Abstract
Free full text

Distinct Roles for Mammalian Target of Rapamycin Complexes in the Fibroblast Response to Transforming Growth Factor-beta
Abstract
Transforming growth factor-beta (TGF-β) promotes a multitude of diverse biological processes including growth arrest of epithelial cells and proliferation of fibroblasts. While the TGF-β signaling pathways that promote inhibition of epithelial cell growth are well characterized, less is known regarding the mechanisms mediating the positive response to this growth factor. Given that TGF-β has been demonstrated to promote fibrotic diseases and desmoplasia, identifying the fibroblast-specific TGF-β signaling pathways is critical. Here we investigate the role of mammalian target of rapamycin (mTOR), a known effector of PI3K and promoter of cell growth, in the fibroblast response to TGF-β. We show that TGF-β activates mTORC1 in fibroblasts but not epithelial cells via a PI3K-Akt-TSC2 dependent pathway. Rapamycin, the pharmacological inhibitor of mTOR, prevents TGF-β mediated anchorage-independent growth without affecting TGF-β transcriptional responses or extracellular matrix protein induction. In addition to mTORC1, we also examined the role of mTORC2 in TGF-β action. mTORC2 promotes TGF-β induced morphological transformation and is required for TGF-β induced Akt S473 phosphorylation, but not mTORC1 activation. Interestingly, both mTOR complexes are necessary for TGF-β mediated growth in soft agar. These results define distinct and over-lapping roles for mTORC1 and mTORC2 in the fibroblast response to TGF-β and suggest that inhibitors of mTOR signaling may be useful in treating fibrotic processes such as desmoplasia.
INTRODUCTION
Transforming growth factor-beta (TGF-β) is a versatile cytokine that regulates a variety of biological processes including tissue growth, differentiation, cell migration, angiogenesis, immunity, and ECM (extracellular matrix) production among others (1). One of the most intriguing aspects of TGF-β biology is the diversity of cellular responses that can be induced depending on the cell type and stimulation context (2). For instance, TGF-β has been demonstrated to suppress tumor formation while also promoting wound healing via fibroblast proliferation and differentiation into myofibroblasts, spindle-shaped cells that are professional secretors of ECM proteins (3-6). Though the TGF-β signaling pathways that mediate epithelial cell growth arrest are well characterized, less is known regarding the mechanisms mediating the positive fibroblast response.
While the physiological role of TGF-β mediated production of myofibroblasts is to promote wound healing, under certain circumstances, this program can become dysfunctional and lead to fibrotic pathologies (7, 8). For instance, carcinomas originating in various organs are well characterized to be associated with a growth promoting fibrotic (desmoplastic) reaction (9-12). Not surprising, TGF-β has been shown to mediate fibrotic processes such as desmoplasia in various contexts (11, 13-15). Interestingly, carcinoma associated fibroblasts exhibit similar in vitro characteristics as normal fibroblasts except they express higher levels of TGF-β and possess a significantly increased ability to grow in soft agar (16). Given the known role of TGF-β in promoting or exacerbating fibrotic pathologies, it is necessary to further elucidate the mechanisms whereby this cytokine promotes fibroblast activation.
TGF-β initiates signal transduction by utilizing two receptor serine/threonine kinases referred to as the type I (ALK5) and type II (TβR-II) receptors. TGF-β binding mediates the formation of a heterotetrameric receptor complex whereby the constitutively active TβR-II phosphorylates the glycine-serine rich region in the juxtamembrane region of the dormant ALK5 leading to kinase activation (17). Activated ALK5 directly phosphorylates the receptor-regulated Smad proteins (R-Smads) on a C-terminal SM/VS motif (18). In most cell types, TGF-β treatment leads to phosphorylation of Smad2 and Smad3, which subsequently complex with the Co-Smad (Smad4) and accumulate in the nucleus where they recognize Smad binding elements (SBE: AGAC) and collaborate with other transcription factors to regulate gene expression (19).
While it is clear that Smad proteins are critical TGF-β effectors, distinct cellular phenotypes result even though the same Smad proteins (Smad2 and Smad3) are activated. One potential explanation for the variability in the cellular response to TGF-β is the existence of cell type-specific signaling pathways. Consistent with the ability of TGF-β to induce fibroblast proliferation, a number of mitogenic targets including PAK2, Ras, PI3K, and c-Abl have been identified which are activated by TGF-β in a subset of fibroblast, but not epithelial lines (3, 5, 6, 20).
In addition, TGF-β has been shown to activate the serine/threonine kinase Akt downstrem of PI3K (3). However, the Akt effectors that promote fibroblast activation in the context of TGF-β signaling remain unclear. The current model of Akt activation proposes that the generation of phosphatidylinositol 3,4,5-trisphosphate by PI3K mediates membrane recruitment of Akt via its pleckstrin homology domain. Akt is then regulated by two phosphorylation events which include the modification of T308 within the T loop of its catalytic domain by PDK1, and also S473 within its C-terminal hydrophobic motif (HM) by PDK2 (21).
Despite the large number of Akt effectors, evidence from Drosophila and murine studies suggest that the pro-growth signals mediated by Akt are primarily via activation of mTORC1 (mammalian target of rapamycin complex 1) (22, 23). mTOR is a serine/threonine kinase that exists in two complexes referred to as mTOR complex 1 (mTORC1: mTOR, RAPTOR, mLST8, PRAS40) and complex 2 (mTORC2: mTOR, RICTOR, mLST8, mSIN1, PROTOR) (24). mTORC1, a known promoter of cell growth, is controlled by a wide variety of factors including receptor tyrosine kinases, nutrients, and cellular energy status (25). mTORC1 activity is induced by the small G protein Rheb which is negatively regulated by two tumor suppressors, TSC1 (Hamartin) and TSC2 (Tuberin) encoded by the tuberous sclerosis complex 1 and 2 genes (25). TSC1 and TSC2 form a complex in which the GAP (GTPase activating protein) domain of TSC2 promotes hydrolysis of Rheb-GTP to Rheb-GDP, thereby inhibiting mTORC1 (26). Receptor tyrosine kinases have been shown to promote the accumulation of GTP-bound Rheb via inhibition of the TSC1/TSC2 complex by inducing the phosphorylation of TSC2 (24). Akt has been well documented to be one of the kinases capable of directly phosphorylating and inactivating TSC2 (27-29). Once activated, mTORC1 phosphorylates a number of effectors including S6 kinase 1 (S6K1) and eukaryotic initiation factor 4E-binding protein 1 (4E-BP1) to promote translation initiation (25).
