfected cells were set to 100% (n = 6 for each group). **, P < 0.01; ***, P < 0.001. Each experiment is representative for three independent experiments. Results are shown as means ± SEM.
VE-PTP affects the association of VE-cadherin with plakoglobin
To find out how VE-PTP supports VE-cadherin function, we tested whether the anchoring of VE-cadherin with the catenins would be affected by VE-PTP. We compared anti–VE-cadherin immunoprecipitates from triple-transfected (VE-cadherin– and VEGFR-2–expressing) CHO cells that were or were not induced for VE-PTP expression by mifepristone. We found that the amount of plakoglobin coprecipiated with VE-cadherin was clearly increased upon induction of VE-PTP, whereas the amount of β-catenin was unchanged (Fig. 9). Similar results were obtained with triple-transfected CHO cells that expressed VE-YallF instead of WT VE-cadherin (Fig. 9). We conclude that VE-PTP stimulates the association of VE-cadherin and plakoglobin and that this effect is independent of tyrosine phosphorylation levels of VE-cadherin.
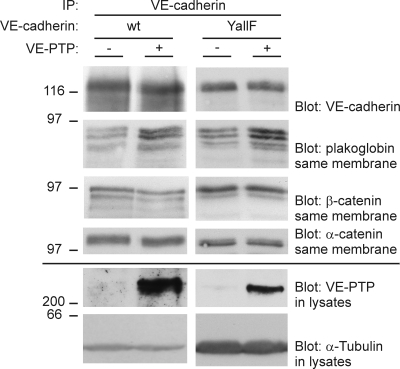
Induction of VE-PTP expression in CHO cells leads to increased association of plakoglobin with VE-cadherin. CHO cells triple-transfected with WT–VE-cadherin, VEGFR-2, and inducible VE-PTP (WT), and CHO cells stably transfected with VE-cadherin YallF mutant (all tyrosines mutated to phenylalanines), VEGFR-2, and inducible VE-PTP (YallF) were either induced for VE-PTP expression (+) or not induced (−). Subsequently, VE-cadherin was immunoprecipitated, and immunocomplexes (top four panels) and cell lysates (bottom two panels) were analyzed in immunoblots for the indicated antigens. This experiment is representative of four independent experiments. Molecular weights are shown.
VEGF stimulates dissociation of the VE-cadherin–VE-PTP complex
VEGF has been reported to stimulate tyrosine phosphorylation of the VE-cadherin–catenin complex and endothelial cell layer permeability in HUVECs (28). We could confirm this for bEnd.5 mouse endothelioma cells (Fig. 10 B). Interestingly, phosphorylation of plakoglobin was clearly much more strongly induced than phosphorylation of β-catenin and VE-cadherin (Fig. 10 B). Of note, in contrast to previous reports, the cells were neither starved in serum-free medium nor pretreated with vanadate or even peroxyvanadate before VEGF treatment and analysis. Thus, we used the same conditions as when incubating the endothelial cells with leukocytes. Similar results were obtained with HUVECs (not depicted). Our results prompted us to test whether VEGF would interfere with the complex between VE-cadherin and VE-PTP. Indeed, we found that VEGF treatment induced dissociation of the complex within minutes (Fig. 10 A), in agreement with the kinetics of tyrosine phosphorylation of the VE-cadherin–catenin complex. Because plakoglobin is necessary for VE-PTP to support cell adhesion, we tested whether it is required for leukocyte- or VEGF-triggered disassociation of VE-cadherin. Using plakoglobin-null endothelioma cells, we found that leukocytes as well as VEGF could still dissociate the VE-PTP–VE-cadherin complex (Fig. 10 C), suggesting that plakoglobin is indeed affected by the dissociation of VE-PTP from VE-cadherin rather than it effecting the dissociation of this complex itself.
