Abstract
Free full text

MYOSIN PHOSPHATASE TARGETING SUBUNIT1 REGULATES MITOSIS BY ANTAGONIZING POLO-LIKE KINASE1
SUMMARY
Myosin phosphatase targeting subunit1 (MYPT1) binds to the catalytic subunit of protein phosphatase1 (PP1C). This binding is believed to target PP1C to specific substrates including myosin II, thus controlling cellular contractility. Surprisingly, we found that during mitosis mammalian MYPT1 binds to polo-like kinase1 (PLK1). MYPT1 is phosphorylated during mitosis by proline-directed kinases including cdc2, which generates the binding motif for the polo box domain of PLK1. Depletion of PLK1 by small interfering RNAs is known to results in loss of γ-tubulin recruitment to the centrosomes, blocking centrosome maturation, leading to mitotic arrest. We found that co-depletion of MYPT1 and PLK1 reinstates γ-tubulin at the centrosomes, rescuing the mitotic arrest. MYPT1 depletion increases phosphorylation of PLK1 at its activating site (Thr210) in vivo, explaining, at least in part, the rescue phenotype by co-depletion. Taken together, our results identify a previously unrecognized role for MYPT1 in regulating mitosis by antagonizing PLK1.
INTRODUCTION
Protein phosphatase1 (PP1) is known to play critical roles in a variety of mitotic events. In S. pombe (Ohkura et al., 1989), as well as in Aspergillus (Doonan and Morris, 1989) and S. cerevisiae (Bloecher and Tatchell, 1999), mutations of its catalytic subunit (PP1C) have been demonstrated to cause defects in chromosome separation. In Drosophila, a PP1C mutation results in multiple effects including defective spindle organization and abnormal sister chromatid segregation (Axton et al., 1990). In mammalian cells, PP1C inactivation results in failure in anaphase progression, as well as in mitotic exit (Fernandez et al., 1992) and cytokinesis (Cheng et al., 2000).
These pleiotropic effects indicate that PP1C controls the phosphorylation states of diverse substrates in different locations and at various steps of cell division. Because PP1C itself has a broad substrate specificity, one critical question is how PP1C targets specific substrates at the right time and in the right place. Recent studies emphasize the critical role of PP1C targeting subunits in targeting PP1C to specific substrates at precise locations (Ceulemans and Bollen, 2004; Cohen, 2002). More than 50 targeting subunits have been reported to form a complex with PP1C, and this would generate many forms of the phosphatase with distinct substrate specificities and/or intracellular targeting ability. Such complex formation with various targeting subunits provides a means for the limited number of PP1C isoforms to antagonize a wide array of Ser/Thr kinases with different substrate specificities. Two recent studies, for example, have demonstrated that Repo-Man, a novel PP1C targeting protein, is essential for recruiting PP1Cγ, one of three mammalian PP1C isoforms, to chromatin during anaphase and plays a critical role in the maintenance of chromosome architecture (Trinkle-Mulcahy et al., 2006; Vagnarelli et al., 2006). Because of the complexity of temporal and spatial control of mitotic events, the multitude of PP1C targeting subunits must be involved. Despite the wealth of knowledge regarding the regulation of mitotic kinases, little is known of how PP1C targeting molecules control various aspects of PP1Cs’ functions in mitotic progression, or which serine/threonine kinases they antagonize.
Myosin phosphatase targeting subunit1 (MYPT1, also called MBS or M130) is a known regulator of PP1C (Cohen, 2002; Hartshorne et al., 2004). MYPT1 binds to PP1Cβ and to a 20kDa subunit of unknown function, to form a hetrotrimeric holoenzyme. MYPT1 also binds to certain substrates, bringing that substrate and the catalytic subunit together. The MYPT1-containing holoenzyme is often called myosin phosphatase because it effectively dephosphorylates the regulatory light chain of myosin II and controls actomyosin contractility in smooth muscle and nonmuscle cells. This holoenzyme has also been shown to dephosphorylate, at least in vitro, other substrates including adducin, moesin, tau, MAP2 and a transcriptional repressor HDAC7, suggesting that MYPT1 could have broader functions than myosin regulation (Matsumura and Hartshorne, 2008).
We previously showed that MYPT1 is specifically phosphorylated during mitosis (Totsukawa et al., 1999). Here we identify these mitosis-specific phosphorylation sites, revealing that MYPT1 is phosphorylated by proline-directed kinases including cdc2 kinase. This phosphorylation generates a binding motif for the polo-box domain (PBD) of polo-like kinase1 (PLK1). Indeed, MYPT1 directly binds to PLK1 via its PBD, co-immunoprecipitates with PLK1 as a complex, and co-localizes with PLK1 on centrosomes, as well as on kinetochores.
PLK1 is an essential mitotic kinase that is involved in a variety of mitotic events including the G2/M transition, centrosome maturation and separation, mitotic spindle formation, chromosome segregation and cytokinesis (Barr et al., 2004; Blagden and Glover, 2003; Dai and Cogswell, 2003). As expected from PLK1’s multiple functions, PLK1 phosphorylates a diverse set of substrates and changes its subcellular localization from the centrosome and kinetochore during prophase and metaphase, to the central spindle during anaphase and telophase. However, it is not well understood how the activity of PLK1 is controlled during execution of these diverse mitotic events, or what phosphatase antagonizes PLK1 in vivo.
The physical association between MYPT1 and PLK1 suggests that MYPT1/PP1C phosphatase is involved in PLK1-mediated mitotic events. In mammalian cells, PLK1 depletion by siRNA or PLK1 inactivation by antibody injection is known to block bipolar spindle assembly, resulting in mitotic arrest and apoptosis (Lane and Nigg, 1996; Liu and Erikson, 2002; Sumara et al., 2004; van Vugt et al., 2004). We found that simultaneous reduction of MYPT1 and PLK1 expression rescues these phenotypes. Importantly, MYPT1 depletion increases phosphorylation of PLK1 at Thr210, a site responsible for the activation of PLK1, and MYPT/PP1C is able to dephosphorylate PLK1 at Thr210 in vitro. Our results indicate that MYPT1/PP1C is a phosphatase that counteracts PLK1, and suggest a new mode of kinase regulation by a PP1C targeting subunit: phosphorylation of a PP1C targeting subunit recruits a kinase to its antagonizing phosphatase, allowing regulation of kinase’s activity.
RESULTS
Cdc2 phosphorylates mitosis-specific sites in MYPT1
As a first step toward understanding the significance of the mitosis-specific phosphorylation of MYPT1, we identified its phosphorylated residues. Using a series of truncation mutants coupled with analyses by phosphopeptide mapping of point mutants at possible phosphorylation sites, we found that MYPT1 residues Ser432, Ser473, and Ser601 were phosphorylated during mitosis (Fig. 1A). These residues are located in the central region of MYPT1 and had no previously assigned function (D). Because all three phosphorylation sites are followed by proline, we tested whether a proline-directed kinase such as cdc2 can phosphorylate MYPT1 at these sites. As Fig. 1B shows, cdc2 kinase stoichiometrically phosphorylated full-length MYPT1: radioisotope experiments revealed that approximately 3 moles of PO4 per mol of full-length MYPT1 were incorporated (data not shown). Phosphopeptide mapping (C) revealed that cdc2 kinase was able to phosphorylate each of the three mitosis-specific sites. Another proline-directed kinase, MAPK, could also phosphorylate these same sites (data not shown).