In contrast to mTORC1, the regulation and effectors of mTORC2 are less well understood. Recently, mTORC2 has been demonstrated to be the elusive PDK2 responsible for phosphorylating Akt on S473 (30-32). Modification of Akt by mTORC2 is not necessary for kinase activation, but is required for phosphorylation of certain substrates such as FoxO transcription factors (31, 32). In addition to Akt, mTORC2 is required for phosphorylation of PKCα on Ser657 within its HM, a modification that promotes PKCα stability (31-33). Finally, mTORC2 has been implicated in regulating cytoskeletal dynamics via the activation of Rho GTPases (33-35). Therefore, mTOR exists in two complexes that exhibit functions associated with Akt signaling and are demonstrated to promote cell growth and cell shape changes.
Here we investigate the role of mTOR signaling in the fibroblast response to TGF-β and show that (i) TGF-β activates mTORC1 in fibroblasts but not epithelial cells; (ii) mTORC1 activation occurs via a canonical PI3K-Akt-TSC2 dependent pathway; (iii) rapamycin inhibits TGF-β mediated anchorage-independent growth of fibroblasts without affecting TGF-β transcriptional responses or ECM protein induction; (iv) mTORC2 is required for TGF-β induced Akt S473 phosphorylation but not mTORC1 signaling; (v) mTORC2 is uniquely required for TGF-β mediated fibroblast morphological transformation; and (vi) both mTORC1 and mTORC2 are required for TGF-β mediated colony formation in soft agar. These results define distinct as well as over-lapping roles for mTORC1 and mTORC2 in the fibroblast response to TGF-β and suggest that inhibitors of mTOR signaling may be useful in treating fibrotic processes such as desmoplasia.
MATERIALS AND METHODS
Cell Culture
Cells were grown in high glucose DMEM (Invitrogen, Life Technologies, Inc., Gaithersburg, MD) supplemented with 10% fetal bovine serum (FBS; Hyclone, Logan, UT). For signaling experiments, cells were seeded at 2.5 × 106 in 100 mm tissue culture dishes, grown to confluence, and subsequently serum-starved by replacing media with either 0.1% FBS/DMEM or serum-free DMEM for 24 ours. TSC2 -/- MEFs were obtained from Dr. David Kwiatkowski (Harvard Medical School, Cambridge, MA). mLST8 +/- and mLST8 -/- MEFs were obtained from Dr. David Sabatini (Whitehead Institute, MIT, Cambridge, MA). All other cell lines were purchased from ATCC (Rockville, MD). Human TGF-β was obtained from R&D Systems (Minneapolis, MN).
Pharmacological inhibitors
Pharmacological agents LY294002 (PI3K inhibitor) and U0126 (MEK inhibitor) were purchased from Calbiochem (San Diego, CA). Rapamycin (mTOR inhibitor) was purchased from LC Laboratories (Woburn, MA).
Antibodies
Anti-phospho-S6K1 T389, anti-phospho-ERK, anti-phospho-Akt S473, anti-phospho-TSC2 S939, anti-phospho-TSC2 T1462, anti-TSC2, anti-Raptor, anti-Rictor, and anti-mTOR antibodies were purchased from Cell Signaling Technology (Beverly, MA). Anti-phospho-Smad2 was purchased from Calbiochem (San Diego, CA). Anti-Smad2 and Anti-Smad3 antibodies were purchased from Zymed Laboratories (Carlsbad, CA) while anti-HA 12CA5 was obtained from Sigma-Aldrich (St. Louis, MO). Anti-ERK, anti-fibronectin, anti-collagen1A1, donkey anti-rabbit HRP, and goat anti-mouse HRP antibodies were purchased from Santa Cruz Biotechnology (Santa Cruz, CA). The anti-phospho-Smad3 antibody to the peptide COOH-GSPSIRCSpSVpS was generated in our laboratory (5).
Western blotting
Cells were lysed with 50 mM Tris-HCl (pH 7.4), 1% TritonX-100, 0.25% Na-deoxycholate, 150 mM NaCl, 1 mM EDTA, 1 mM PMSF, 1 mM Na3VO4, 5 mM NaF, and 1x Complete protease inhibitor (Roche Applied Science, Indianapolis, IN). Equivalent total protein was separated by SDS-PAGE. Protein was transferred to either PVDF (Millipore; Billerica, MA) or nitrocellulose (Bio-Rad; Hercules, CA). Membranes were probed with indicated antibodies following the manufactures protocol.
Immunoprecipitations
Transfected TSC2 -/- MEFs were lysed as described above. Approximately 500 μg of lysate was incubated with 4 μg of anti-HA 12CA5 overnight at 4°C. Immune complexes were collected by addition of 50 μL protein G sepharose (Upstate Biotechnology; Charlottesville, MA) for two hours. Sepharose beads were washed four times with lysis buffer and subsequently suspended in 50 μL 2x Laemmli buffer.
Morphological Transformation
AKR-2B cells were seeded at 2.5 × 106 in 6 well tissue culture dishes, grown to confluence, and subsequently serum-starved by replacing media with serum free DMEM for 24 hours. The cells were then pretreated for 30 minutes with either EtOH (vehicle) or 10 nM rapamycin and left untreated or stimulated with 5 ng/ml TGF-β for 48 hours.
Soft Agar Assay
To prevent cells from settling on the plate bottom and adhering, 1 ml bottom plugs containing 0.8% Sea Plaque-agarose (FMC Bioproducts, Rockland, ME), 10% FBS/DMEM were cast in 35 mm plates. 1 ml top plugs were composed of 0.4% agarose, 10% FBS/DMEM, 104 AKR-2B cells in the presence or absence of 5 ng/ml TGF-β. As indicated, top plugs contained vehicle or the pharmacological inhibitor rapamycin. After 10 days at 37°C, the number of colonies greater than 25 μm in diameter were counted by microscopy using a 1.0-cm grid. Ten grid regions were counted on each of 3 plates. Quantization represents the average and standard deviation of three independent experiments each done in triplicate.
