Abstract
Free full text

Involvement of tissue inhibition of metalloproteinases-1 in learning and memory in mice
Abstract
Tissue inhibitor of metalloproteinases (TIMP-1) is one of the four-member family (TIMPs-1–4) of multifunctional proteins that inhibit matrix metalloproteinases (MMPs). Its expression in the hippocampus is neuronal-activity-dependent and dramatically induced by stimuli leading to long-term potentiation (LTP), suggesting that TIMP-1 is a candidate plasticity protein potentially involved in learning and memory processes. We tested this hypothesis in a hippocampus-dependent task using the new olfactory tubing maze, with mice carrying a null mutation for TIMP-1 (TIMP-1 KO) and mice overexpressing TIMP-1 (TIMP-1 (tg)).
The TIMP-1 KO mice were significantly impaired in making correct odor-reward associations when compared with their respective wild type (WT) littermates, while TIMP-1 overexpressing mice performed better than their WT controls. Both genetically modified mice learned the paradigm and the timing of the task, like their respective WTs, and no olfactory dysfunctioning was observed.
These data suggest that TIMP-1 is involved in learning and memory processes related to the hippocampus, and support the hypothesis that the MMP/TIMP ratio, and hence MMP activity, modulates neuronal plasticity in normal learning and memory processes, while altered proteolytic activity could impair cognitive functions.
1. Introduction
The tissue inhibitor of metalloproteinases-1 (TIMP-1) belongs to a family of multifunctional secreted proteins (TIMPs 1–4) that regulate the proteolytic activity of matrix metalloproteinases (MMPs) [22]. In the central nervous system (CNS), deregulations of the MMP/TIMP balance have been associated with the development of pathological processes (for a review, see [27]) [37,21] and more recently, with behavioral impairments [19,20]. Behavioral data suggesting that TIMP-1 could play a role in neuronal plasticity are supported by anatomical and immunohistochemical data, demonstrating that TIMP-1 expression is induced in the hippocampus after stimuli leading to long-term potentiation (LTP) [24]. Moreover the expression of TIMP-1 is dependent on neuronal activity, which is dramatically induced in the hippocampus and other limbic structures following seizures, and its expression is persistent after seizures in areas of profound axo-dendritic remodelling like the dentate gyrus [26]. Neural network reorganization following specific patterns of neuronal activity produces long-lasting modifications of the synaptic structures, constituing the neurobiological substrate of mnesic processes. Synaptic placticity requires structural rearrangements of pericellular and extracellular matrix (ECM) molecules [10]. For instance, a correlation has been established in animal models of epilepsy between the expression of tenascin-C (a substrate for MMPs) and the induction of changes in synaptic efficiency and remodeling [14,23]. Mutant mice expressing decreased levels and abnormal forms of tenascin-C show neurotransmitter-system alterations and behavioral deficits [15]. Furthemore, an integrin-like matrix receptor is implicated in the stabilization of potentiated synapses following induction of LTP [2], a form of synaptic plasticity required for modifying the efficacy of neural networks that support long-term memory, at least in behavioral experiments using olfacto-mimetic cues (LTP correlation and saturation experiments) (for a review, see [30]), contrary to spatial learning tasks, where some forms of LTP are not required [32]. Together, MMPs and TIMPs are the principal regulators of the ecology of the pericellular environment, especially the turnover of extracellular matrix proteins, bioavailability of trophic factors, and shedding of membrane receptors [36]. From the above, it follows that MMPs and TIMPs could actively contribute to learning and memory processes.
Among the mnesic processes that involve the hippocampus, olfactory learning is of utmost importance for rodents. In rats, olfactory learning increases synaptic efficacy first in the hippocampus, and then in the piriform cortex (olfactory cortex) pyramidal neurons (for a review, see [30]). In order to assess learning and memory in mice with regard to their ethological abilities and genetic backgrounds, a new olfactory tubing maze was specially developed and then demonstrated to be a hippocampus-dependent task [9,29]. In addition, it was recently demonstrated in C57 mice, using this device that TIMP-1 deficient mice exhibited impaired learning capacities [20]. The objective of the present study was to further characterize this learning impairment and to compare the learning abilities of TIMP-1-deficient mice [34] with transgenic mice that overexpress TIMP-1 in a constitutive manner [1], and the data further support the idea that TIMP-1 is a candidate plasticity protein involved in the molecular and cellular mechanisms underlying learning and memory.