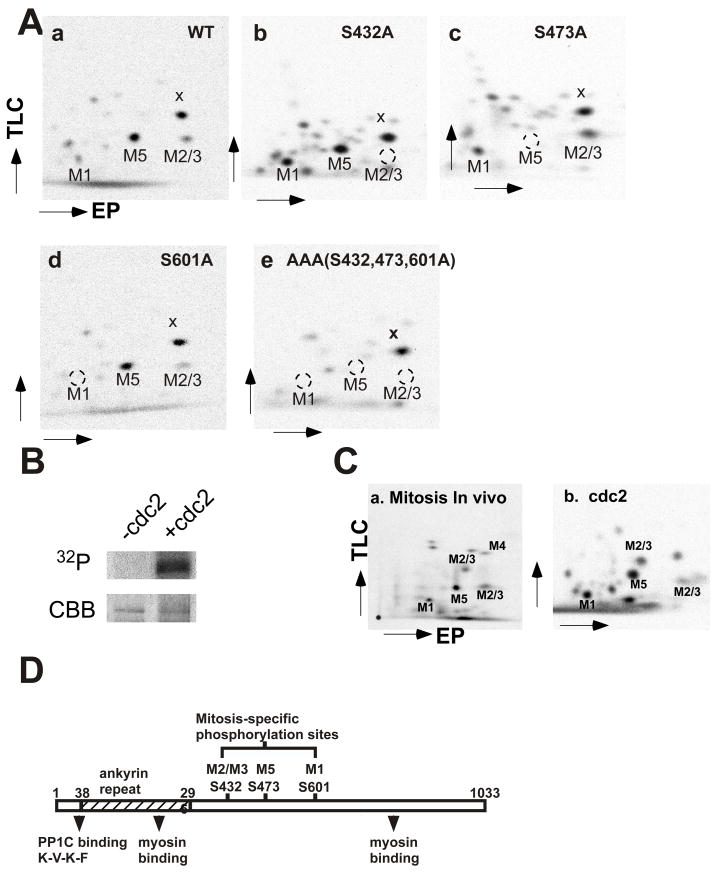
Identification of the mitosis-specific phosphorylation sites of MYPT1. A, Phosphopeptide map analyses of wild-type (a), as well as single point mutants of S432A (b), S473A (c), S601A (d) and a triple mutant (e). M2/3 spot disappeared by S432A (Ser432 replaced with Ala) mutation (b), M5 by S473A mutation (c); M1 by S601 mutation (d). M1, M2/3 and M5 all disappeared by triple mutation (e). X is a Xenopus specific spot. The directions of the first dimension (electrophoresis, EP) and the second dimension (thin layer chromatography, TLC) are indicated by arrows. B, In vitro phosphorylation of MYPT1 by cdc2. Upper panel, autoradiography; lower panel, Coomassie brilliant blue staining. C, Phosphopeptide map analyses of MYPT1 phosphorylated in vivo (a) and phosphorylated in vitro by cdc2 (b). Phosphorylation at the M2/3 site sometimes gave two spots as shown here (Totsukawa et al., 1999). D, diagram of rat MYPT1 indicating locations of the mitosis-specific phosphorylation sites.
Association of MYPT1 and PLK1
Interestingly, we noted that phosphorylation at Ser473 and Ser601 constitutes a motif (S-phosphoS-P, see Fig. 2A for the Ser473 phosphorylation site) for binding to the polo-box domain (PBD) of PLK1 (Elia et al., 2003a). Indeed, we could co-immunoprecipitate MYPT1 and PLK1 from lysates of mitotic cells. Western blotting revealed that MYPT1 immunoprecipitates from mitotic cells contained both PLK1 and PP1C (Fig. 2B, lane 4). The level of Aurora B in the immunoprecipitate, on the other hand, was much lower than that of PLK1, suggesting a specificity of the association between MYPT1 and PLK1. In a reciprocal experiment, we found that the PLK1 immunoprecipitates from mitotic cells also contain MYPT1 and PP1C (lane 5). A negative control of EB1 immunoprecipitates contained neither PLK1 nor MYPT1 (lane 6).
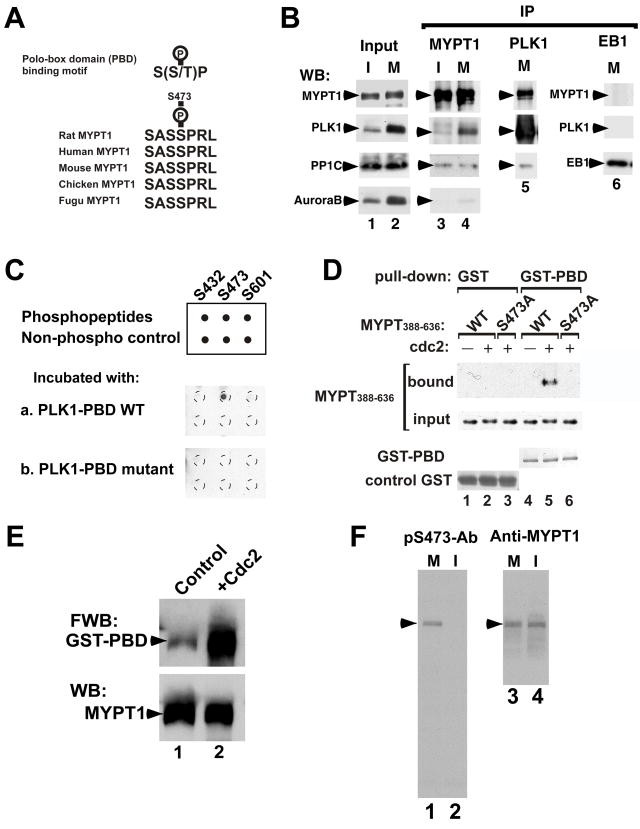
Phosphorylation-dependent association of MYPT1 and PLK1. A, Polo-box domain binding motif and sequence alignment of various vertebrate MYPT1s at Ser473. B, Co-immunoprecipitation of MYPT1 and PLK1. I, interphase (lanes 1 & 3); M, mitosis (lanes 2, 4, 5, 6). MYPT1 immunoprecipitates (IP) (lane 3 and 4) were blotted with anti-MYPT1, PLK1, PP1C and aurora B antibodies. PLK1 IP (lane 5) was blotted with anti-MYPT1, PP1C and PLK1 antibodies. EB1 immunoprecipitates (lane 6) as a negative control. C, Dot blot analyses. Binding of the GST-tagged PBD or mutant PBD to phosphopeptides was detected by an anti-GST antibody. D, GST pull-down assay. The central domain (388–636) of MYPT (lanes 4 & 5) and its mutant (Ser473A, lane 6) were phosphorylated with (lanes 5 & 6) or without (lane 4) cdc2, and their binding to GST-PBD was examined by Western blotting. Lanes 1–3, GST alone as a control. E, Far-Western ligand binding assay. Binding of GST-PBD to unphosphorylated, control MYPT1 (lane 1) and cdc2-phosphorylated MYPT1 (lane 2) was detected with a GST antibody (upper panel). Equal loading of MYPT1 was confirmed by Western blotting (lower panel). F, Western blot analyses of an antibody against S473-phosphorylated MYPT1 (pS473-Ab, lanes 1 & 2). M, mitosis; I, interphase. Lanes 3 & 4, Western blot with a general MYPT1 antibody.
We extended these findings by examining whether MYPT1 directly binds to PLK1 in a phosphorylation-dependent manner. First, dot blot analyses were performed to determine which phosphorylation site is critical for binding to the PBD of PLK1. Three phosphopeptides containing pSer432, pSer473 or pSer601, as well as the corresponding unphosphorylated peptides, were incubated with the GST-tagged PBD, and the binding of PBD was detected by Western blotting. As Fig. 2C shows, only the peptide containing phosphorylated Ser473 bound to PBD (Fig. 2C, a). A mutant (K540M/H538A) of GST-PBD that shows no binding to phosphopeptides (Elia et al., 2003a), on the other hand, did not bind to any of the phosphopeptides (Fig. 2C, b). The failure of the binding of the Ser601-phosphorylated peptide to PBD indicates that the sequence of S-pS-P alone is not sufficient for the PBD binding. It is worthy of note that the Ser473 site is well conserved among vertebrate MYPT1s whereas Ser601 is specific to rat MYPT1.
Second, we performed GST pull-down assays to determine whether Ser473 is required for MYPT1 to bind to the PBD of PLK1 (Fig. 2D). The central domain of histagged, wild-type MYPT1 (MYTP388–636), as well as its mutant (S473A) with Ser473 replaced with Ala, was phosphorylated with cdc2, and then incubated with GST-PBD. Only wild type MYPT1 (lane 5), but not the mutant MYPT1 (lane 6), was able to bind to GST-PBD. Without phosphorylation by cdc2 kinase, wild-type MYPT1 did not bind to PBD (lane 4). GST alone did not bind to either wild-type or mutant MYTP388–636, regardless of phosphorylation. These results indicate that MYPT1 binds directly to PBD via Ser473-phosphorylation. Finally, the binding of phosphorylated, full-length MYPT1 with GST-PBD was confirmed by a Far-Western ligand binding assay (Fig. 2E). Phosphorylated MYPT1 showed much stronger binding to the PBD (lane 2) than did control, unphosphorylated MYPT1 (lane 1). Our identification of MYPT1 as an PLK1-binding partner is consistent with recent proteomic screening, in which MYPT1 has been identified as one of the mitotic PBD-interacting proteins (Lowery et al., 2007).
Co-localization of MYPT1 and PLK1 on the centrosomes and kinetochores of mitotic cells
Because Ser473 phosphorylation is critical for MYPT1-PLK1 association, and because Ser473 is the most conserved of the three sites among vertebrate MYPT1s, we generated an antibody against Ser473-phosphorylated MYPT1 (called here pS473-Ab) and examined the intracellular localization of phosphorylated MYPT1 during mitosis. The specificity of the pS473-Ab was verified by Western blot analyses of total cell lysates (Fig. 2F). The antibody reacted only with MYPT1 from mitotic cells (lane 1) but not from interphase cells (lane 2).