Transfections
All transfections were performed in 10% FBS/DMEM using Lipofectamine 2000 transfection reagent (Roche Diagnostics, Indianapolis, IN). For transfection of TSC2 -/- MEFs, cells were plated at 2 × 106 cells per 100 mm tissue culture plates. The following day, cells were transfected with 5 μg HA-S6K1 and either 5 μg FLAG-TSC2 WT or 5 μg FLAG-TSC2 SATA. After 4 hours, the media was changed to 10% FBS/DMEM and cells were allowed to recover for 12 hours. Constructs and conditions for the transfection of AKR-2B and 293FT cells are described below.
Luciferase Assays
AKR-2B cells were plated in six-well plates at 2 × 105 per well. The next day, cells were transfected with 0.5 μg of CMV-β-galactosidase and either SBE-Luc (3.5 μg), ARE-Luc (2 μg) + FAST-1 (2 μg), Fibronectin promoter-Luc (3.5 μg), or Type I collagen promoter-Luc (3.5 μg). After 4 hours, media were changed to DMEM-5% FBS, and the cells allowed to recover for 12 hours. Cells were subsequently serum-starved in 0.1% FBS/DMEM for 24 hours. Prior to stimulation, cells were pretreated for 30 minutes with either EtOH or 10 nM rapamycin and then treated ± 5 ng/ml TGF-β1 for 24 hours.
Lentiviruses
pLKO.1-puro plasmids encoding shRNAs targeting raptor, rictor, and mTOR were obtained from the Mayo Clinic Jacksonville RNA interference Technology Resource. Lentivirus packaging was performed using the ViraPower Lentiviral Expression System (Invitrogen, Life Technologies, Inc., Gaithersburg, MD). 293FT cells were co-transfected with pLKO.1-puro shRNA and ViraPower DNA mix using Lipofectamine 2000 transfection reagent. 12 hours post-transfection media was changed to 10% FBS/DMEM. Supernatants were collected 48-72 hours post-transfection. AKR-2B fibroblasts were transduced in the presence of 6 μg/ml polybrene (Sigma-Aldrich). Stable cell clones were selected and isolated in 1.5 μg/ml puromycin.
RESULTS
TGF-β activates mTORC1 in fibroblasts but not epithelial cells
In order to determine whether TGF-β activates mTORC1 in fibroblasts, AKR-2B cells were stimulated with TGF-β and the appearance of S6K1 phosphorylated on T389, a known mTORC1 site, was monitored. Phosphorylated S6K1 was observed after 2 hours of treatment and remained detectable through 12 hours (Fig. 1A, left panel). This increase in S6K1 T389 phosphorylation occurred in conjunction with a reduction in the electrophoretic mobility of S6K1 (Fig. 1A, left panel). In addition, TGF-β stimulation induced the phosphorylation of Smad2 within 30 minutes (Fig. 1A, left panel). In contrast, Mv1Lu epithelial cells did not induce phosphorylation of S6K1 nor alter its electrophoretic mobility, though phosphorylated Smad2 was readily detected (Fig. 1A, right panel). In order to determine whether phosphorylation of S6K1 represents a cell type-specific response to TGF-β, three representative fibroblast cell lines (AKR-2B, Swiss3T3, IMR-90) and three epithelial cell lines (Mv1Lu, Hela, MDCK) were stimulated with TGF-β and the phosphorylation of S6K1 examined. As shown in Fig. 1B, though the degree of signal induction varied, all three fibroblast cell lines exhibited robust phosophorylation of S6K1 in response to TGF-β whereas no detectable signal was observed from any of the epithelial cells.

A, AKR-2B fibroblasts (left panel) or Mv1Lu epithelial cells (right panel) were serum-starved overnight and stimulated with 5 ng/ml TGF-β. At the indicated times, Western analysis was performed using antibodies to phospho (pS6K1 T389 and pSmad2) or total S6K1 and Smad2. B, Three fibroblast (AKR-2B, Swiss3T3, and IMR-90) and three epithelial (Mv1Lu, MDCK, and Hela) cell lines were treated as above and stimulated with TGF-β for 4 hours. Western blot analysis was performed as in panel A.
TGF-β activates mTORC1 via a PI3K-Akt-TSC2 dependent pathway
The current model of receptor tyrosine kinase mediated inhibition of TSC1/TSC2 involves inducing the phosphorylation of TSC2 via either Akt or ERK-RSK (24). Given that TGF-β has been shown to activate both PI3K-Akt and Ras-ERK activity in fibroblasts (3, 20), we investigated whether either pathway(s) might be necessary for TGF-β mediated mTORC1 signaling. In order to address this issue, serum-starved AKR-2B fibroblasts were pretreated with various pharmacological inhibitors and subsequently treated with TGF-β. As shown in Fig. 2A, the PI3K inhibitor LY294002 abolished the ability of TGF-β to induce phosphorylation of S6K1 to a similar degree as rapamycin. However, the MEK inhibitor U0126 had no effect despite completely preventing ERK phosphorylation.

A, AKR-2B cells were serum-starved overnight followed by pretreatment with vehicle (0.1% DMSO), LY294002 (10 μM), UO126 (10 μM), or Rapamycin (10 nM) for 30 minutes. Cells were left untreated (-) or stimulated (+) with TGF-β (5 ng/ml) for 4 hours in the presence of the indicated inhibitors. Western blot analysis was performed as described in Materials and Methods. B, AKR-2B fibroblasts were prepared as in panel A and stimulated with 5 ng/ml TGF-β for the indicated times. Western analysis was performed using antibodies to phospho (pTSC2 S939 and pAkt S473) or total TSC2 and Akt. C, TSC2 -/- MEFs were transfected with HA-S6K1 and TSC2 wild type (WT) or TSC2 SATA. Cells were serum-starved overnight and subsequently stimulated with 5 ng/ml TGF-β for 4 hours. HA-S6K1 was immunoprecipitated and assayed for phosphorylation (pS6K1 T389) or total expression (Anti-HA). Western blot analysis was performed on total cell lysate for FLAG-TSC2, phospho-Smad2 (pSmad2), and total Smad2. D, AKR-2B cells were serum-starved overnight followed by pretreatment with vehicle (H2O) or CHX (cycloheximide, 1.25 ug/ml) for 30 minutes. Cells were subsequently stimulated with TGF-β for 6 hours. Western blot analysis performed as described in Materials and Methods.