2. Materials and methods
2.1. Animals
Mice were bred in our animal facility, had access to food and water ad libitum, and were housed under a 12 h light–dark cycle at 22–24 °C.
TIMP-1-deficient mice were generated as described previously [34] in the 129/SVJae Jac strain by homologous recombination. Functional TIMP-1 protein was detected in wild-type cells, but not in co-isogenic cell lines carrying the mutation in Timp 1. Homozygous TIMP-1 KO mice and WT mice were litter-mates following mating of heterozygous mice, and thus had identical genetic backgrounds. Genomic DNA was extracted from the tail tips of mice to determine the genotype by PCR. Both the TIMP-1 KO and WT groups contained seven mice that were tested in Experiment 1.
TIMP-1 overexpressors were generated by Alexander et al. [1]. Briefly, 4.3 kb of the 5′ sequence from the human beta-actin gene (including 3 kb of flanking sequence, 78 bp of noncoding sequence, and 832 bp of intron 1) were placed upstream of a HindIII cloning site and 877 bp of 3′ untranslated sequence from the same gene were inserted in a pBluescript vector (Stratagene, La Jolla, CA). A full-length human cDNA for TIMP-1 (an EcoRI fragment tailed with HindIII linkers) was inserted, and the entire construct was removed by cleavage with KpnI and XbaI for microinjection into the pronuclei of CD1 mouse eggs using of standard transgenic technology [18]. The average circulating levels of hTIMP-1 heterozygote and homozygote transgenic mice were 300 and 600 ng/ml serum, respectively (N. Ortega, personal communication). Both the CD1 transgenic and WT groups characterized by PCR genotyping contained eight mice that were tested in Experiment 2.
2.2. Olfactory tubing maze
The olfactory tubing maze (Fig. 1) is composed of four testing chambers joined to each other by plastic elbow tubes extended on each side by a straight plastic tube [29]. At each lateral extremity of the testing chambers, a water port in the shape of a well and a buzzer are located above the air and odor port. Entry to or exit from the testing chamber is achieved by opening automated doors. The olfactory maze is set on a table above the floor, with a central circular hole 50 cm in diameter for connecting the internal extremities with the different tubes carrying the neutral air and odor flow.
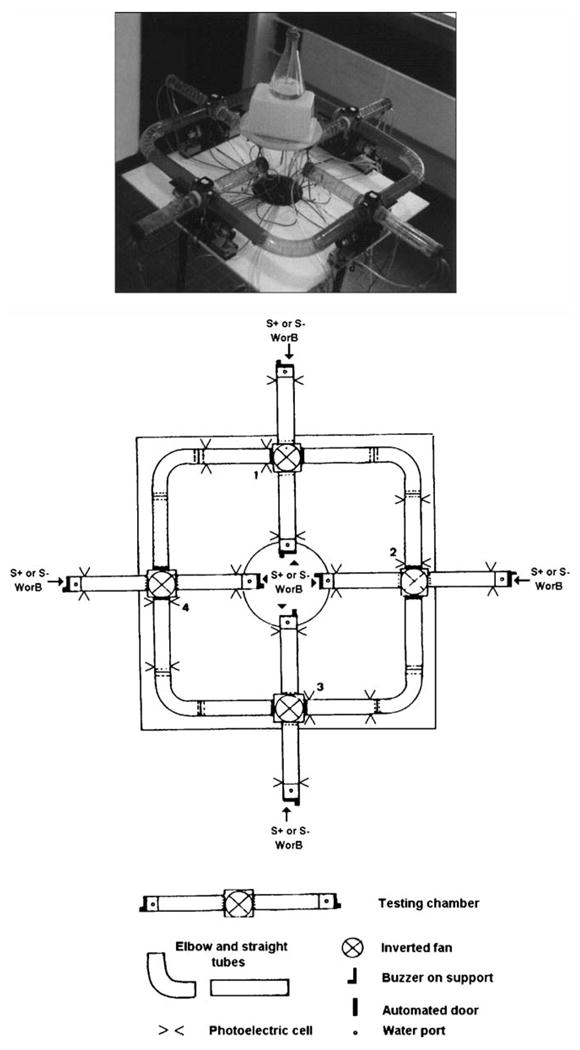
Top panel: photograph of the olfactory tubing maze set on a table, viewed from above. Bottom panel: technical diagram of the tubing maze on a table, on a scale of 1/10. The maze is made up of four testing chambers. Each chamber is numbered (1–4) and consists of an empty cube with an inverted fan on the top and two tubes on the sides, one on the outer side (external) and one on the inner side (internal). Rewards are delivered and neutral or scented air is ejected at the ends of the side tubes. The streaked tube indicates the place where the mouse is introduced into the maze. S+ is the positive stimulus (associated with the water reward). S− is the negative stimulus (associated with the buzzer). W: water; B: buzzer. On each trial, the water or buzzer reward is distributed only at one of the two extremities of the testing chamber, where the mice respond to the presentation of odors. S+ and S− stimuli are ejected in all testing chambers on opposite sides, and the side for S+ and S− is pseudo-randomly assigned across trials.