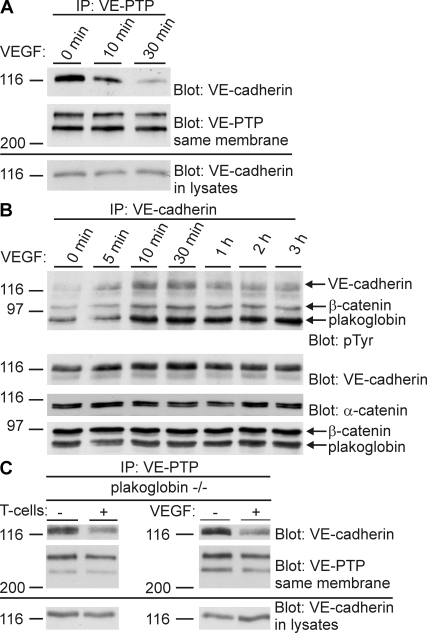
Stimulation of endothelial cells with VEGF dissociates VE-cadherin from VE-PTP and triggers tyrosine phosphorylation of the VE-cadherin–catenin complex. (A) bEnd.5 cells were stimulated with VEGF for the indicated time periods. Subsequently, VE-PTP was immunoprecipitated, and immunocomplexes (top two panels) and cell lysates (bottom panel) were analyzed in immunoblots for VE-cadherin and VE-PTP. (B) After stimulating bEnd.5 cells with VEGF for the indicated time periods, VE-cadherin was immunoprecipitated, and immunocomplexes were analyzed in immunoblots for tyrosine phosphorylation (pTyr), VE-cadherin, α-catenin, β-catenin, and plakoglobin (as indicated). (C) T cells (left) or VEGF (right) were added (+) or not (−) to plakoglobin-null endothelioma cells for 10 min (T cells) or 20 min (VEGF). Subsequently, VE-PTP was immunoprecipitated, and immunocomplexes (top two panels) and cell lysates (bottom panel) were analyzed in immunoblots for VE-cadherin and VE-PTP (as indicated on the right). Each experiment is representative for seven (A), four (B), and two (C) independent experiments. Molecular weights are shown.
DISCUSSION
In this study, we report a novel mechanism for the control of endothelial cell contacts during leukocyte extravasation. We show that VE-PTP is an important component of the VE-cadherin–catenin complex, which is required for the maintenance of the endothelial barrier function. Leukocytes rapidly dissociate VE-PTP from this complex, suggesting that this receptor-type phosphatase represents a switch that leukocytes can use to open endothelial cell contacts. In addition, VE-PTP may be more generally relevant for the regulation of endothelial cell contacts because the vascular permeability–inducing growth factor VEGF is also able to dissociate the phosphatase from VE-cadherin. Importantly, plakoglobin is an essential component of the cadherin complex, which is necessary for VE-PTP to support cell adhesion, whereas neither the tyrosine residues of the intracellular domain of VE-cadherin nor β-catenin are required for this effect of VE-PTP.
Regulation of cadherin adhesive function has been attributed to tyrosine phosphorylation of adherens junction proteins in various cell types (26, 43, 44). Of these proteins, β-catenin and p120 are among the best characterized (45, 46). Several papers describe the dissociation of β-catenin from the respective cadherin as a central step in regulating adhesion. For the vascular system, numerous lines of evidence have demonstrated that tyrosine phosphorylation of adherens junction proteins, especially β-catenin, correlate with the dissociation of endothelial cell contacts. Tyrosine phosphatases that were found to affect the stability of endothelial junctions are the β-catenin–associated SHP-2 (30), and the receptor-type RPTP-μ that directly associates with the cytoplasmic tail of VE-cadherin (47). However, none of them has yet been studied in the context of leukocyte transmigration.
With VE-PTP, we have found the first tyrosine phosphatase that is relevant for the control of endothelial cell contacts during leukocyte transmigration. In addition, we demonstrate that it is plakoglobin that is required for VE-PTP to stimulate VE-cadherin function in endothelial cells, whereas β-catenin is dispensable (Fig. 8). This selectivity for only one of the two catenins that connect VE-cadherin with α-catenin clearly suggests that VE-PTP either affects plakoglobin directly as a substrate or affects factors that indirectly influence plakoglobin. Our results indeed suggest that plakoglobin is a substrate of VE-PTP, because a substrate-trapping mutant of VE-PTP binds to plakoglobin in cadherin-free L929 cell lysates and silencing of VE-PTP in endothelial cells predominantly affects the tyrosine phosphorylation of plakoglobin.