Interestingly, immunofluorescence microscopy with this phospho-specific antibody (Fig. 3A) revealed that phosphorylated MYPT1 was localized to the centrosomes, as well as to the mitotic spindle and kinetochores in a variety of cultured mitotic cells including HeLa, SW962, COS7 and PTK2 cells. During prophase in HeLa cells, the pS473-Ab stained the kinetochores (arrowhead in Fig. 3A-a) and centrosomes (arrow) while the prophase spindle was only weakly stained. Double staining with a PLK1 antibody showed that PLK1 and phosphorylated MYPT1 were co-localized on centrosomes, as well as on kinetochores. Co-localization of both proteins on centrosomes and kinetochores persisted through prometaphase (panel A, e–h), while staining of the mitotic spindle with pS473-Ab became stronger. During telophase (panel A, i–l), phosphorylated MYPT1 and PLK1 were partially co-localized, though the zone positive for PLK1 was much narrower than that for MYPT1. It should be noted that the pS473-Ab did not stain microtubules in interphase cells (data not shown). The localization of MYPT1 on the centrosomes, as well as the mitotic spindles and kinetochores, was confirmed by two different general antibodies against MYPT1, which react with the C-terminus and the N-terminus of MYPT1, respectively (data not shown). These observations not only confirmed our notion that these proteins associate with each other during mitosis, but also suggest a role(s) of phosphorylated MYPT1 in the regulation of mitotic spindle assembly.
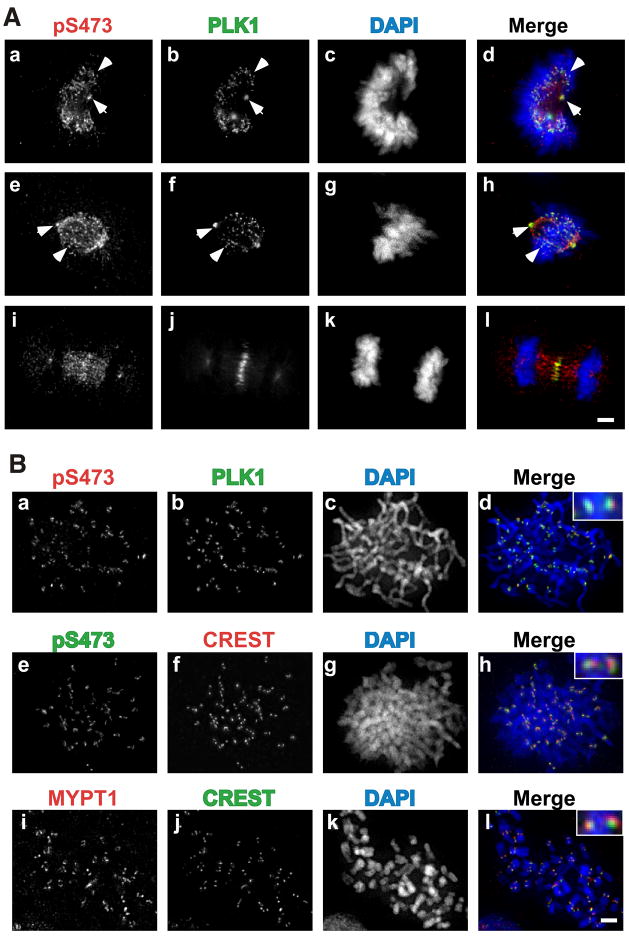
Immunofluorescent localization of S473-phosphorylated MYPT1 during mitosis. A, HeLa cells at prophase (a–d), prometaphase (e–h) or telophase (i–l) were stained with the anti-S473-phosphorylated MYPT1 antibody (pS473) and an anti-PLK1 antibody. Bar, 5μm. B, localization of phosphorylated MYPT1 at the kinetochores. Mitotic chromosome spreads were stained with pS473 and PLK1 antibodies (a–d) or pS473 and CREST antibodies (e–h) or MYPT1 and CREST antibodies (i–l).
The kinetochore localization of phosphorylated MYPT1 was more clearly seen with mitotic chromosome spreads (Fig. 3B). Double immunofluorescent staining with the pS473-Ab and PLK1 antibody (panel B, a–d) confirmed that phosphorylated MYPT1 (red) was co-localized with PLK1 (green) on the kinetochores. Double staining with a CREST antibody (panel B, e–h) revealed that both phosphorylated MYPT1 (green) and CREST (red) signals overlapped at the kinetochore with MYPT1 at the outside of CREST (see inset in “h”). Likewise, double labeling (panel B, i–l) with the rabbit monoclonal MYPT1 antibody (red) and the CREST antibody (green) confirmed the overlapping localization of these proteins at the kinetochores, again with MYPT1 at the outside of CREST (see the inset).
MYPT1 depletion rescues cells from mitotic arrest caused by reduction in PLK1 levels
PLK1 controls a number of mitotic events including centrosome maturation and separation, assembly of the mitotic spindle, chromosome segregation, and cytokinesis (Barr et al., 2004). The association of MYPT1 with PLK1 suggested the possibility that MYPT1/PP1C is involved in one or more of the PLK1’s mitotic functions. To test this hypothesis, we examined whether MYPT depletion by siRNA treatment affects mitosis, and how co-depletion of PLK1 and MYPT1 alters the phenotypes caused by PLK1 depletion. We found that double knockdown rescued, at least in part, the phenotypes caused by PLK1 knockdown.
Western blot analyses (Fig. 4A) indicate that single siRNA treatments for 48hr reduced the levels of MYPT1 and PLK1 in mitotic SW962 cells to 15% (lane 2 of Fig. A) and 8% (lane 3) of wild-type levels, respectively. As expected, double depletion reduced both proteins to similar levels (lane 4). Phase-contrast microscopy (Fig. 4B) of control (a), MYPT1-depleted (b), PLK1-depleted (c), and both MYPT1 and PLK1-depleted (d) SW962 cells revealed the following. First, PLK1 depletion vastly increased the number of mitotic rounded cells as reported previously (Liu and Erikson, 2002; Sumara et al., 2004; van Vugt et al., 2004). Second, MYPT1 depletion, though to a much lesser extent, also increased mitotic cell number, suggesting that MYPT1 is involved in mitotic progression. Third, most remarkably, double depletion rescued mitotic arrest caused by PLK1 depletion. Quantitative measurements of the mitotic index (Fig. 4C), obtained by nuclear staining with DAPI, confirmed the above notions. Whereas single depletion of MYPT1 or PLK1 increased the index from 3% (control) to 29%, or to 56% respectively, double depletion reduced the index to 19% (the difference between MYPT1 single depletion and double depletion is statistically significant as judged from a p value of <0.0001; indicated by an asterisk). Similar rescue by simultaneous depletion was observed with HeLa cells, indicating that the antagonistic relationship between PLK1 and MYPT1 is not cell-type specific.
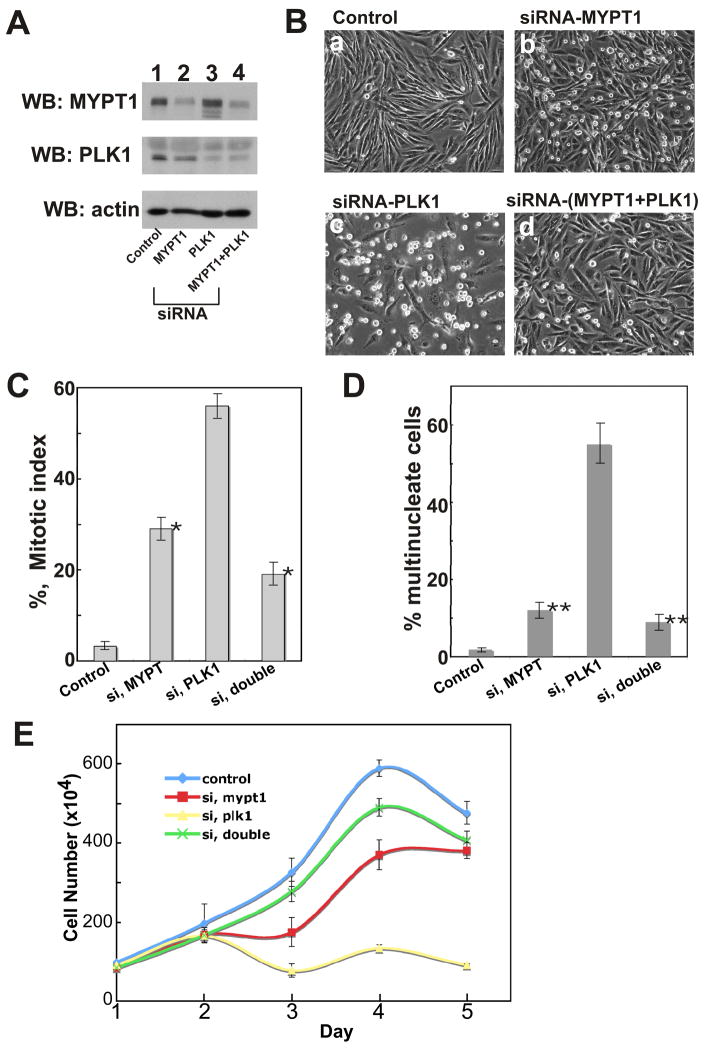
MYPT1 depletion rescues mitotic arrest caused by PLK1 depletion. A, Western blot analyses to detect levels of MYPT1 and PLK1 of control (lane 1), MYPT1-depleted (lane 2), PLK1-depleted (lane 3) or both MYPT1- and PLK1-depelted (lane 4) SW962 cells. B, Phase-contrast images of control (a), MYPT1-depleted (b), PLK1-depleted (c), and double-depleted (d) SW962 cells. C, Mitotic indexes of control, MYPT1-depleted, PLK1-depleted, double-depleted cells (200–300 cells were counted, repeated three times). D, Percentage of multinucleate cells in control, MYPT1-depleted, PLK1-depleted, and double-depleted cells (400–500 cells were counted, repeated three times). E, Cell proliferation analyses of control, MYPT1-depleted, PLK1-depleted, and double-depleted HeLa cells. HeLa cells were transfected at Day1 with siRNA. Note that about two-thirds of PLK1-depleted cells at Day2 were mitotically arrested. Error bars for C, D & E are standard deviation.