At least one week before the first session, all male adult mice approximately 8 weeks old were individually housed. For Experiments 1 and 2, on the first day starting at 8:00 a.m., all mice were weighed, handled for 5 min, and then placed in the olfactory maze for 5 min. The doors were opened, with free access to all areas. All water ports were loaded with 0.1 ml of water. Then the mice were returned to the vivarium and water deprived. On the second day, the mice were again weighed, as they were every day, before being reintroduced into the maze, with free access to all testing chambers. As on the first day, all water ports were loaded once with 0.1 ml of water. After 20 min of the shaping session, the mice had usually drunk all the water available in the cup at every extremity of the maze. One day later, the training started. At the beginning of the training session, the top half of the tube (streaked tube in Fig. 1) located between the fourth and first testing chambers was lifted to introduce the mouse into the apparatus, and then put back on, with the mouse in the maze. Before the automated door opened, the mouse could wait anywhere in the compartment. At this time, two different odors were ejected from the right and left tubes, respectively. One odor (positive odor) was associated with the positive reward [a drop of water (0.03 ml)] while the other (negative odor) was associated with a 3-s buzzer sound. Then the mouse had to make a right or left turn to go to the end of one of the tubes. When the choice was made, the mouse ran through one of the lateral tubes and crossed the photoelectric cell beam at the end of the tube, releasing either the water or the buzzer sound, depending on the odor ejected in the tube. Once the positive or negative reward was released, the entrance door closed and the outside door of the testing chamber opened. The mouse had to backtrack to the testing chamber to go into the second compartment and gain access to the next testing chamber, which started the second trial, and so on. For the training procedure, a non-correction method was applied. The animal could not correct an error after a wrong response on any trial and no water was delivered if an error was made. During the intertrial period, neutral air was ejected from all testing-chamber extremities.
2.3. Training procedure
A session consisted of 20 trials. On each trial, the positive odor was randomly assigned to the left or right tube, and inversely for the negative odor. Once per trial, the water or the buzzer was delivered depending on the mouse's choice. At the end of the training session, the outside of the fourth testing chamber remained closed, and the mouse was removed and returned to the vivarium, where it had free access to water from 2:00 to 2:05 p.m. The stream of neutral or scented air was ejected constantly from the eight lateral tube extremities.
The entire procedure was controlled by a microcomputer from a program developed in our lab. No more than three successive trials could be made with the same choice (i.e., distribution of the same odor from the same side, for example, S− external and S+ internal). This was disigned to prevent the mice from developing a locomotor strategy.
Three parameters were examined. (1) The first was the percentage of correct responses, which was the ratio of the number of correct responses to the total number of odor presentations (20 in these three experiments). This percentage is used to evaluate the effectiveness of the association between an odor and its reinforcement, a process which falls under the hippocampal-dependent declarative memory subcategory, when an animal has to perform the task with two simultaneous odors [9,11]. (2) The second was the intertrial interval, which is equal to the time between the response to an odor presentation in one testing chamber and the response in the next chamber. The minimum intertrial interval was set at 15 s. Changes in the intertrial interval reflect the fact that, after a (correct or incorrect) response to an odor, the animal must learn to backtrack to the testing chamber and run to the next chamber to wait for the entrance door to open for the next trial. This constitutes a procedural aspect of the task. In this task, if an animal waits in front of the testing room door after the door opens or waits in front of the water port, or if it looks for water after a response and the distribution of the corresponding reinforcement, it means that the animal has not mastered the procedural aspect of the task [9]. In this case, the intertrial interval (ITI) will be high. During the successive trials, if the animal learns the procedure, the ITI will decrease. Mean ITI is the sum of the time elapsed between each pair of successive trials, divided by 19. (3) The third parameter was latency, which corresponds to the time between the entrance door of the testing room, from where the mouse can smell the different odors ejected from the lateral tubes, and the response given at the end of one of the two tubes. The mean time taken to respond to the odor is taken as an indication of the proper functioning of the olfactory system. A mean latency decrease is usually observed across sessions until this mean latency reaches its floor level when the mouse has learned that it can get water once odors are presented. Further information about the olfactory tubing maze and training procedure can be found in [29].