The selective plakoglobin dependence of the VE-PTP influence on VE-cadherin function is remarkable for two reasons. First, in contrast to the numerous reports on the relevance of β-catenin for the regulation of cadherin adhesiveness (45), our results establish a mechanism that regulates VE-cadherin function selectively via plakoglobin. Very few papers have yet analyzed the impact of the phosphorylation of plakoglobin on the association with a cadherin (48). Second, several years ago it was reported that plakoglobin is absent from recently confluent endothelial cell contacts. The synthesis of plakoglobin is up-regulated and becomes assembled with VE-cadherin in junctional complexes only when the cells have reached high confluence after several days of culture (49). It is intriguing, that similar to plakoglobin, VE-PTP is also located at endothelial cell contacts only days after cells have reached confluence. At the same time, VE-PTP is lost from an intracellular location that is at least in part overlapping with the endocytic compartment (Fig. 1). Thus, maturation of endothelial junctions is accompanied by the redistribution of VE-PTP from an endosome-like compartment to cell contacts and by increased association of plakoglobin and VE-PTP with VE-cadherin. We suggest that VE-PTP and plakoglobin are both required for the stabilization of endothelial cell contacts, and VE-PTP exerts this function through plakoglobin.
Despite the selective dependence on plakoglobin, we do not yet precisely know how VE-PTP supports cell contact integrity. It is interesting that the induction of VE-PTP in CHO cells cotransfected with VE-cadherin and VEGFR-2 selectively enhanced the association between plakoglobin and VE-cadherin, whereas no effect was seen for β-catenin. This could suggest that VE-PTP might stabilize the association of plakoglobin with VE-cadherin. However, we were not able to detect in endothelial cells that the dissociation of VE-PTP from VE-cadherin, triggered by leukocytes, would lead to the dissociation of plakoglobin from VE-cadherin (indirectly implicated in the results of Fig. 6 A). Although this discrepancy cannot yet be fully explained, we have to consider that much less VE-PTP than VE-cadherin is expressed in endothelial cells and that it is well possible that a considerable fraction of plakoglobin molecules is associated with VE-cadherin independently of VE-PTP. If this were the case, a large fraction of plakoglobin molecules would perhaps not be immediately affected by the dissociation of VE-PTP, and the small fraction of potentially dissociating plakoglobin molecules might be too small to be detectable in our assays. Nevertheless, regardless of whatever mechanism might be established in the future, plakoglobin is a key to understand how VE-PTP affects VE-cadherin.
Very recently, it was reported that tyrosine phosphorylation of VE-cadherin itself is involved in the transmigration of leukocytes. Lymphocyte and monocyte binding to endothelial cells, as well as cross-linking of ICAM-1, stimulated tyrosine phosphorylation of VE-cadherin. In addition, point-mutated VE-cadherin with certain tyrosine residues replaced by phenylalanine reduced leukocyte transmigration through endothelial cell layers (35, 36). In this context, it is remarkable that induction of VE-PTP in CHO cells still enhanced VE-cadherin function despite the absence of all tyrosine residues in the cytoplasmic domain of VE-cadherin. This demonstrates that yet other substrates besides VE-cadherin exist that mediate the enhancing effect of VE-PTP on VE-cadherin function. However this does not exclude that in endothelial cells in a situation where leukocytes are bound, VE-PTP may contribute to the dephosphorylation of those VE-cadherin tyrosine residues that are relevant for the regulation of VE-cadherin function. Indeed, tyrosine phosphorylation of VE-cadherin is reduced by VE-PTP in COS-7 and CHO cells (40). In addition, we found that inhibiting the expression of VE-PTP in plakoglobin−/− endothelial cells had a much reduced but still significant inhibitory effect on VE-cadherin function, suggesting that besides plakoglobin there are yet other adherens junction proteins that could serve as adhesion-affecting substrates, including VE-cadherin itself.
We were surprised that none of the endothelial adhesion receptors that interact with neutrophils and lymphocytes (ICAM-1, ICAM-2, E- and P-selectin, PECAM-1, and CD99) were essential for leukocyte-induced dissociation of the VE-PTP–VE-cadherin complex. VCAM-1 was not analyzed because it is not of importance for neutrophils. Even the combined deletion of ICAM-1 and -2 or of P- and E-selectin did not abrogate the effect. Soluble factors including oxygen radicals are unlikely to be involved, because scavenging of oxygen radicals did not inhibit the effect. Several potential soluble factors that can be released by neutrophils could be excluded, because lymphocytes had the same activity. Furthermore, the dissociation activity could not be transferred to fresh endothelial cells with medium taken from endothelial cells that had been preincubated with neutrophils. Thus, elucidating the receptor mechanism that transmits the leukocyte-triggered effect on dissociating the VE-PTP–VE-cadherin complex will require more experimental work in the future. Interestingly, we found with the VEGF-receptor a leukocyte-independent mechanism that also triggers the dissociation of VE-PTP from VE-cadherin. Thus, different stimuli that affect endothelial cell contact stability seem to converge on the stability of the same complex. The attractive possibility, however, that VEGF itself might be mediating the leukocyte effect as secreted mediator could be excluded, because blocking anti-VEGF antibodies did not inhibit the complex-dissociating activity of leukocytes (unpublished data).