Simultaneous depletion of MYPT1 and PLK1 also reduced the percentage of multinucleate cells caused by PLK1 depletion. As Fig. 4D shows, while single depletion of PLK1 increased multinucleate cells from 2% (control) to 55%, the simultaneous depletion with MYPT1 greatly reduced multinucleate cells to 9%. MYPT depletion alone increased multinucleate cells to 12%, which is consistent with the proposed role of MYPT1 in cytokinesis (Kawano et al., 1999; Totsukawa et al., 1999). The difference in the number of multinucleate cells between double depletion (9%) and MYPT1 single depletion (12%), however, may not be statistically significant (p=0.065, double asterisk), suggesting that MYPT1 may have an additional function in cytokinesis independent of PLK1.
Cell proliferation analyses with HeLa cells supported the above results: as Fig. 4E shows, double-depleted cells proliferated almost as well as control cells, and better than MYPT1-depleted cells whereas cell growth of PLK1-depleted cell was completely blocked due to mitotic arrest.
Live cell imaging reveals that double-depleted cells exit from mitosis
To analyze in more detail how single or double depletion affects mitotic progression, we performed live-cell imaging of GFP-histone H2B in stably tranfected HeLa cells (Fig. 5A). Control cells typically underwent the metaphase-anaphase transition about 60min after chromosome condensation (top panel, video 1). In contrast, more than 80% (n=43) of PLK1-depleted mitotic cells after 24hr or 48hr treatment of siRNA did not enter into anaphase (second panel, video 2), even after prolonged observation (up to 4 hr). However, most double-depleted cells (78%, n=51) were able to exit from mitosis with a normal time course (third panel, video 3), confirming that double depletion rescued mitotic arrest caused by PLK1 depletion.
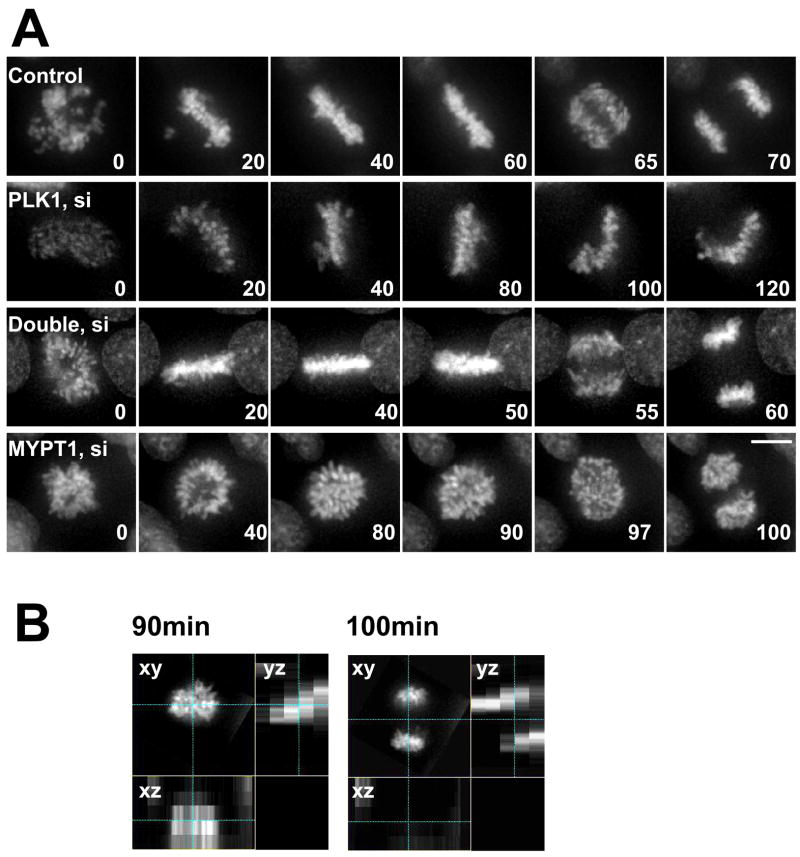
Live cell imaging of control, PLK1-depleted, double-depleted and MYPT1-depleted cells. A, HeLa cells stably expressing histone H2B-GFP were transfected with siRNAs to deplete PLK1, MYPT1 or both and imaged using a DeltaVision Image Restoration Microscope system. Five Z-section images were taken in 1min intervals, deconvoluted, and projected images were shown. Bar, 10μm. Time at minutes. B, orthogonal images of MYPT1-depleted cells at 90min and 100min.
Live-cell imaging revealed that MYPT1-depleted cells (Fig. 5A, bottom panel, video 4) were slightly delayed in the metaphase-anaphase transition (average time, 75min). Interestingly, chromatid segregation in most MYPT1-depleted cells (80%, n=35) occurred obliquely, which is easily discernible in the orthogonal views (yz plane, Fig. 5B) of the chromatids just before (90min) and after (100min) the metaphase-anaphase transition. The oblique mitotic spindle axis may be caused by an increased cortical tension due to myosin II activation because changes in cell shape and/or cortical tension have been reported to affect spindle positioning and assembly (O'Connell and Wang, 2000; Rosenblatt et al., 2004; Vasiliev et al., 2004). It is worthy of note that double depletion partially rescues oblique segregation. While 80% of MYPT1-depleted cells show oblique segregation, double depletion reduced this number to 30%. This suggests that PLK1 may also be involved in the oblique chromosome segregation, though the exact mechanism is currently unknown.
Double depletion of MYPT1 and PLK1 reinstates γ-tubulin at centrosomes and restores bipolar spindle assembly
PLK1 inactivation in mammalian cells has been shown to block the recruitment of γ-tubulin at centrosomes, resulting in monopolar spindle formation (Lane and Nigg, 1996; Sumara et al., 2004; van Vugt et al., 2004). We thus examined whether double depletion of MYPT1 and PLK1 restores γ-tubulin assembly at the centrosomes.
Fig. 6A shows localization of γ-tubulin and PLK1 in control (a–d), MYPT1-depleted (e–h), PLK1-depleted (i–l), and double-depleted (m–p) mitotic SW962 cells. Control cells showed co-localization of γ-tubulin (red) with PLK1 (green) at the centrosomes (Panel A, a–d). MYPT1 depletion blocked neither γ-tubulin accumulation nor PLK1 localization in the centrosomes (Panel A, e–h, n=91). The intensity ofγ-tubulin in each centrosome, as well as that of PLK1, appeared to be similar to those of control cells. The lack of effects was confirmed by double staining with the γ-tubulin (red) and MYPT1 (green) antibodies (Fig. 6B). The centrosomes of control cells (Panel B, a) are yellow, whereas the centrosomes of MYPT1-depleted cells are red (panel B, b). It is worthy of note that about 50% of MYPT1-depleted cells showed multiple (more than 3) γ-tubulin-containing foci (arrowhead in A-e and B-b, Fig. 6C). This may suggest an involvement of MYPT1 in centrosome assembly because the increase cannot be solely explained by a failure of cytokinesis. Multinucleate cells comprise only 23% of the population after MYPT1 depletion.