The daily training session was held until one group of mice (WTs or their respective TIMP-1KO (Experiment 1) or TIMP-1 (tg) mutants (Experiment 2)) reached a level of 80 ± 10% correct responses. Two weeks later, all mice were submitted to a reversal session in which the valences of the previous odors were reversed. In general, when mice reach a significant level of 80 ± 10% correct responses, the percentage of correct responses obtained in the reversal session is low enough to be different from the chance level, demonstrating the long-term memory of the learning of the previous odor's meaning.
2.4. Statistical analysis
All data were analyzed in multivariate analyses of variance (MANOVAs) with repeated mesures, using the SPSS/PC+ statistics 11.0 software (SPSS Inc., Chicago, IL). Behavioral performance (percentage of correct responses) in comparison to chance levels was processed using a one-sample t-test. In the figures: *p < 0.05, **p < 0.01, and ***p < 0.001.
3. Results
In Experiment 1, a learning effect across the seven training and reversal sessions was observed for each group, WT (129/SV) and TIMP-1 KO (129/SV littermates), on the percentage of correct responses, intertrial interval, and latency (MANOVAs, F(14,126) = 5.56, p < 0.001 and F(14,126) = 1.89, p < 0.05). Consequently, no significant differences were found between the two groups (MANOVA, F(14,252) = 1.62, NS) (Fig. 2).

Mean performance (±S.E.M.) on the olfactory discriminative association task using two different odors, for TIMP-1 KO (in 129/SVJae background) (N = 7) and WT littermates (129/SVJae) (N = 7) mice. (A) Mean percentage of correct responses. The dashed line denotes the chance level. (B) Mean intertrial interval (in s). The dashed line indicates the minimum fixed intertrial interval (15 s). (C) Mean latency (in s) in responding to odor presentations. TIMP-1 KO mice were significantly impaired on the percentage of correct responses but not on the intertrial interval or on latency. Rev: reversal.
Nevertheless, the WT mice improved their correct-response level across the seven sessions and obtained a low score on the reversal session (MANOVA, F(7,42) = 5.92, p < 0.001). In contrast, the TIMP-1 KO mice performed close to chance level throughout all sessions (MANOVA, F(7,42) = 1.85, NS) (Fig. 2A).
A significant decrease in the intertrial interval across sessions was observed for both groups (MANOVAs, WT: F(7,42) = 9.65, p < 0.001; TIMP-1 KO: F(7,42) = 5.33, p < 0.001) (Fig. 2B), with no significant difference between the two groups (MANOVA, F(7,84) = 1.87, NS). Concerning the response latency to odors, a decrease was observed during the first four sessions (MANOVA, WT: F(7,42) = 5.72, p < 0.001; TIMP-1 KO: F(7,42) = 11.26, p < 0.001) (Fig. 2C); it was equivalent in WT and mutant mice (MANOVA, F(7,84) = 0.94, NS).
From these results, it appears that TIMP-1 KO mice were able to reduce their intertrial interval and latency, but unlike WT, they were unable to make consistent odor-reward associations. Indeed from the fourth session on, a significant difference in the percentage of correct responses appeared during the reversal sessions between the TIMP-1 KO mice and the WT mice (Fig. 2A) (MANOVA, F(4,48) = 2.56, p = 0.05). This difference was observed on the fourth, sixth, seventh, and reversal sessions, where WT mice obtained a percentage of correct responses significantly above chance (t-test, t6 ≤ 2.5 and t6 = −2.62, p ≤ 0.05). This difference appeared for the TIMP-1 KOs only on the seventh session (t6 = 2.89, p < 0.05).