ICAM-1 cross-linking on resting endothelial cells only increases tyrosine phosphorylation of VE-cadherin, but not of the catenins (36). We could confirm this for the binding of lymphocytes to resting endothelium (unpublished data). In contrast, binding of lymphocytes to TNF-α–activated endothelial cells clearly increased tyrosine phosphorylation of VE-cadherin, β-catenin, and plakoglobin. Thus, leukocyte-induced tyrosine phosphorylation of catenins and dissociation of VE-PTP from VE-cadherin both require preactivation of the endothelium with TNF-α. This further supports the concept that the dissociation of VE-PTP from the VE-cadherin complex indeed participates in leukocyte-induced tyrosine phosphorylation of catenins. In addition, the fact that leukocytes cannot dissociate VE-PTP from VE-cadherin in resting cells suggests that VE-PTP may provide a mechanism that maintains endothelial cell contact integrity in nonactivated endothelial cells that are not actively supporting transmigation. Only in the presence of inflammatory stimuli are leukocytes able to operate this “switch” and destabilize endothelial contacts via dissociation of VE-PTP from VE-cadherin. Whether this switch would be operational without the requirement of TNF activation in high endothelial cells specialized for efficient lymphocyte entry into lymph nodes will be an interesting question to study.
In conclusion, our results establish VE-PTP as a new membrane protein at endothelial junctions that is involved in the regulation of endothelial cell contacts during leukocyte transmigration. Because VE-PTP is the first known endothelial-specific tyrosine phosphatase and because it is a receptor-type protein with a large extracellular domain accessible from within the vasculature, VE-PTP represents a very interesting novel pharmacological target for the treatment of inflammatory diseases.
MATERIALS AND METHODS
Reagents and antibodies.
Transferrin–Texas red (Rockland), soybean trypsin inhibitor (Sigma-Aldrich), DNase (Roche), laminin (Sigma-Aldrich), recombinant human TNF-α (PeproTech), gelatin (Sigma-Aldrich), fluorescent mounting medium (Dako), 250 kD FITC-dextran (Sigma-Aldrich), VEGF165 (PeproTech), and proteasome inhibitor MG 132 (EMD) were all used.
The following antibodies were used: mAb 109.1 against VE-PTP (38); pAb VE–PTP-C against VE-PTP (cross-reactive to the human homologue hPTP-β) (40); mAb 11D4.1 against mouse VE-cadherin and pAb C5 against mouse VE-cadherin (21); pAb against mouse CD99 (13) and mAb 1G8 against ESAM (50); pAb against human ESAM; mAb against PECAM-1 1G5.1 and 5D2.6 (11); pAb against JAM-A (51), mAb cadherin-5 against human VE-cadherin, mAb against β-catenin, mAb against plakoglobin, and mAb against α-catenin (BD Biosciences); pAb C-19 against human VE-cadherin and pAb Z-5 against GST (Santa Cruz Biotechnology, Inc.); pAb against Rab11 (Invitrogen); mAb 4G10 against phosphotyrosine (Millipore); and mAb against α-tubulin (Sigma-Aldrich). Secondary antibodies were purchased from Dianova. Alexa Fluor 488– and Alexa Fluor 568–coupled antibodies were purchased from Invitrogen.
DNA constructs.