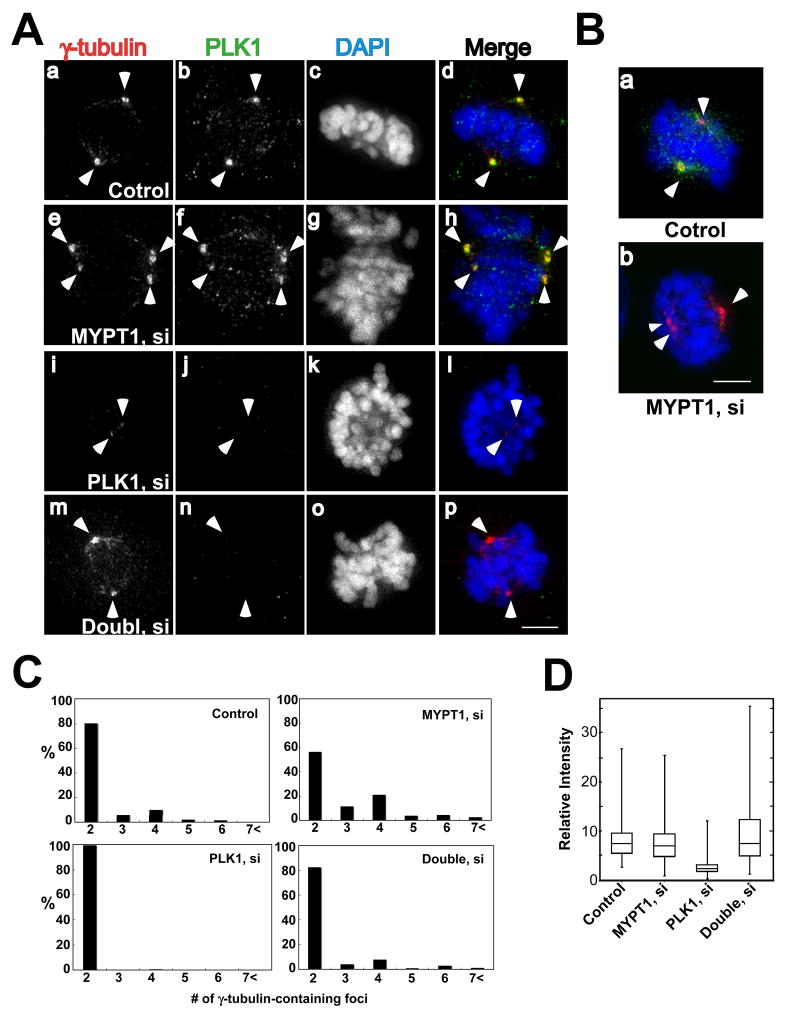
Double depletion of MYPT1 and PLK1 reinstates γ-tubulin at centrosomes. A, Localization of γ-tubulin (red), PLK1 (green) and DNA (blue) in control (a–d), MYPT1-depleted (e–h), PLK1-depleted (i–l), and double-depleted (m–p) SW962 cells. Arrowheads indicate centrosomes. Bar, 5μm. B, Localization of γ-tubulin (red), MYPT1 (green) and DNA (blue) in control (a) and MYPT1-depleted (b) cells. C, Distributions of the number of γ-tubulin-containing foci per cell for control, MYPT1-depleted, PLK1-depleted, and both MYPT1- and PLK1-depleted mitotic SW962 cells. D, Box plot of γ-tubulin intensities of individual centrosomes of control, MYPT1-depleted, PLK1-depleted, and double-depleted cells. The upper and lower edge of each box represent upper and lower quartilies, respectively. The median indicated by a horizontal line.
PLK1 depletion greatly reduced γ-tubulin staining at the centrosomes (i–l of Fig. 6A), and blocked separation of centrosomes in most (more than 90%, n=40) mitotic cells, the phenotypes of which are consistent with previous reports (Sumara et al., 2004; van Vugt et al., 2004). Double-depletion (Panel A, m–p) restored γ-tubulin accumulation in the centrosomes (n=60). The staining intensity of γ-tubulin in double-depleted cells (panel A, m) became as strong as that of control cells (panel A, a) where PLK1 depletion was confirmed by PLK1 antibody staining (compare n with b of panel A). It is worthy of note that double depletion suppressed the abnormal increase in the number of γ-tubulin-containing foci induced by MYPT1 single depletion (Fig. 6C), indicating that this MYPT1 phenotype depends upon PLK1.
Quantitative measurement confirmed the above conclusion. Fig. 6D shows a box plot illustrating the distributions of fluorescent intensities of individual centrosomes of control (n=99, centrosome number), MYPT1-depleted (n=214), PLK1-depleted (n=166), and double-depleted (n=210) cells. These plots indicate that the median γ-tubulin intensity per centrosome (indicated by a horizontal line in each box) was reduced by 75% after PLK1 depletion and that double depletion restored the γ-tubulin intensity to a level similar to that of control.
We further examined whether double depletion restores the assembly of the bipolar mitotic spindles (supplemental figure 1). Consistent with previous reports (Sumara et al., 2004; van Vugt et al., 2004), PLK1 depletion resulted in the assembly of monopolar mitotic spindles in more than 90% of PLK1-depleted, mitotic cells (n=125). Less than 10% of cells showed bipolar spindle assembly without focused centrosomes. In contrast, 76% of double-depleted mitotic cells (n=170) assembled either bipolar (48%) or multipolar (28%) spindles, indicating that the block of bipolar spindle assembly by PLK1 depletion is rescued by simultaneous depletion of MYPT1. Theses results, taken together, suggest that MYPT1/PP1C phosphatase antagonizes PLK1 during centrosome maturation, as well as during separation and bipolar spindle formation.
Depletion of MYPT1 increases phosphorylation of PLK1 at Thr210, an activation site for PLK1
If MYPT1/PP1C phosphatase indeed antagonizes PLK1, then phosphorylation of PLK1 substrates should be restored by double depletion. We found that this is the case for Cdc25c, a well-known PLK1 substrate that is essential for entry into mitosis. Cdc25c is known to exhibit a phosphorylation-dependent, mobility up-shift during mitosis (Ouyang et al., 1999). Fig 7A shows a Cdc25c Western blot of control (lane 3), MYPT1-depleted (lane 4), PLK1-depleted (lane 5) and double-depleted (lane 6) mitotic cells. PLK1 depletion decreased phosphorylation of Cdc25c as judged from the faster mobility of Cdc25c, confirming that Cdc25c is a substrate of PLK1. Double depletion, however, restores phosphorylation of Cdc25c, as seen by its mobility returning to a similar position as that of control cells.
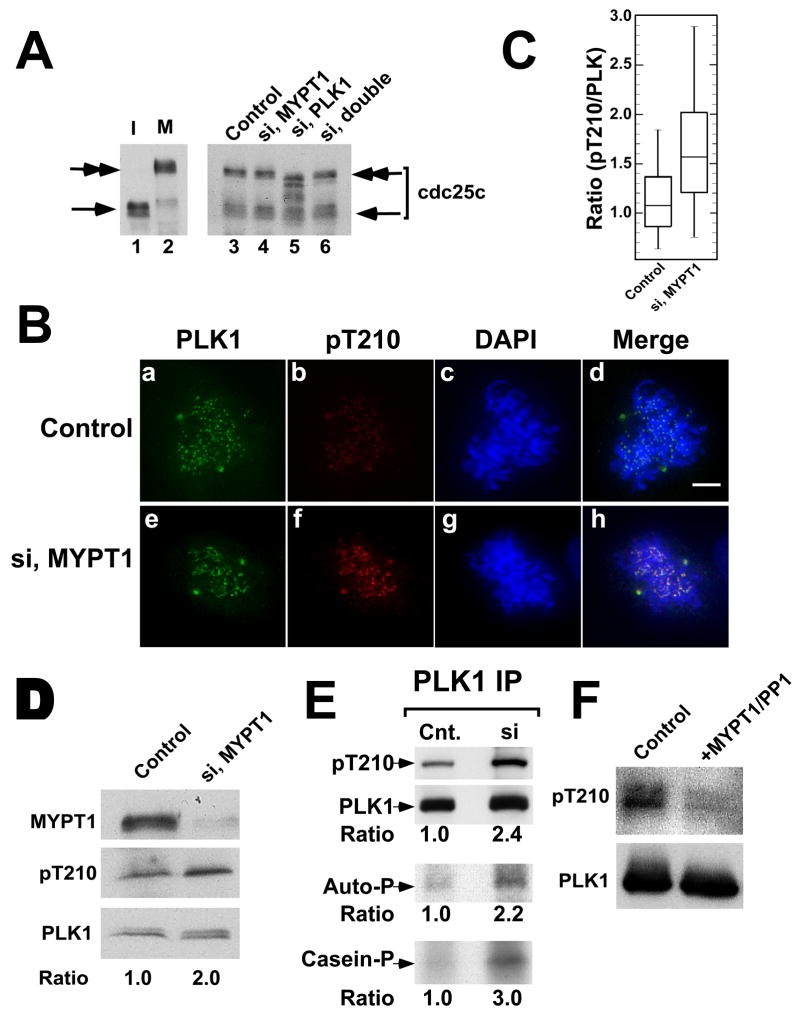
Restoration of Cdc25c phosphorylation by double depletion and increase in Thr210 phosphorylation by MYPT1 depletion. A, Cdc25c phosphorylation in control (lane 3), MYPT1-depleted (lane 4), PLK1-depleted (lane 5), and double-depleted (lane 6), mitotic cells was immunoblotted with the anti-Cdc25c antibody. The mobility of interphase Cdc25c is shown in lanes 1 for comparison with that of nocodazole arrested, mitotic Cdc25c (lane 2). B, double label immunofluorescence of control and MYPT1-depleted cells stained with anti-phospho-Thr210 (red) and anti-PLK (green) antibodies. C, Box-plot to reveal an increase in Thr210 phosphorylation by MYPT1 depletion. Ratios (pThr210/PLK1) of staining intensities on centrosomes (n=52 for control, n=88 for depleted cells) were analyzed. D, Western blot of total lysates from control and MYPT1-depleted cells. E, Western blot of PLK immunoprecipitates from control and MYPT1-depleted cells. Kinase activities were assayed by autophosphorylation of PLK1 (panel ‘Auto-P’) or phosphorylation of casein (panel “casein-P”). F, In vitro phosphatase assay by MYPT1/PP1. Baculovirus-expressed PLK1 (20ng) was incubated at 30oC for 20min without (lane 1) or with 8ng of MYPT1/PP1 (lane 2), and blotted with the anti-phosphoThr210 antibody.