A detailed analysis by block of trials revealed that from the fourth session on (S4) (Fig. 3), three blocks were different from chance level for the WT mice (t6 ≥ 3.24, p ≤ 0.05), whereas only one block differed from chance for TIMP-1 KO mice (t6 = 3.87, p < 0.01). On session 5 (S5), one block was different in the WT group (t6 = 2.52, p < 0.05) but none differed in the TIMP-1 KO group. On session 6, two blocks were different for WT mice (t6 ≥ 3.28, p ≤ 0.05) but none were for TIMP-1 KO mice. On the last training session (S7), all trial blocks were different in the WT group (t6 ≥ 2.51, p ≤ 0.05) but only one was so in the TIMP-1 KO group (t6 = 2.52, p < 0.05). This difference in performance between WT and mutant mice on odor-reward associations is consistent with the differing percentages of correct responses exhibited by the two strains during the reversal session (Rev). In the WT group, two blocks were significally different from chance (t6 ≤ −2.5, p ≤ 0.05) but none were so in the TIMP-1 KO group.
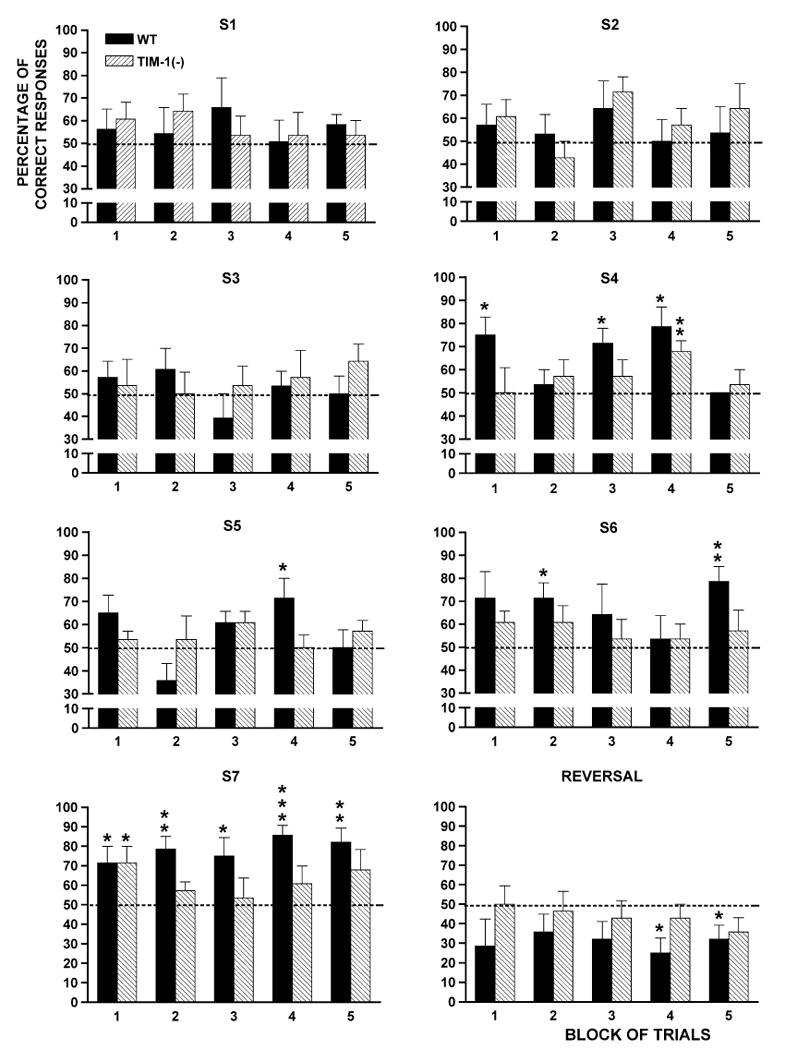
Evolution of the percentage of correct responses, by block of four trials, reached by the 129/SVJae TIMP-1 KO and WT mice across the seven successive daily sessions (S) and 2 weeks later on a reversal session. The impairment of the TIMP-1 KO mice in making the correct odor-reward associations appeared on the fourth session. A profound deficit was observed on session seven (S7) where, for the TIMP-1 KO, only one block was different from the chance level instead of all blocks for the WT mice. On the reversal session, the TIMP-1 KO mice performed near the chance level, while WT mice still remembered the meaning of the odors learned two weeks earlier.