Based on the mouse cDNA of VE-cadherin (52) inserted via EcoRI/XbaI sites into pcDNA3, truncation mutants VE-cadherin Δ42 or Δ83 were generated by PCR using the following primers: the sense primer T7 (5′-TAATACGACTCACTATAGGG-3′) and the antisense primers VECP33 (5′-CGTCTAGACTAGGACTCTGCGATGGACTCTGCG-3′) for VE-cadherin Δ42 and VECP32 (5′-CGTCTAGACTATCCGTGCAGTCCAGGCG-3′) for VE-cadherin Δ83. VE-cadherin YallF mutant was generated by using the QuikChange Site-Directed Mutagenesis Kit (Agilent Technologies). GST–VE-PTP and the trapping mutant GST–VE-PTP C/S were previously described (37). The D/A mutation was introduced into GST–VE-PTP C/S by PCR using the following primers: antisense (5′-CTCTGGGACCCCATGGGCTGGCCACACCGTGTAG-3′) and sense (5′-CTACACGGTGTGGCCAGCCCATGGGGTCCCAGAG-3′). GST, GST–VE-PTP, and GST–VE-PTP C/S D/A fusion proteins were expressed in Escherichia coli (strain BL21).
Cell culture.
The following cells were propagated as described: CHO cells (40), CHO cells expressing mouse VE-cadherin (21), bEnd.3 cells (53), bEnd.5 cells (54) and HUVECs (55), E- and P-selectin−/− endothelioma bEndE/P.5 cells (56), ICAM-1 and -2−/− endothelioma bEndI1/2.1 cells (57), PECAM-1−/− endothelioma luEnd.PECAM-1.9 cells (58), and L929 fibroblasts (Sigma-Aldrich). OTII-Ova T cells expressing α and β chains of a TCR specific for peptide 323–339 of ovalbumin in the context of I-A b were isolated from OTII mice (The Jackson Laboratory). Embryonic endothelioma cells were cultured in bEnd.5 growth medium. For stimulation with VEGF, bEnd.5 cells were grown to confluence in DMEM/10%FCS, washed twice with MCDB 131 medium (Invitrogen) containing 1% BSA, and stimulated with 100 ng/ml of recombinant VEGF165 (PeproTech) in MCDB 131 medium with 1% BSA for the time periods indicated in the figures (from 5 min to 3 h). Cells were analyzed by immunoprecipitation.
Generation of stable cell lines.
CHO-πA36#25 cells (expressing VE-PTP upon mifepristone induction) were generated by cotransfecting pSwitch plasmid (hygromycin resistance; Invitrogen) with the expression plasmid pGene/V5-HisA containing the mouse VE-PTP fl cDNA. Co-transfecting CHO-πA36#25 cells with the expression plasmid pCDNA3 (neomycin resistance; Invitrogen) containing mouse VE-cadherin YallF cDNA together with the expression plasmid pEF6 (blasticidin resistance; Invitrogen) containing the mouse VEGFR-2 cDNA gave rise to CHO-YallF. CHO Δ42 and Δ83 were generated by cotransfecting CHO-πA36#25 with the expression plasmid pCDNA3 containing mouse VE-cadherin Δ42 or Δ83 cDNA together with expression plasmid pEF6 containing the mouse VEGFR-2 cDNA. Expression of transfected genes was assessed by FACS analysis. Expression of VE-PTP was induced with mifepristone for 10 h at 37°C. Mifepristone is a synthetic steroid compound driving an inducible expression system (GeneSwitch; Invitrogen).
Generation of plakoglobin−/− and β-catenin−/− endothelioma cell lines.
Plakoglobin−/− and β-cateninfl/Δ endothelioma cells were generated by polyoma middle T immortalization of cell cultures established from plakoglobin-deficient E9.5 mouse embryos, or from primary brain endothelial cell cultures established from newborn β-cateninfl/Δ mice, respectively, according to previously published methods (54, 59). Gene-disrupted mice were provided by R. Kemler (Max-Planck-Institute of Immunobiology, Freiburg, Germany). All mice were housed under pathogen-free conditions at the Max-Planck-Institute's Animal Facility, and were used in accordance with guidelines and under permission of the Regierungspräsidium Münster. Deletion of the floxed β-catenin allele was accomplished by infection with Cre-expressing adenovirus obtained from U. Müller (Friedrich Miescher Institute for Biomedical Research, Basel, Switzerland).
Immunofluorescence staining.
For detection of VE-PTP in endothelioma of increasing confluency, 104 bEnd.3 endothelioma cells were seeded on laminin-coated Transwell filters and stained 2, 5, and 8 d after seeding. Primary antibodies were detected with Cy2- and Cy3-coupled or with Alexa Fluor 488– or Alexa Fluor 568–coupled secondary antibodies. Commercially available antibodies against human VE-PTP could not be used because they gave unspecific signals in immunoprecipiatation and immunofluorescence. To label early endosomes, subconfluent bEnd.3 cells grown on chamber slides for 48 h were serum starved for 30 min and then incubated for 30 min with 100 μg/ml of Texas red–conjugated mouse transferrin in internalization medium (MEM supplemented with 0.8 g/liter NaHCO3, 20 mM Hepes, 1% BSA) at 37°C. Fluorescence signals were detected using a confocal laser scanning microscope (model TSP2; Leica).