How could MYPT1/PP1C antagonize PLK1? PLK1 is known to be activated by phosphorylation at its activating site, Thr210 (Jang et al., 2002; Kelm et al., 2002). It is thus possible that MYPT1/PP1C controls PLK1 activity by reducing phosphorylation at Thr210. By double labeling HeLa cells with a phospho-specific antibody against Thr210-phosphorylated PLK1 (pT210, red) and an anti-PLK antibody (green), we found that MYPT1 depletion raised Thr210 phosphorylation. As Fig. 7B shows, both centrosomes and kinetochores of MYPT1-depleted cells showed increased staining with the pT210 antibody (compare b and f of panel B). Quantitative analyses (Fig. 7C) of pT210/PLK1 fluorescent intensity ratios at the centrosomes (n=52 for control, n=88 for depleted cells) revealed that the mean ratio is increased from 1.1 to 1.7 while PLK1 expression levels were similar between control and MYPT1-depleted cells (see supplemental figure 2).
This was confirmed by immunoblot analyses of total cell lysates prepared from nocodazole-arrested, mitotic cells. As Fig. 7D shows, PLK1 of MYPT1-depleted cells exhibited a 2-fold increase in the reactivity against the pT210 antibody when compared with control. The increased phosphorylation of PLK1 was further verified by Western blot analyses of PLK1 immunoprecipitates (Fig. 7E). PLK1 from MYPT1-depleted cells showed 2.4 times higher phosphorylation than that of control cells. Consistent with the increased phosphorylation, the PLK immunoprecipitate from MYPT1-depleted cells showed a similar increase in kinase activity when their activities were measured by autophosphorylation (E, panel “Auto-P”) or casein phosphorylation (E, panel “casein-P”). Finally, we found that MYPT1/PP1 phosphatase is capable of dephosphorylating PLK1 at Thr210 in vitro (Fig. 7F). Taken together, these results indicate that MYPT1/PP1C antagonizes PLK1 by, at least in part, reducing Thr210 phosphorylation.
DISCUSSION
MYPT1 knockdown rescues mitotic arrest caused by PLK1 depletion and restores assembly of bipolar mitotic spindles
How could double depletion rescue the mitotic arrest? Our results indicate that MYPT1/PP1C antagonizes PLK1. It is likely that in double-depleted cells, the reduction of MYPT1/PP1C phosphatase activity can compensate for low PLK1 kinase activity by balancing phosphorylation levels of critical PLK1 targets. Indeed, we have observed that cdc25C phosphorylation is restored by double depletion. (Fig. 7A). There are two possible, though not mutually exclusive, mechanisms for the antagonism between MYPT1 and PLK1. One is that PLK1 and MYPT/PP1C share common substrates, thereby counteracting each other at the level of substrate phosphorylation. The other is that MYPT1/PP1C reduces phosphorylation at the activating site of PLK1, thus antagonizing PLK1. In the latter case, MYPT1/PP1C may directly dephosphorylate Thr210 or alternatively, MYPT1/PP1C could negatively regulate an upstream kinase that phosphorylates Thr210 of PLK1.
We have demonstrated that MYPT1 depletion increased both Thr210 phosphorylation of PLK1 and its kinase activity by about 2-fold, suggesting that the latter mechanism explains, at least in part, the rescue. While this increase seems to be modest, it could be sufficient for the rescue of the mitotic arrest caused by PLK1 depletion because as little as 5% of PLK1 activity can promote mitotic progression in certain cells (Liu et al., 2006). It is also quite possible that both mechanisms work simultaneously, further compensating for the loss of PLK1.
MYPT1/PP1C confers a new regulatory pathway of PLK1
MYPT1/PP1C phosphatase activity is itself controlled by phosphorylation by a number of kinases, including ZIP-like kinase, Rho kinase (ROCK), PAK (Hartshorne et al., 2004) and Aurora B (GT & FM. unpublished result). Such upstream kinases would thus constitute a network for the spatiotemporal regulation of PLK1 during cell division. It is possible, for example, that Rho-kinase, which has been reported to be present in centrosomes (Chevrier et al., 2002), could locally phosphorylate and inhibit MYPT1/PP1 activity at the centrosome, thereby activating PLK1 at the centrosomes. Likewise, Aurora B might phosphorylate MYPT1/PP1C at the kinetochore before the metaphase-anaphase transition and/or at the central spindle during cytokinesis. Such phosphorylation events, if any, could result in activation of PLK1 in these two locations where PLK activity has been shown to be critical for the tension-sensing mechanism (Ahonen et al., 2005; Wong and Fang, 2005) and for cytokinesis, respectively (Burkard et al., 2007; Carmena et al., 1998; Petronczki et al., 2007). Future work will be directed toward elucidating how MYPT1-PLK1 association contributes to the spatiotemporal regulation of PLK1 during the course of cell division.
Roles of vertebrate MYPT1s in cell proliferation and cancer
It is worthy of note that the phosphorylation site of MYPT1 responsible for PLK1 binding is well conserved among vertebrates including fish, but not conserved with invertebrate MYPT orthologues such as those of Drosophila and C. elegans. This lack of conservation may be reflected in differences in the severity of phenotypes caused by MYPT mutations. Drosophila MYPT mutants show defects in late embryogenesis including a failure of dorsal closure due to a migration defect in an ectoderm cell sheet but apparently display no defects in mitosis (Mizuno et al., 2002; Tan et al., 2003). Likewise, MYPT mutations in C. elegans mainly cause defects in morphogenesis. In addition, cytokinesis failure, though mild, is reported (Piekny and Mains, 2002) but without apparent defects in mitosis. In sharp contrast, mice lacking MYPT1 die at a very early stage of development before 7.5dpc and no MYPT1 null cells have been so far isolated (Okamoto et al., 2005). These differences in the phenotypes suggest that, during evolution vertebrate MYPT1 gained a new function critical for mitosis and cell proliferation. Such a notion is consistent with our finding that vertebrate MYPT1 participates in the PLK1-mediated pathways to control a variety of mitotic events.
The antagonistic relationship between MYPT1 and PLK1 suggests that the expression of both proteins must be tightly controlled for proper mitotic progression. In fact, both up-regulation and down-regulation of PLK1 are known to be detrimental for cell cycle progression (Barr et al., 2004; Blagden and Glover, 2003; Dai and Cogswell, 2003). Likewise, up- and down-regulation of MYPT1 appear toxic: Overexpression of a GFP-MYPT1 construct induces apoptosis (Wu et al., 2005) and MYPT1 knockout mice are embryonic lethal (Okamoto et al., 2005). Furthermore, PLK1 is known to be upregulated in many cancers (Eckerdt et al., 2005). Curiously, some cancer cells show downregulation of PLK1 (Simizu and Osada, 2000). It would be interesting to examine whether MYPT1 is downregulated in such cells to compensate for downregulated PLK1.
EXPERIMENTAL PROCEDURES
Cell culture and antibodies
SW962 human vulva carcinoma (HTB-118, ATCC, Manassas, VA), HeLa cells, and COS7 cells were maintained in Dulbecco’s modified Eagle’s medium (DME) containing 10 % fetal calf serum.