The transgenic mice overexpressing TIMP-1 (tg) were generated in a CD1 background, unlike the TIMP-1 KOs, which were generated in a 129SV background. So the CD1 TIMP-1 tg were compared to their CD1 WT littermates. It is known, and was further confirmed in our study, that mice with a CD1 genetic background have better learning capacities than 129SV mice.
In Experiment 2, the CD1 WT and the TIMP-1 (tg) mice increased their percentage of correct responses, decreased the intertrial interval and latencies across the seven sessions, and remembered the odor-reward associations during the reversal session (MANOVAs, F(8,84) = 4.9 and F(8,84) = 11.42, p < 0.001), with no significant difference between the two mouse groups (MANOVA, F(8,168) = 1.16, NS) (Fig. 4). Both groups improved the percentage of correct responses across the seven learning sessions and obtained a below-chance level score during the reversal session (MANOVAs, WT: F(4,28) = 5.26, p < 0.01; TIMP-1 (tg): F(4,28)= 15.96, p < 0.001) (Fig. 4A), and no significant difference between the two groups was observed on these parametres (MANOVA, F(4,56) = 1.46, NS). A significant decrease in the intertrial interval was found for the WT (MANOVA, F(4,28) = 7.35, p < 0.001) and TIMP-1 (tg) groups (MANOVA, F(4,28) = 7.05, p < 0.001), but without a significant difference between them across sessions (F(4,56) = 0.59, NS) (Fig. 4B). Similarly, a latency decrease was obtained for in both groups across sessions, particularly between sessions 1 and 2 and sessions 2 and 3 (MANOVAs, WT: F(4,28) = 14.77, p < 0.001; TIMP-1 (tg): F(4,28) = 8.32, p < 0.001). These data suggest that the two groups of mice had a similar capacity for associative learning F(4,56) = 1.29, NS) (Fig. 4C). However, one difference between WT and overexpressors was that the latter performed faster and at a higher level in the odor-reward associations on sessions 2 and 4 and on the reversal session (session 2, t7 = 3.2, p < 0.05; t7 = 1.26, NS; session 4 t7 = 6.56, p < 0.001; t7 = 3.64, p < 0.01). Also, the transgenic mice remembered these associations better during the reversal session (t7 = −3.39, p < 0. 01; t7 = −2.56, p < 0.05).

Mean performance (±S.E.M.) on the olfactory discriminative association task using two different odors, for CD1 TIMP-1 (tg) (N = 8) and WT (N = 8) mice. (A) Mean percentage of correct responses. The dashed line denotes the chance level. (B) Mean intertrial interval. The dashed line indicates the minimum fixed intertrial interval (15 s). (C) Mean latency (in s) in responding to odor presentations. TIMP-1 (tg) showed slight but significant improvement on the percentage of correct responses and obtained similar performance on the intertrial interval and latency in comparison to the WT mice. Rev = reversal.
Indeed, detailed session-by-session analyses of the percentage of correct responses by block of trials (Fig. 5) for the WT and TIMP-1 (tg) groups indicated the onset of learning of the odor-reward associations as early as the first session for both groups (t7 = 2.4, p < 0.05; t7 = 2.97, p < 0.05). On session 2, one block for each group was significantly different from the chance level (WT: t7 = 3.42, p < 0.05; TIMP-1 (tg): t7 = 3.86, p < 0.01). On session 3, the last two blocks were significantly different in the WT mice (t7 ≥ 2.97, p < 0.05) and in only one block for the TIMP-1 (tg) mice (t7 = 2.4, p < 0.05). On the last training session (S4), the TIMP-1 (tg) mice performed more consistently overall than the WT mice; in this case, three of the five blocks were different from chance level (t7 ≥ 2.4, p ≤ 0.05) for TIMP-1 (tg) mice but only two blocks were different for WT mice (t7 ≥ 2.64, p ≤ 0.05). The better performance of the mutant mice on the last training session was correlated with better performance on the reversal session two weeks later. While the TIMP-1 (tg) group exhibited fewer correct responses, with two blocks significantly different from chance (t7 ≤ −2.65, p ≤ 0.05), the WT mice only had one block that was different (t7 = −3.7, p < 0.01).