Akt promotes mTORC1 activation via phosphorylation of TSC2 (28, 36). Given the previous pharmacologic data indicating PI3K-Akt signaling as the primary mediator of TGF-β dependent S6K1 phosphorylation (Fig. 2A), we investigated whether TGF-β induces phosphorylation of TSC2. As shown in Fig. 2B, TGF-β promotes Akt and TSC2 modification with similar kinetics. Although Figs. 2A and 2B clearly implicate Akt in TGF-β stimulated mTORC1 activity, to conclusively determine if Akt mediated phosphorylation of TSC2 is necessary for TGF-β mediated mTORC1 activation a genetic approach was utilized. While multiple Akt phosphorylation sites exist on TSC2, S939 and T1462 are the predominantly modified sites and are necessary for Akt mediated inhibition of TSC2 (28). Therefore, we transfected TSC2 -/- MEFs with constructs encoding HA-S6K1 and either wild-type TSC2 or TSC2 possessing alanines at Ser939 and Thr1462 (SATA). TSC2 -/- MEFs transfected with wild-type TSC2 exhibited TGF-β mediated phosphorylation of HA-S6K1 whereas cells transfected with the TSC2 SATA mutant failed to induce HA-S6K1 phosphorylation (i.e., TGF-β signaling could not inactivate the SATA mutant), despite displaying normal Smad2 phosphorylation (Fig. 2C). The results are consistent with the model whereby TGF-β activates mTORC1 via the canonical PI3K-Akt-TSC2 dependent pathway.
Interestingly, the kinetics of TGF-β mediated PI3K-Akt-mTORC1 signaling is delayed compared to receptor tyrosine kinases, which active this pathway within minutes of ligand treatment. While we have observed a weak early activation of PI3K after TGF-β treatment that is independent of new protein synthesis (3) (data not shown), in order to investigate whether synthesis of an intermediate factor(s) is required for this late signaling event we stimulated serum-starved AKR-2B cells with TGF-β in the absence or presence of the protein synthesis inhibitor cycloheximide. As shown in Fig. 2D, Akt phosphorylation upon 6 hours TGF-β treatment is completely inhibited by cycloheximide (Fig. 2D). Unfortunately, we were unable to examine the activation of mTORC1 in this experiment since both transcriptional and translational inhibitors alone promote S6K1 phosphorylation (37, 38) (data not shown).
Rapamycin inhibits TGF-β mediated anchorage-independent growth of AKR-2B cells
We next investigated whether mTOR plays a role in the fibroblast biological response to TGF-β. Several fibroblast cell lines have been documented to morphologically transform into a myofibroblast phenotype and undergo anchorage-independent growth (AIG) following TGF-β treatment (3-5, 39). In order to determine whether these responses are dependent upon mTOR, we utilized the pharmalogical agent rapamycin, a potent inhibitor of mTORC1 that has also been reported to attenuate mTORC2 with prolonged treatment, up to 24 hours (40). As shown in Fig. 3A, rapamycin only modestly lessened TGF-β mediated AKR-2B morphological transformation. However, rapamycin completely prevented TGF-β stimulated AIG with half maximal inhibition occurring at sub nM concentrations (Figs. 3B and 3C).
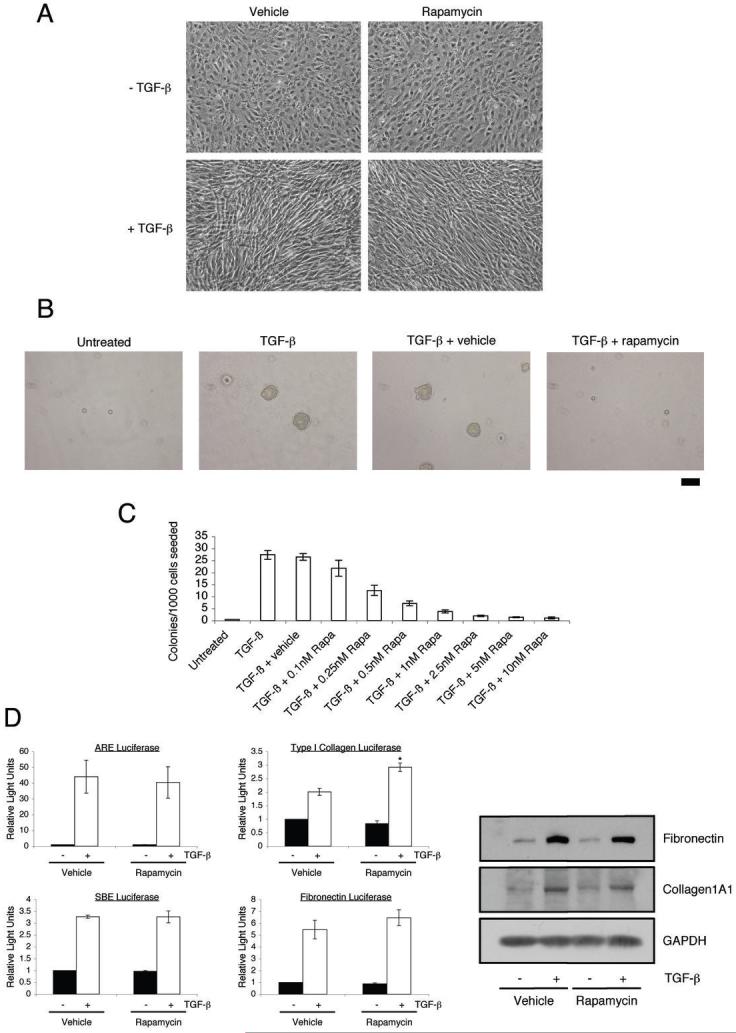
A, AKR-2B fibroblasts were serum-starved overnight and subsequently pretreated with either vehicle (0.1% EtOH) or 10 nM rapamycin for 30 minutes. Cells were then left untreated (-) or stimulated (+) with 5 ng/ml TGF-β for 48 hours. Photographs of representative fields are shown. B, AKR-2B cells were seeded in soft agar with the indicated treatments as described in Materials and Methods. Photographs of representative fields are shown following 10 days growth. Trypan blue exclusion showed that rapamycin did not increase cell death over the course of seven days (data not shown). Scale bar (far right) represents 100 μm. C, Quantitation of AKR-2B soft agar experiments performed with indicated treatments. Data represent the mean ± standard error of 3 independent experiments each done in triplicate. D, AKR-2B cells were transfected with ARE, SBE, Type I collagen, or Fibronectin responsive luciferase reporter constructs, serum-starved overnight, and subsequently pretreated with either vehicle (0.1% EtOH) or 10 nM rapamycin for 30 minutes. Cells were then left untreated (black) or stimulated (white) with 5 ng/ml TGF-β for 24 hours. Relative lights units are presented with all values relative to unstimulated control cells. Mean ± standard error of 3 independent experiments performed in triplicate are shown. Asterisk represents statistical significance (p<0.05) compared to control (vehicle and TGF-β treated) using an independent two sample t-test. D (right panel), AKR-2B fibroblasts were serum-starved overnight and subsequently pretreated with either vehicle (0.1% EtOH) or 10 nM rapamycin for 30 minutes. Cells were then left untreated (-) or stimulated (+) with 5 ng/ml TGF-β for 24 hours. Western analysis was performed to determine the protein expression of fibronectin and Collagen1A1. GAPDH was used as a loading control.