Immunoprecipitation and immunoblotting.
For coimmunoprecipitations, cells were lysed in lysis buffer (20 mM imidazole [pH 6.8], 100 mM NaCl, 2 mM CaCl2, 1% Triton X-100, 0.04% NaN3, and 1× Complete EDTA-free protease inhibitor cocktail [Roche]). For detection of phosphotyrosine after immunoprecipitation, cells were lysed in lysis buffer containing 20 mM Tris-HCl (pH 7.4), 150 mM NaCl, 2 mM CaCl2, 1 mM Na3VO4, 1% Triton X-100, 0.04% NaN3, and 1× Complete EDTA-free. At no time were intact cells exposed to vanadate or peroxyvanadate. For coimmunoprecipitations from mouse lung lysates, lungs were homogenized with an Ultra Turrax (IKA-Werke) in three parts of PBS containing 1 mM CaCl2 and 2× Complete EDTA-free protease inhibitor cocktail. Subsequently, one part of 4× lysis buffer (PBS, 4% NP-40, 4 mM dithiothreitol [DTT], 1 mM CaCl2) was added and incubated for 4 h at 4°C. Lysates were centrifuged at 4°C for 30 min or 1 h for lung lysates at 20,000 g, aliquots were set aside for direct blot analysis, and aliquots for immunoprecipitation were incubated for 2 h to overnight at 4°C with protein A–sepharose loaded with the respective antibodies. Immunocomplexes were washed five times with lysis buffer and analyzed by SDS-PAGE. Total cell or organ lysates (3 × 105 cells/lane, 50 μg of protein/lane) or immunoprecipitated material were separated by electrophoresis on 6% (immunoprecipitation) or 8% (direct immunoblots of cell lysates) SDS-PAGE and transferred to nitrocellulose (Schleicher & Schuell) by wet blotting. Blots were analyzed as previously described (51). For detection of phosphotyrosine, milk powder in the blocking buffer was replaced by 2% BSA, and 200 μM Na3VO4 was added.
VE-PTP substrate trapping.
For substrate trapping pull-down experiments, three 15-cm plates of confluent L929 cells were pretreated with 10 μM of proteasome inhibitor for 2–3 h (to increase plakoglobin expression levels) and 1 mM pervanadate for 30 min and lysed (20 mM Tris/HCl [pH 7.5], 100 mM NaCl, 1 mM EDTA, 1% Triton X-100, 10% glycerine, 5 mM iodoacetic acid [IAA], 1× Complete EDTA-free protease inhibitor cocktail [Roche]) for 30 min at 4°C. Subsequently, 10 mM DTT was added to inactivate IAA. After centrifugation at 14,000 g for 30 min at 4°C, cleared lysates were incubated overnight with 7 μg GST, GST–VE-PTP, or GST–VE-PTP C/S D/A bound to glutathione-sepharose, as previously described (42). Beads were washed four times with 5 ml and five times with 1 ml of lysis buffer and were analyzed by SDS-PAGE and immunoblotting.
RNA interference.
For RNA interference of VE-PTP and hPTP-β expression, the following siRNAs were used: VE-PTP-34, 5′-CCUCACUGAGGGUAACAGU-3′ (targeting VE-PTP); and siPTP-82, 5′-GACAGUAUGAGGUGGAAGU-3′ (targeting hPTP-β; Ambion). For negative controls, an siRNA was used that does not target any known mammalian gene (5′-UUCUCCGAACGUGUCACGU-3′; QIAGEN). Routinely, 106 bEnd.5 cells or HUVECs were transfected with 3 or 4 μg siRNA, respectively, using nucleofection (Amaxa Biosystems) according to manufacturer's instructions. For detection of tyrosine phosphorylation, single nucleofection reactions of bEnd.5 cells and HUVECs were scaled up five- and threefold, respectively.
Permeability assay.