Evolution of the percentage of correct responses, by block of four trials, reached by the CD1 TIMP-1 (tg) and WT mice across the four successive sessions (S) and 2 weeks later on a reversal session. The slight improvement for the TIMP-1 (tg) mice in learning to make consistent odor-reward associations was observed on session 4 and during the reversal session, in comparison to WT mice.
4. Discussion
One question immediately raised by our results is whether altering the MMP/TIMP balance has an effect on the sense of olfaction in mutant mice. However, the behavior of the mice in the olfactory tubing maze indicates that changes in TIMP-1 expression did not interfere with their capacity to smell odors and to respond to odor presentation (i.e., latency performance) in the same way as their respective WT controls. This strongly suggests that the olfactory system was not significantly affected in the mutant mice, nor was their ability to learn the procedural aspect of the task (i.e., intertrial interval performance). Conversely, our analyses of the percentage of correct responses revealed that TIMP-1 KO mice were significantly impaired for making and remembering the correct odor-reward associations, while the TIMP-1 (tg) mice demonstrated a slight but significant improvement across sessions in the learning and memory of these associations.
These results may be related to recent data demonstrating that selective bilateral lesions of the hippocampal formation cause selective impairment of subcategories of long-term memory in mice tested in the olfactory tubing maze [9]. Indeed, a dissociation between brain mechanisms for “learning how” versus “learning where” to navigate was recently attributed respectively to thalamic and hippocampal mechanisms in spatial navigation [6,7]. Rats with medial thalamic lesions were impaired in water-maze strategy learning, which could be related to the procedural aspect of the task, while the hippocampal formation was involved in spatial mapping and memory and could be related to declarative memory in humans. This clear dissociation was demonstrated both with and without a non-spatial pretraining procedure in lesioned rats [6]. In the present experiments, mice were placed in the olfactory tubing maze with free access to all testing chambers for two sessions, which can be considered as a pretraining procedure. In addition, mice with hippocampal lesions [9] without locomotor deficits did not have any procedural or strategic impairments, as in the present experiment, and so the deficit was attributed to hippocampal dysfunctioning. This impairment has been ascribed to an alteration of relational processes [11–13] or to a deficit in the processes required to store odor-reward associations [8,28]. Both hypotheses used to account for the processing of incoming olfactory information are hippocampus-dependent and related to declarative or reference memory. Thus, whether their performance was significantly impaired or slightly improved for learning the odor-reward associations, the mutant mice did not learn the procedural aspect of the task differently from their respective WT controls. The results obtained with mice in the olfactory tubing maze resemble those obtained with human and non-human primates that led Squire and Zola [35] to propose a taxonomy of long-term memory. These authors concluded that declarative memory processed by the hippocampus was indeed disturbed in amnesia, aging, or during the initial stage of Alzheimer's disease. In keeping with these findings, we postulate that the imbalance in the MMP/TIMP system affects this category of long-term memory. Another hypothesis would be that both hippocampal-lesioned and TIM-1KO/Tg mice display normal intertrial interval and latency performance (rule-learning) and consequently normal learning of general strategies to cope with the olfactory associative task, while being significantly impaired in learning the information (meaning associated to olfactory cues) necessary for correctly performing it, which is also hippocampus-dependent and related to long-term memory [6,7].
In the CNS, it has been postulated that changes in the MMP/TIMP ratio and MMP activity underlie some important developmental and pathological processes [21,27,37]. The expression of TIMP-1 is dramatically increased in the hippocampus by stimuli leading to seizures [26] or LTP [24], which is required in the hippocampus and piriform cortex for learning and long-term memory of odor-reward associations (for a review, see [30]).
These reports, added to previous data [20] and the present findings, provide evidence that TIMP-1 contributes to the plasticity mechanisms involved in learning and long-term memory of hippocampus-dependent tasks. One can hypothesize that MMP activities contribute to plasticity through the regulation of neurotransmitter receptors or ion channels, since it is already becoming evident that the intracellular domains of receptors or channels are cleaved by intracellular proteinases. Bi et al. [4,5] demonstrated that intracellular calpain activation produces a partial proteolysis in the intracellular C-terminal domain of the GluR1 subunits of the α-amino-3-hydroxy-5-methylisoxazole-4-propionic acid receptors and of the NR2 subunits of the N-methyl-d-aspartate receptors [3,31]. Calpain also cleaves an L-type Ca2+ channel in hippocampal neurons, leading to a truncated channel with increased activity [17]. From these observations, it is conceivable that the extracellular domains of receptors or channels may also be targets for secreted proteinases, providing additional mechanisms for regulating neuronal plasticity related to cognitive functions. Several lines of evidence and work carried out on other extracellular proteinases support this idea. In this respect, the tissue plasminogen activator (tPA), a secreted serine proteinase, cleaves the extracellular domain of the NR1 receptor and increases the channel's efficacy. tPA is also induced in the hippocampus by LTP stimuli [25] and in the cerebellum during motor learning tasks [33]. Moreover, tPA-deficient mice show altered LTP [16].