To further assess the role of mTOR in TGF-β signaling, the effect of rapamycin on the induction of various TGF-β responsive promoters was determined. Rapamycin did not inhibit the transcriptional induction of ARE (Smad2-dependent), SBE (Smad-3 dependent), Fibronectin, or Type I collagen (Fig. 3D). Furthermore, consistent with the transient reporter analyses, there was no detectable impact of rapamycin on TGF-β stimulated fibronectin or Type I collagen protein expression (Fig. 3D, right panel). These findings indicate that while mTORC1 is critical for TGF-β AIG, it is not a general regulator of TGF-β transcriptional or translational responses.
mTORC2 is required for TGF-β mediated Akt S473 phosphorylation but not mTORC1 signaling
Though initial studies suggested that mTORC1 is rapamycin sensitive while mTORC2 is resistant to this pharmacological agent, recent evidence indicates that prolonged rapamycin treatment (24 hours) can also inhibit mTORC2 (40). Given that our soft agar assay is performed over a 10 day period, this would preclude determining whether rapamycin blocked cell growth due to inhibition of mTORC1, mTORC2, or both. As such, to investigate the potential role of mTORC2 in TGF-β action, we first investigated whether mTORC2 has a similar role in TGF-β signaling as reported for receptor tyrosine kinases.
Previous reports have demonstrated that mTORC2 is required for phosphorylation of Akt on S473 within its C-terminus, but is not required for Akt T308 phosphorylation (30-32). Of note, while Akt S473 phosphorylation appears to be required for a subset of Akt substrates, many (including TSC2) can still be phosphorylated in the absence of S473 phosphorylation (31, 32). To address the role of mTORC2 in the context of pro-fibrotic TGF-β signaling, we utilized MEFs deficient in mLST8, a component of both mTOR complexes which is required for mTORC2 function, but not mTORC1 (32). As shown in Fig. 4A and consistent with that observed for receptor tyrosine kinases, while mLST8 -/- MEFs fail to induce phosphorylation of Akt S473 in response to TGF-β, Akt T308 phosphorylation as well as TSC2 and S6K1 signaling remain intact.
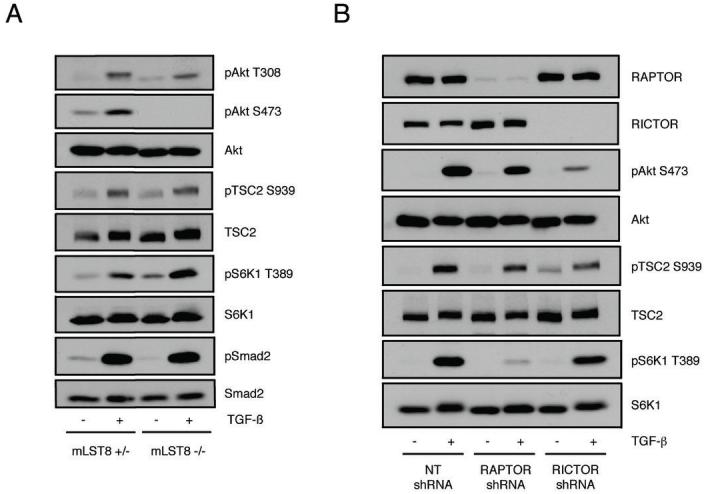
A, mLST8 +/- and mLST8 -/- MEFs were serum-starved overnight and either left untreated (-) or stimulated (+) with 5 ng/ml TGF-β for 4 hours. Western blots were performed with indicated phospho and corresponding total antibodies. B, Stable AKR-2B cell lines expressing either Non-targeting (NT), RAPTOR targeting, or RICTOR targeting shRNA were serum-starved overnight and either left untreated (-) or stimulated (+) with 5 ng/ml TGF-β for 6 hours and processed for Western analysis with indicated antibodies.