To determine paracellular permeability, 1.25 × 105 endothelioma cells were seeded per 6.5-mm-diameter Transwell filters (Costar 3413; Corning) with a 0.4-μm pore size, coated with 50 μg/ml laminin. After nucleofection, bEnd.5 cells were cultured for 24 h before the assay. Permeability was analyzed with 0.25 mg/ml FITC-dextran (250 kD; Sigma Aldrich), and diffusion was allowed for 30 min at 37°C and 10% CO2. Fluorescence in the lower chamber was measured with a fluorimeter (Fluoromax-2; Jobin-Yvon). Confluence of cells was determined by immunofluorescence staining for ESAM after each assay.
Adhesion assay.
For adhesion of bEnd.5, plakoglobin−/−, and β-catenin−/− cells to immobilized VE-cadherin–Fc, 96-well plates were coated with 20 μg/ml of mouse VE-cadherin–Fc (21) in PBS containing 1 mM CaCl2 and 1 mM MgCl2 (PBS-MC) overnight at 4°C, followed by blocking with 3% BSA in PBS-MC for 1 h at room temperature. For negative controls, wells were coated with E-selectin–Fc. Before the assay, cells were stained with 10 μg/ml Hoechst dye in adhesion medium (DMEM containing 1 mM MgCl2, 1 mM CaCl2, and 25 mM Hepes [pH 7.3]) for 30 min at 37°C and harvested with 0.05 mg/ml trypsin without EDTA for 15–20 min, and trypsin was removed and residual trypsin was inhibited with 1 mg/ml of soybean trypsin inhibitor. 105 cells in 100 μl of adhesion medium were seeded per well and incubated for 30–60 min at 37°C. After washing with PBS-MC until background binding to E-selectin–Fc was minimal, adhering cells were fixed for 20 min with cold methanol (−20°C), rehydrated in PBS-MC, and counted by fluorescence microscopy.
Transmigration assay.
Transendothelial migration of PMNs across bEnd.5 monolayers was performed essentially as previously described (14), except that 106 PMNs were added per Transwell filter, TNF-α activation was omitted, and transmigration was allowed for 15 min. Transmigration across HUVEC monolayers was analyzed by adding 4 × 105 human neutrophils for 20 min to TNF-α–activated HUVECs grown on gelatin-coated coverslips, followed by fixation with 2% glutaraldehyde. Transmigrated cells were identified and counted by phase-contrast microscopy.
Adhesion of leukocytes to endothelium.
Highly confluent bEnd.5 cells in a 90-mm dish either stimulated for 6 h with 5 nM TNF-α or left unstimulated were incubated for 5 min with either 3 × 107 PMNs (freshly isolated from mouse bone marrow) or the same number of antigen-stimulated T cells (both previously described in reference 14) in 2.5 ml of growth medium at 37°C and 10% CO2. Adhering cells were removed by washing three to five times with prewarmed PBS. Of note, in contrast to human leukocytes added to HUVECs, mouse leukocytes added to mouse endothelioma could be removed quantitatively with PBS. In some experiments, lymphocytes were incubated for 30 min and then removed. Subsequently, endothelial cells were lysed and analyzed by immunoprecipitation.
Aggregation assays.
Aggregation assays with transfected CHO cells were essentially performed as previously described (13, 14), except that cells were harvested with 0.05% trypsin in HBSS supplemented with 25 mM Hepes, pH 7.3, (HHBSS) in the presence of 5 mM CaCl2 and 2 mM MgCl2. Trypsin was stopped with DMEM containing 10% FCS and 0.05% soybean trypsin inhibitor. Cells were pelleted and resuspended at 7.5 × 105 cells/ml in HHBSS without Ca2+ and Mg2+. For aggregation, 1 ml of this cell supension was added to 1 ml of HHBSS supplemented with 10 mM Ca2+, 2 mM Mg2+, and 100 U DNase.
Statistical analysis.
Statistical significance was analyzed using the Student's t test for independent samples. p-values are indicated by asterisks: *, P < 0.05; **, P < 0.01; and ***, P < 0.001. Results are shown as means ± SEM.
Online supplemental material.
Fig. S1 shows that distribution and expression of various endothelial cell contact proteins are unaffected by VE-PTP or hPTPβ silencing (24 h after transfection) in mouse endothelioma or HUVECs, respectively. Online supplemental material is available at http://www.jem.org/cgi/content/full/jem.20080406/DC1.