A number of studies should be carried out in the future to complement this first observation that the MMP/TIMP ratio influences learning and memory processes. Such studies should test the learning and memory of successive odor pairs, but also in vivo LTP in TIMP-1-deficient or overexpressing mice
Acknowledgments
This research was supported by funding from CNRS, Université de Provence, Université de la Méditerranée, and the National Institute of Health, USA (grant R01 NS039278 to Z. Werb). J. Jourquin was granted a doctoral fellowship from the French Ministry of Research.
References
Full text links
Read article at publisher's site: https://doi.org/10.1016/j.bbr.2006.06.020
Read article for free, from open access legal sources, via Unpaywall:
https://europepmc.org/articles/pmc2659720?pdf=render
Citations & impact
Impact metrics
Citations of article over time
Alternative metrics
Smart citations by scite.ai
Explore citation contexts and check if this article has been
supported or disputed.
https://scite.ai/reports/10.1016/j.bbr.2006.06.020
Article citations
Phosphorylation of RPT6 Controls Its Ability to Bind DNA and Regulate Gene Expression in the Hippocampus of Male Rats during Memory Formation.
J Neurosci, 44(4):e1453232023, 24 Jan 2024
Cited by: 0 articles | PMID: 38124005 | PMCID: PMC10860611
Roles of Matrix Metalloproteinases and Their Natural Inhibitors in Metabolism: Insights into Health and Disease.
Int J Mol Sci, 24(13):10649, 26 Jun 2023
Cited by: 16 articles | PMID: 37445827 | PMCID: PMC10341707
Review Free full text in Europe PMC
Matrix Metalloproteinases and Their Inhibitors in Pulmonary Fibrosis: EMMPRIN/CD147 Comes into Play.
Int J Mol Sci, 23(13):6894, 21 Jun 2022
Cited by: 28 articles | PMID: 35805895 | PMCID: PMC9267107
Review Free full text in Europe PMC
Astrocyte HIV-1 Tat Differentially Modulates Behavior and Brain MMP/TIMP Balance During Short and Prolonged Induction in Transgenic Mice.
Front Neurol, 11:593188, 15 Dec 2020
Cited by: 11 articles | PMID: 33384653 | PMCID: PMC7769877
MMP-2: A modulator of neuronal precursor activity and cognitive and motor behaviors.
Behav Brain Res, 333:74-82, 27 Jun 2017
Cited by: 11 articles | PMID: 28666838
Go to all (27) article citations
Data
Similar Articles
To arrive at the top five similar articles we use a word-weighted algorithm to compare words from the Title and Abstract of each citation.
Tissue inhibitor of metalloproteinases-1 (TIMP-1) modulates neuronal death, axonal plasticity, and learning and memory.
Eur J Neurosci, 22(10):2569-2578, 01 Nov 2005
Cited by: 52 articles | PMID: 16307599
Strain differences in rewarded discrimination learning using the olfactory tubing maze.
Behav Genet, 36(6):923-934, 20 Jun 2006
Cited by: 3 articles | PMID: 16786425
Timp-3 deficiency impairs cognitive function in mice.
Lab Invest, 89(12):1340-1347, 05 Oct 2009
Cited by: 14 articles | PMID: 19806081 | PMCID: PMC3047444
Effects of gene deletion of the tissue inhibitor of the matrix metalloproteinase-type 1 (TIMP-1) on left ventricular geometry and function in mice.
J Mol Cell Cardiol, 32(1):109-120, 01 Jan 2000
Cited by: 70 articles | PMID: 10652195
Funding
Funders who supported this work.
NINDS NIH HHS (2)
Grant ID: R01 NS039278-09
Grant ID: R01 NS039278