In order to further delineate the roles of mTORC1 and mTORC2 in the fibroblast response to TGF-β, we created stable AKR-2B cell lines expressing shRNAs targeting RAPTOR and RICTOR. We were unable to isolate a stable cell clone with efficient knockdown of mTOR, suggesting that long-term reduction in mTOR expression is incompatible with AKR-2B cell viability. In Fig. 4B, it is shown that knockdown of RAPTOR inhibits TGF-β mediated phosphorylation of S6K1 without affecting phosphorylation of Akt S473 or TSC2. In agreement with the results using the mLST8 null MEFs (Fig. 4A), RICTOR knockdown diminishes Akt Ser473 phosphorylation without significantly affecting phosphorylation of TSC2 or S6K1 (Fig. 4B).
mTORC1 and mTORC2 provide distinct and over-lapping actions in the fibroblast response to TGF-β
Given that mTORC2 has been implicated in cytoskeletal dynamics (33, 34), and TGF-β morphologic transformation is associated with changes in cytoarchitecture (4), we further investigated the role of mTORC2 in TGF-β mediated fibroblast morphologic transformation. As shown in Fig. 5A and consistent with the results of Fig. 3A using rapamycin, expression of control or RAPTOR-targeting shRNA in AKR-2B fibroblasts has no affect on the morphological changes induced by TGF-β. However, fibroblasts expressing a RICTOR-targeting shRNA exhibit a significant attenuation in TGF-β mediated formation of spindle-shaped cells (Fig. 5A). Thus, mTORC2 might be involved in TGF-β mediated morphological changes that are insensitive to rapamycin.

A, The effect of Non-targeting (NT), RAPTOR targeting, or RICTOR targeting shRNA on AKR-2B morphologic transformation was determined as discussed in Fig. 3A and Materials and Methods. Photographs of representative fields are shown. B, AKR-2B cells were serum-starved for 24 hours in the presence of 0.1% EtOH or 10 nM rapamycin. Cells were then left untreated or stimulated with TGF-β (5 ng/ml) for 4 hours. Western analysis was performed using antibodies to phospho (pAkt S473 and pS6K1 T389) or total Akt and S6K1. C, Three fibroblast (AKR-2B, Swiss3T3, and IMR-90) cell lines were grown in DMEM supplemented with 10% serum in the presence of 0.1% EtOH (-) or 10 nM rapamycin (+) for 24 hours. Western analysis was performed with indicated antibodies on proliferating cultures.
The finding that rapamycin does not affect TGF-β mediated morphological transformation (Fig. 3A) whereas RICTOR knockdown attenuates this process (Fig. 5A) suggests that mTORC2 is not significantly inhibited by rapamycin in AKR-2B cells. To investigate the sensitivity of mTORC2 in AKR-2B cells to rapamycin, we treated serum-starved AKR-2B cells with vehicle or rapamycin for 24 hours prior to TGF-β stimulation. As shown in Fig. 5B, prolonged rapamycin treatment did not attenuate TGF-β mediated Akt S473 phosphorylation though it completely inhibited S6K1 T389 phosphorylation. Although this might appear to differ from the study by Sarbassov et al., those investigators also reported that the sensitivity of mTORC2 to prolonged (24 hours) rapamycin treatment varied considerably among different cell lines with some exhibiting nearly complete loss of Akt S473 phosphorylation in the presence of 10% serum while others showed no attenuation (40). As such, in order to further define the sensitivity of mTORC2 in fibroblasts, AKR-2B, Swiss3T3, and IMR-90 fibroblasts were treated with either EtOH or rapamycin in the presence of 10% serum for 24 hours. Fig. 5C demonstrates that while rapamycin completely abrogates S6K1 phosphorylation, it has no affect on the phosphorylation of Akt Ser473. These results indicate that mTORC2 expressed in a subset of human and murine fibroblast lines is rapamycin-insensitive, as has been described for other cell types (40).
Next, we investigated the role of both mTOR complexes in TGF-β mediated AIG. Given that cells can exhibit variability in the extent of growth in soft agar, we performed transient transduction with lentiviruses expressing shRNA molecules to avoid differences in growth due to clonal selection. Fig. 6A demonstrates shRNA expressing lentiviruses were effective at reducing the expression of RAPTOR, RICTOR, and mTOR without influencing the expression of other mTOR complex components. These AKR-2B cultures were then used to determine the ability of TGF-β to induce soft agar colony formation. Interestingly, knockdown of either RAPTOR, RICTOR, or mTOR significantly inhibited the ability of TGF-β to induce AIG (Fig. 6B). As only mTORC2 was required for TGF-β morphologic transformation (Figs. (Figs.3A3A and and5A),5A), these results suggest a dual role for mTOR in the fibroblast response to TGF-β with both mTORC1 and mTORC2 having distinct, but critical actions.
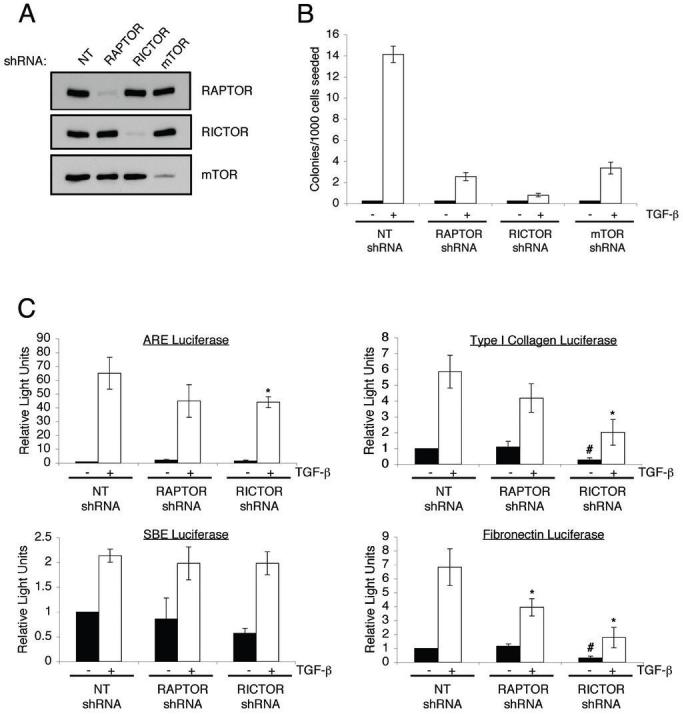
A, AKR-2B cells were transiently transduced with lentiviruses expressing either Non-targeting (NT), RAPTOR targeting, RICTOR targeting, or mTOR targeting shRNAs. 72 hours post-transduction cells were lysed and Western blots performed with indicated antibodies to show loss of the specific protein targeted. B, AKR-2B cells were transduced with lentivirus as in panel A and 72 hours post-transduction were seeded in soft agar with (white) or without (black) 5 ng/ml TGF-β. Quantitation of colony formation after 10 days of growth is shown. Data represent mean ± standard error from 3 experiments done in triplicate. C, AKR-2B cell lines stably expressing Non-targeting (NT), RAPTOR targeting, or RICTOR targeting shRNAs were transiently transfected with ARE, SBE, Type I collagen, or Fibronectin responsive luciferase reporter constructs, serum-starved overnight, and left untreated (black) or stimulated (white) with 5 ng/ml TGF-β for 24 hours. Relative lights units are presented with all values relative to unstimulated control cells. Mean ± standard error relative to unstimulated control cells of 3-4 independent experiments done in triplicate are shown. The fold induction values of the Type I collagen reporter for NT shRNA, RAPTOR shRNA, and RICTOR shRNA are 5.8±1.0, 4.2±0.9, 8.0±0.8. The fold induction values of the FN reporter for NT shRNA, RAPTOR shRNA, and RICTOR shRNA are 6.8±1.3, 3.5±0.6, 5.5±0.7. Asterisk and pound symbols represent statistical significance (p<0.05) difference in relative light units compared to control cells using an independent two sample t-test. * is compared to NT shRNA cells treated with TGF-β while # is compared to untreated NT shRNA cells.