The following antibodies were used: rabbit polyclonal antibodies against MYPT1 (Totsukawa et al., 2000); a rabbit monoclonal against MYPT1/2 (Epitomics, Burlingame, CA); a human polyclonal against CREST (kindly provided by Dr. Brinkley, Univ. of Texas); a monoclonal against PLK1 (Zymed Laboratories, South San Francisco, CA); a rabbit polyclonal against PLK1 (Bethyl, Montogomery, TX); a monoclonal against alpha-tubulin (GE Healthcare Life Sciences, Arlington Heights, IL); a polyclonal against GST (Molecular Probes, Eugene, OR); a monoclonal against polyhistidine; a monoclonal against γ-tubulin (Sigma, Saint Louis, MO); a monoclonal against AuroraB (BD Biosciences, San Diego, CA); a monoclonal against PP1C (BD Biosciences) and a rabbit polyclonal (C-20) against Cdc25c (Santa Cruz Biotechnology, Santa Cruz, CA). A mouse monoclonal antibody (clone K50–483) against Thr210-phosphorylated PLK1 was from BD Biosciences (San Jose, CA), the specificity of which was confirmed by the following observations. The antibody reactivity was increased when PLK1 is phosphorylated at Thr210 by protein kinase A (Kelm et al., 2002) and was lost when PLK1 is dephosphorylated by λ-phosphatase treatment (see supplemental figure 3A). Furthermore, immunofluorescent staining of centrosomes and kinetochores with this antibody is diminished by depletion of PLK1 (supplemental figure 3B).
An antibody against Ser473-phosphorylated MYPT (pS473-Ab) was raised in mice using the sequence of a KLH-conjugated phosphopeptide of CGVIRSASS*PRLSSS (where S* indicates phosphoserine). The antibody was affinity-purified with phosphopeptide-conjugated agarose gel via SulfoLink coupling (Pierce, Rockford, IL), following absorbing with a corresponding non-phosphorylated peptide.
immunofluorescence
Immunofluorescence was performed as described (Totsukawa et al., 2000). For staining with the pS473-Ab, cells were first permeabilized with 0.2% Triton X-100 in the PHEM buffer (54mM Pipes, 22.5mM Hepes, 10mM EGTA and 8mM MgSO4, pH 7.0) with 100nM microcystin-LR (EMD Biosciences, San Diego, CA) for 1 min at 37oC. After fixation with 3.7% formaldehyde in PHEM for 10 min, cells were permeabilized again with 0.2% TritonX-100 for 5min, blocked with normal goat serum (Jackson laboratory, Bar Harbor, ME), and labeled with the pS473-Ab. Similar results were obtained when cells were fixed without prior permeabilization. Chromosomes were detected by DAPI staining (Molecular Probes). In the case of SW962 cells, mitotic cells were obtained by the shake-off method, cytospun onto poly-D-lysine coated coverslips, and analyzed by immunofluorescence as described above. For quantitative analyses of phosphoThr210 staining, cells were first fixed with 3.7% formaldehyde, permeabilized with acetone at 20oC, and processed as above. Images were taken as Z-stacks with a DeltaVision Image Restoration Microscope system (Applied Precision Instrument, LLC Issaquah, WA), deconvoluted and processed with the SoftWoRx software (Applied Precision Instruments). Exposure times and settings for deconvolution were constant for all samples to be compared within any given experiment. Image contrast and brightness were adjusted by Photoshop (Adobe, San Jose, CA).
siRNA
An siRNA duplex (5’-AGUACUCAACCAUAAUUAAdTdT-3’) targeting human MYPT1 was used at a concentration of 20nM. Similar results were obtained with two other siRNA duplexes (5’-GCGCCAGAAGACCAAGGUGdTdT-3’, 5’-GCUAUGAUGUUAAUAUUAAdTdT-3’), confirming the specificity of MYPT depletion phenotypes. PLK1 depletion was performed using 5nM of an siRNA duplex (5’-GAUCACCCUCCUUAAAUAUdTdT-3’), as published previously (Spankuch-Schmitt et al., 2002). siRNA transfection was performed using siLentFect (BioRad, Hercules, CA) reagents according to the manufacturers’ instructions. Single-strand, sense RNA was used for a mock control.
Phosphorylation, phosphopeptide analyses and immunoprecipitation
Mitotic (CSF-arrested) extracts prepared from Xenopus eggs were used for phosphorylation of MYPT1 and its mutants, as described (Totsukawa et al., 1999). Phosphorylation of MYPT was also performed with immunoprecipitated cdc2 kinase as described (Yamakita et al., 1994). Phosphopeptide mapping experiments were as described (Totsukawa et al., 1999). Immunoprecipitation was performed as described (Totsukawa et al., 1999).
Identification of the mitosis-specific phosphorylation sites
Mitosis-specific phosphorylation of rat MYPT1 yields 5 phospho-serine-containing peptide spots on a phosphopeptide map (M1–M5, see Fig. 1C-a) (Totsukawa et al., 1999). M5 is most abundant while M4 is a minor spot. The same phosphopeptide spots except M4 were observed by phosphorylation of MYPT1 with Xenopus mitotic extracts.
A series of truncation mutants of rat MYPT1 were phosphorylated with Xenopus mitotic extracts, and analyzed by phosphopeptide mapping to determine whether truncation eliminates mitosis-specific spot. Such analyses narrowed down the site of M1 between residue 460 and 499, and M5 between 583 and 622. Similar analyses with synthetic peptides covering the above two regions further narrowed M5 between 470 and 483 and M1 between 596 and 612. Point mutations at all possible Ser sites within these regions revealed that the mutation of Ser473 with Ala eliminated the M5 spot whereas M1 spot disappeared by the replacement of Ser601 with Ala.
Because both M1 and M5 show the Ser-Pro motif, we re-evaluated the M2/M3 sites, which were tentatively identified to be either Ser435 and/or Ser432 (Totsukawa et al., 1999). The Ser432 site has the Ser-Pro motif whereas the Ser435 does not. The M2/3 spots disappeared by the replacement of Ser432 with Ala, but not by the replacement of Ser435 with Ala, indicating that all three sites have the Ser-Pro motif.
Kinase assay
Kinase activity of immunoprecipitated PLK1 was assayed in a total volume of 30μl in a buffer containing 50mM Tris-HCl, 10mM MgCl2, 0.2mM DTT, 0.03% Brij35, 0.1mM ATP and 0.1μCi/μl of γ32P-ATP with or without 0.03μg/μl of casein, and reactions were performed at 30oC for 10min. After SDS-PAGE electrophoresis, gels were cut half: one containing PLK1 was processed for Western blot analyses with the anti-phosphoT210 antibody, followed by the pan PLK1 antibody. To detect autophosphorylation of PLK1, membranes were further processed for autoradiography. The other half of SDS gels containing casein was processed for autoradiography. Alternatively, the incorporation of phosphate into casein was measured by Cherenkov counting.
Baculovirus expression
Rat1 isoform of MYPT1 and human PP1Cβ were cloned into a His-tagged, Baculovirus expression vector (pDEST10, Invitrogen, Carlsbad, CA) and a non-tagged Baculovirus vector (pDEST8, Invitrogen), respectively. MYPT1/PP1C complex was purified after co-infection of Sf9 cells with baculovirus expressing MYPT1 and PP1Cβ . Baculovirus expressing full-length PLK1 was described previously (Golsteyn et al., 1995). Baculovirus expressing full-length GST-PLK1 was kindly provided by Dr. R. Erikson.
MYPT1-PLK1 Binding assay
His-tagged, wild-type MYPT1388–636, as well as its single point mutant (S473A), was phosphorylated with immobilized cdc2 kinase. After removing cdc2 kinase, both samples (1 μg) were incubated for 1 hr at 4°C in an IP buffer (50 μl) with GST-PBD (10μg). Glutathione beads were then added to recover GST-PBD, washed extensively with the IP buffer, and bound MYPT1 was detected by the anti-polyhistidine antibody. Peptide dot blot assays for PBD binding were performed as described (Elia et al., 2003b). Far Western ligand binding assay was performed as described (Neef et al., 2003).
Supplementary Material
01
02
03
04
05
Acknowledgments
We thank Dr. M. Yaffe for GST-PBD constructs, Dr. Erich E. Nigg for a baculovirus construct of PLK1, Dr. R. Erickson for baculovirus constructs of GST-PLK1, Dr. B. R. Brinkley for a CREST antibody, Dr. G.M. Wahl for HeLa cells stably expressing histone H2B-GFP, and Dr. Barth Grant for his critical reading. We also thank Dan Lee for his help on data collection for Fig. 7B. This work was supported by the NCI grant, CA42742 (FM and SY) and HL 23615 (to DJH).
Footnotes
Publisher's Disclaimer: This is a PDF file of an unedited manuscript that has been accepted for publication. As a service to our customers we are providing this early version of the manuscript. The manuscript will undergo copyediting, typesetting, and review of the resulting proof before it is published in its final citable form. Please note that during the production process errors may be discovered which could affect the content, and all legal disclaimers that apply to the journal pertain.