Acknowledgments
We thank Dr. Volker Gerke for valuable discussions, and Monika Rieschel and Stefanie Merfeld for technical help in establishing endothelioma cell lines.
This project was funded by the Max-Planck-Gesellschaft and by grants from the Deutsche Forschungsgemeinschaft to B. Engelhardt (SFB 629, A9) and to D. Vestweber (SFB629, A1).
The authors have no conflicting financial interests.
Notes
Abbreviations used: CHO, Chinese hamster ovary; ESAM, endothelial cell–selective adhesion molecule; GST, glutathione S-transferase; HUVEC, human umbilical vein endothelial cell; ICAM, intercellular adhesion molecule; JAM, junctional adhesion molecule; PECAM-1, platelet endothelial cell adhesion molecule; siRNA, small interfering RNA; VE, vascular endothelial; VEGF, vascular endothelial growth factor; VE-PTP, vascular endothelial protein tyrosine phosphatase.
References
Articles from The Journal of Experimental Medicine are provided here courtesy of The Rockefeller University Press
Full text links
Read article at publisher's site: https://doi.org/10.1084/jem.20080406
Read article for free, from open access legal sources, via Unpaywall:
https://rupress.org/jem/article-pdf/205/12/2929/1195171/jem_20080406.pdf
Free after 6 months at intl.jem.org
http://intl.jem.org/cgi/reprint/205/12/2929.pdf
Free to read at intl.jem.org
http://intl.jem.org/cgi/content/abstract/205/12/2929
Free after 6 months at intl.jem.org
http://intl.jem.org/cgi/content/full/205/12/2929
Citations & impact
Impact metrics
Citations of article over time
Alternative metrics
Smart citations by scite.ai
Explore citation contexts and check if this article has been
supported or disputed.
https://scite.ai/reports/10.1084/jem.20080406
Article citations
Ubiquitination of VE-cadherin regulates inflammation-induced vascular permeability in vivo.
EMBO Rep, 25(9):4013-4032, 07 Aug 2024
Cited by: 0 articles | PMID: 39112792 | PMCID: PMC11387630
Molecular Mechanisms Regulating Vascular Endothelial Permeability.
Int J Mol Sci, 25(12):6415, 11 Jun 2024
Cited by: 0 articles | PMID: 38928121
Review
Beyond VEGF: Angiopoietin-Tie Signaling Pathway in Diabetic Retinopathy.
J Clin Med, 13(10):2778, 09 May 2024
Cited by: 0 articles | PMID: 38792322 | PMCID: PMC11122151
Review Free full text in Europe PMC
Heterogeneity of endothelial VE-PTP downstream polarization, Tie2 activation, junctional claudin-5, and permeability in the aorta and vena cava.
Cell Tissue Res, 395(1):81-103, 30 Nov 2023
Cited by: 1 article | PMID: 38032480 | PMCID: PMC10774230
Spleen Tyrosine Kinase phosphorylates VE-cadherin to cause endothelial barrier disruption in acute lung injury.
J Biol Chem, 299(12):105408, 28 Oct 2023
Cited by: 1 article | PMID: 38229397 | PMCID: PMC10731244
Go to all (153) article citations
Data
Data behind the article
This data has been text mined from the article, or deposited into data resources.
BioStudies: supplemental material and supporting data
Similar Articles
To arrive at the top five similar articles we use a word-weighted algorithm to compare words from the Title and Abstract of each citation.
Plakoglobin maintains the integrity of vascular endothelial cell junctions and regulates VEGF-induced phosphorylation of VE-cadherin.
J Biochem, 162(1):55-62, 01 Jul 2017
Cited by: 11 articles | PMID: 28158602
Dissociation of VE-PTP from VE-cadherin is required for leukocyte extravasation and for VEGF-induced vascular permeability in vivo.
J Exp Med, 208(12):2393-2401, 24 Oct 2011
Cited by: 131 articles | PMID: 22025303 | PMCID: PMC3256962
How T cells trigger the dissociation of the endothelial receptor phosphatase VE-PTP from VE-cadherin.
Blood, 122(14):2512-2522, 01 Aug 2013
Cited by: 55 articles | PMID: 23908467
Endothelial permeability and VE-cadherin: a wacky comradeship.
Cell Adh Migr, 7(6):455-461, 01 Nov 2013
Cited by: 43 articles | PMID: 24430214 | PMCID: PMC3916348
Review Free full text in Europe PMC