The role of mTOR complexes in TGF-β transcriptional responses
The inability of long-term rapamycin treatment to inhibit mTORC2 activity in AKR-2B cells suggests that experiments utilizing rapamycin to investigate TGF-β-dependent transcription (Fig. 3D) are only addressing the role of mTORC1. To more conclusively determine the impact of mTORC2 in these transcriptional responses, we utilized AKR-2B cell lines stably expressing RAPTOR and RICTOR targeting shRNAs. As shown in Fig. 6C, neither RAPTOR nor RICTOR knockdown had any overt effect on TGF-β mediated induction of the ARE (Smad2-dependent) or SBE promoters (Smad3-dependent). While statistical analysis indicates a slight attenuation of ARE activity in the RICTOR knockdown cells, it is unclear whether it is biologically significant. Interestingly, as opposed to the results using rapamycin (Fig. 3D), RAPTOR knockdown cells exhibit a modest decrease in TGF-β mediated fibronectin and Type I collagen promoter activity (Fig. 6C). These results suggest distinct effects of long-term vs. acute pharmacological inhibition of mTORC1. Interestingly, the most pronounced effect occurred in the RICTOR knockdown cells which show a reduction in both the basal and TGF-β stimulated activity of the ECM promoters relative to control cells (Fig. 6C). However, the fold induction in the RICTOR knockdown cells was comparable to control cells (see Fig. 6 legend), suggesting that while mTORC2 is necessary for efficient activity of a basal regulatory element, it plays no significant role in regulating TGF-β mediated induction of the Type I collagen and fibronectin promoters. While the mechanisms regulating this effect are unknown, these findings indicate multiple roles for mTOR complexes in regulating profibrotic signaling.
DISCUSSION
Given its known role in fibrotic diseases and desmoplasia, we have focused on defining the targets through which TGF-β stimulates fibroblast activation. To that end, a number of fibroblast-specific non-Smad signaling pathways have been identified regulating this response (3, 5, 39). Currently, the most upstream effector is PI3K which independently leads to the activation of PAK2 and Akt (3, 5). Of note, another TGF-β effector activated by PAK2 in a subset of fibroblast, not epithelial, cell lines is the c-Abl non-receptor tyrosine kinase (39). Not only does this finding tie tyrosine kinase activity to a plasma membrane receptor serine/threonine kinase cascade, the c-Abl inhibitor Imatinib Meslylate/Gleevec prevents TGF-β mediated fibroblast proliferation in vitro and attenuates bleomycin-induced lung fibrosis and ureter obstruction induced kidney fibrosis (41, 42). These in vitro and animal model studies have led to a Phase II clinical trial examining the efficacy of imatinib versus placebo in the treatment of idiopathic pulmonary fibrosis1.
While identifying c-Abl as a PAK2 effector shed new insight into the TGF-β signaling network, the role of Akt remained unclear. As such, in this study we focused on identifying targets downstream of Akt required for TGF-β mediated fibroblast proliferation. These results demonstrate that the serine/threonine kinase mTOR is a critical effector of pro-fibrotic TGF-β signaling (Figs. (Figs.1,1, ,3,3, ,5,5, and and6).6). The lack of inducible phosphorylation of the mTORC1 substrate S6K1 in epithelial cells is consistent with previous data demonstrating that TGF-β fails to activate Akt in epithelia (3). While we do not detect mTORC1 activation following TGF-β treatment of epithelial cultures (Fig. 1), another study demonstrated that NMuMg and HaCaT epithelial cells, which undergo epithelial-mesenchymal transition in response to TGF-β, do induce S6K1 phosphorylation upon TGF-β signaling (43). Although these results would seem to be at odds with our data demonstrating a fibroblast-tropism to mTORC1 activation (Fig. 1), we find a similar increase in mTORC1 activity when NMuMg cells are treated with TGF-β (data not shown), supporting the hypothesis that TGF-β can activate mTORC1 in those few epithelia which possess the ability to gain a mesenchymal phenotype. However, it should be noted that TGF-β mediated activation of mTORC1 in this small subset of epithelial cells does precede conversion to a mesenchymal phenotype (43). The mechanism(s) whereby some epithelial cultures respond to TGF-β by activating mTORC1 clearly requires further investigation. In that regard, it appears that the ability of TGF-β to activate PI3K represents a critical node as it is an upstream target required for mTORC1 activity in both fibroblasts and EMT-responsive epithelial cells (Fig. 2; (3, 43).
Along with demonstrating that mTOR is a critical TGF-β effector in fibroblasts, our results distinguish unique as well as over-lapping activities for mTORC1 and mTORC2. As such, this suggests are a number of areas for future investigations. First, though TGF-β utilizes the same PI3K-Akt-TSC2 pathway to activate mTORC1 as receptor tyrosine kinases, PI3K activation by TGF-β is more complex than previously appreciated. While the early response is independent of new protein synthesis (3), the robust, late activation is prevented by cycloheximide (Fig. 2D). This observation suggests that TGF-β may be inducing this pathway via both direct and indirect mechanisms. We are currently investigating the mechanism and intermediate factor(s) by which TGF-β is coupled to the PI3K-Akt-mTORC1 pathway. Second, it is unclear how mTORC1 is promoting TGF-β mediated AIG since rapamycin does not influence Smad transcriptional responses or induction of ECM components (Fig. 3D). Given the known role of mTORC1 in regulating translation, rapamycin may be preventing the translation of critical TGF-β effectors. Third, it is currently unclear how mTORC2 may be regulating the basal activity of the fibronectin and Type I collagen promoters. Finally, the mTORC2 targets required for TGF-β mediated morphological transformation and AIG are currently unknown. It will be interestingly to determine if known downstream mTORC2 mediators such as Akt, PKCα, and/or P-Rex1 are involved (30-33, 35). Future studies will hopefully elucidate additional potential targets for therapeutic intervention. The identification of mTOR as a critical effector of TGF-β mediated fibroblast proliferation and cytoskeletal rearrangement (Figs. 3B, 3C, ,5A,5A, ,6B)6B) is promising given that there are clinically approved mTOR inhibitors and TGF-β is known to promote fibrotic diseases and desmoplasia (11, 13-15). Furthermore, since rapamycin has been demonstrated to possess anti-cancer and anti-angiogenic properties (44, 45), these agents could target malignant cell growth as well as the associated reactive stromal response. Also, since mTOR represents a cell type-restricted response to TGF-β (Fig. 1), it would not alter other critical functions of this growth factor.
While a great deal of progress has been made in understanding the signaling pathways activated by TGF-β, many questions remain how this single cytokine regulates such a plethora of biological responses. Elucidating these mechanisms will not only shed light on fundamental biological processes, but also provide potential opportunities to modulate aberrant responses contributing to a number of human pathologies.
Acknowledgements
We thank Drs. David Kwiatkowski and David Sabatini for generously providing TSC2 and mLST8 null MEFs, respectively. This work was supported by Public Health Service grants GM-54200 and GM-55816 from the National Institutes of General Medical Sciences and the Mayo Foundation (to E.B.L.).
References
Full text links
Read article at publisher's site: https://doi.org/10.1158/0008-5472.can-08-2146
Read article for free, from open access legal sources, via Unpaywall:
http://cancerres.aacrjournals.org/content/canres/69/1/84.full.pdf
Free to read at cancerres.aacrjournals.org
http://cancerres.aacrjournals.org/cgi/content/abstract/69/1/84
Free after 12 months at cancerres.aacrjournals.org
http://cancerres.aacrjournals.org/cgi/content/full/69/1/84
Free after 12 months at cancerres.aacrjournals.org
http://cancerres.aacrjournals.org/cgi/reprint/69/1/84.pdf
Citations & impact
Impact metrics
Citations of article over time
Alternative metrics
Smart citations by scite.ai
Explore citation contexts and check if this article has been
supported or disputed.
https://scite.ai/reports/10.1158/0008-5472.can-08-2146
Article citations
Vistusertib improves pulmonary inflammation and fibrosis by modulating inflammatory/oxidative stress mediators via suppressing the mTOR signalling.
Inflamm Res, 73(7):1223-1237, 24 May 2024
Cited by: 0 articles | PMID: 38789791
Targeting Pulmonary Fibrosis by SLC1A5-Dependent Glutamine Transport Blockade.
Am J Respir Cell Mol Biol, 69(4):441-455, 01 Oct 2023
Cited by: 4 articles | PMID: 37459644 | PMCID: PMC10557918
Signaling pathway intervention in premature ovarian failure.
Front Med (Lausanne), 9:999440, 25 Nov 2022
Cited by: 6 articles | PMID: 36507521 | PMCID: PMC9733706
Review Free full text in Europe PMC
Senescence Alterations in Pulmonary Hypertension.
Cells, 10(12):3456, 08 Dec 2021
Cited by: 9 articles | PMID: 34943963 | PMCID: PMC8700581
Review Free full text in Europe PMC
Somatic SMAD3-activating mutations cause melorheostosis by up-regulating the TGF-β/SMAD pathway.
J Exp Med, 217(5):e20191499, 01 May 2020
Cited by: 18 articles | PMID: 32232430 | PMCID: PMC7201932
Go to all (64) article citations
Data
Data behind the article
This data has been text mined from the article, or deposited into data resources.
BioStudies: supplemental material and supporting data
Clinical Trials
- (1 citation) ClinicalTrials.gov - NCT00131274
Similar Articles
To arrive at the top five similar articles we use a word-weighted algorithm to compare words from the Title and Abstract of each citation.
A critical role for the mTORC2 pathway in lung fibrosis.
PLoS One, 9(8):e106155, 27 Aug 2014
Cited by: 35 articles | PMID: 25162417 | PMCID: PMC4146613
TGF-β Promotes Metabolic Reprogramming in Lung Fibroblasts via mTORC1-dependent ATF4 Activation.
Am J Respir Cell Mol Biol, 63(5):601-612, 01 Nov 2020
Cited by: 40 articles | PMID: 32668192 | PMCID: PMC7605163
Signaling events downstream of mammalian target of rapamycin complex 2 are attenuated in cells and tumors deficient for the tuberous sclerosis complex tumor suppressors.
Cancer Res, 69(15):6107-6114, 14 Jul 2009
Cited by: 84 articles | PMID: 19602587 | PMCID: PMC2735013
A complex interplay between Akt, TSC2 and the two mTOR complexes.
Biochem Soc Trans, 37(pt 1):217-222, 01 Feb 2009
Cited by: 463 articles | PMID: 19143635 | PMCID: PMC2778026
Review Free full text in Europe PMC
Funding
Funders who supported this work.
NIGMS NIH HHS (7)
Grant ID: GM 55816
Grant ID: GM 54200
Grant ID: R01 GM055816-12
Grant ID: R01 GM054200
Grant ID: R01 GM054200-13
Grant ID: R37 GM055816
Grant ID: R01 GM055816