References
- Ahonen LJ, Kallio MJ, Daum JR, Bolton M, Manke IA, Yaffe MB, Stukenberg PT, Gorbsky GJ. Polo-like kinase 1 creates the tension-sensing 3F3/2 phosphoepitope and modulates the association of spindle-checkpoint proteins at kinetochores. Curr Biol. 2005;15:1078–1089. [Abstract] [Google Scholar]
- Axton JM, Dombradi V, Cohen PT, Glover DM. One of the protein phosphatase 1 isoenzymes in Drosophila is essential for mitosis. Cell. 1990;63:33–46. [Abstract] [Google Scholar]
- Barr FA, Sillje HH, Nigg EA. Polo-like kinases and the orchestration of cell division. Nat Rev Mol Cell Biol. 2004;5:429–440. [Abstract] [Google Scholar]
- Blagden SP, Glover DM. Polar expeditions--provisioning the centrosome for mitosis. Nat Cell Biol. 2003;5:505–511. [Abstract] [Google Scholar]
- Bloecher A, Tatchell K. Defects in Saccharomyces cerevisiae protein phosphatase type I activate the spindle/kinetochore checkpoint. Genes Dev. 1999;13:517–522. [Europe PMC free article] [Abstract] [Google Scholar]
- Burkard ME, Randall CL, Larochelle S, Zhang C, Shokat KM, Fisher RP, Jallepalli PV. Chemical genetics reveals the requirement for Polo-like kinase 1 activity in positioning RhoA and triggering cytokinesis in human cells. Proc Natl Acad Sci U S A. 2007;104:4383–4388. [Europe PMC free article] [Abstract] [Google Scholar]
- Carmena M, Riparbelli MG, Minestrini G, Tavares AM, Adams R, Callaini G, Glover DM. Drosophila polo kinase is required for cytokinesis. J Cell Biol. 1998;143:659–671. [Europe PMC free article] [Abstract] [Google Scholar]
- Ceulemans H, Bollen M. Functional diversity of protein phosphatase-1, a cellular economizer and reset button. Physiol Rev. 2004;84:1–39. [Abstract] [Google Scholar]
- Cheng A, Dean NM, Honkanen RE. Serine/threonine protein phosphatase type 1gamma1 is required for the completion of cytokinesis in human A549 lung carcinoma cells. J Biol Chem. 2000;275:1846–1854. [Abstract] [Google Scholar]
- Chevrier V, Piel M, Collomb N, Saoudi Y, Frank R, Paintrand M, Narumiya S, Bornens M, Job D. The Rho-associated protein kinase p160ROCK is required for centrosome positioning. J Cell Biol. 2002;157:807–817. [Europe PMC free article] [Abstract] [Google Scholar]
- Cohen PTW. Protein phosphatase 1 - targeted in many directions. J Cell Sci. 2002;115:241–256. [Abstract] [Google Scholar]
- Dai W, Cogswell JP. Polo-like kinases and the microtubule organization center: targets for cancer therapies. Prog Cell Cycle Res. 2003;5:327–334. [Abstract] [Google Scholar]
- Doonan JH, Morris NR. The bimG gene of Aspergillus nidulans, required for completion of anaphase, encodes a homolog of mammalian phosphoprotein phosphatase 1. Cell. 1989;57:987–996. [Abstract] [Google Scholar]
- Eckerdt F, Yuan J, Strebhardt K. Polo-like kinases and oncogenesis. Oncogene. 2005;24:267–276. [Abstract] [Google Scholar]
- Elia AE, Cantley LC, Yaffe MB. Proteomic screen finds pSer/pThr-binding domain localizing Plk1 to mitotic substrates. Science. 2003a;299:1228–1231. [Abstract] [Google Scholar]
- Elia AE, Rellos P, Haire LF, Chao JW, Ivins FJ, Hoepker K, Mohammad D, Cantley LC, Smerdon SJ, Yaffe MB. The molecular basis for phosphodependent substrate targeting and regulation of Plks by the Polo-box domain. Cell. 2003b;115:83–95. [Abstract] [Google Scholar]
- Fernandez A, Brautigan DL, Lamb NJ. Protein phosphatase type 1 in mammalian cell mitosis: chromosomal localization and involvement in mitotic exit. J Cell Biol. 1992;116:1421–1430. [Europe PMC free article] [Abstract] [Google Scholar]
- Golsteyn RM, Mundt KE, Fry AM, Nigg EA. Cell cycle regulation of the activity and subcellular localization of Plk1, a human protein kinase implicated in mitotic spindle function. J Cell Biol. 1995;129:1617–1628. [Europe PMC free article] [Abstract] [Google Scholar]
- Hartshorne DJ, Ito M, Erdodi F. Role of protein phosphatase type 1 in contractile functions: myosin phosphatase. J Biol Chem. 2004;279:37211–37214. [Abstract] [Google Scholar]
- Jang YJ, Ma S, Terada Y, Erikson RL. Phosphorylation of threonine 210 and the role of serine 137 in the regulation of mammalian polo-like kinase. J Biol Chem. 2002;277:44115–44120. [Abstract] [Google Scholar]
- Kawano Y, Fukata Y, Oshiro N, Amano M, Nakamura T, Ito M, Matsumura F, Inagaki M, Kaibuchi K. Phosphorylation of myosin-binding subunit (MBS) of myosin phosphatase by Rho-kinase in vivo. J Cell Biol. 1999;147:1023–1038. [Europe PMC free article] [Abstract] [Google Scholar]
- Kelm O, Wind M, Lehmann WD, Nigg EA. Cell cycle-regulated phosphorylation of the Xenopus polo-like kinase Plx1. J Biol Chem. 2002;277:25247–25256. [Abstract] [Google Scholar]
- Lane HA, Nigg EA. Antibody microinjection reveals an essential role for human polo-like kinase 1 (Plk1) in the functional maturation of mitotic centrosomes. J Cell Biol. 1996;135:1701–1713. [Europe PMC free article] [Abstract] [Google Scholar]
- Liu X, Erikson RL. Activation of Cdc2/cyclin B and inhibition of centrosome amplification in cells depleted of Plk1 by siRNA. Proc Natl Acad Sci U S A. 2002;99:8672–8676. [Europe PMC free article] [Abstract] [Google Scholar]
- Liu X, Lei M, Erikson RL. Normal cells, but not cancer cells, survive severe Plk1 depletion. Mol Cell Biol. 2006;26:2093–2108. [Europe PMC free article] [Abstract] [Google Scholar]
- Lowery DM, Clauser KR, Hjerrild M, Lim D, Alexander J, Kishi K, Ong SE, Gammeltoft S, Carr SA, Yaffe MB. Proteomic screen defines the Polo-box domain interactome and identifies Rock2 as a Plk1 substrate. Embo J. 2007;26:2262–2273. [Europe PMC free article] [Abstract] [Google Scholar]
- Matsumura F, Hartshorne DJ. Myosin phosphatase targeting subunit: Many roles in cell function. Biochem Biophys Res Commun. 2008 In press. [Europe PMC free article] [Abstract] [Google Scholar]
- Mizuno T, Tsutsui K, Nishida Y. Drosophila myosin phosphatase and its role in dorsal closure. Development. 2002;129:1215–1223. [Abstract] [Google Scholar]
- Neef R, Preisinger C, Sutcliffe J, Kopajtich R, Nigg EA, Mayer TU, Barr FA. Phosphorylation of mitotic kinesin-like protein 2 by polo-like kinase 1 is required for cytokinesis. J Cell Biol. 2003;162:863–875. [Europe PMC free article] [Abstract] [Google Scholar]
- O'Connell CB, Wang YL. Mammalian spindle orientation and position respond to changes in cell shape in a dynein-dependent fashion. Mol Biol Cell. 2000;11:1765–1774. [Europe PMC free article] [Abstract] [Google Scholar]
- Ohkura H, Kinoshita N, Miyatani S, Toda T, Yanagida M. The fission yeast dis2+ gene required for chromosome disjoining encodes one of two putative type 1 protein phosphatases. Cell. 1989;57:997–1007. [Abstract] [Google Scholar]
- Okamoto R, Ito M, Suzuki N, Kongo M, Moriki N, Saito H, Tsumura H, Imanaka-Yoshida K, Kimura K, Mizoguchi A, et al. The targeted disruption of the MYPT1 gene results in embryonic lethality. Transgenic Res. 2005;14:337–340. [Abstract] [Google Scholar]
- Ouyang B, Li W, Pan H, Meadows J, Hoffmann I, Dai W. The physical association and phosphorylation of Cdc25C protein phosphatase by Prk. Oncogene. 1999;18:6029–6036. [Abstract] [Google Scholar]
- Petronczki M, Glotzer M, Kraut N, Peters JM. Polo-like kinase 1 triggers the initiation of cytokinesis in human cells by promoting recruitment of the RhoGEF Ect2 to the central spindle. Dev Cell. 2007;12:713–725. [Abstract] [Google Scholar]
- Piekny AJ, Mains PE. Rho-binding kinase (LET-502) and myosin phosphatase (MEL-11) regulate cytokinesis in the early Caenorhabditis elegans embryo. J Cell Sci. 2002;115:2271–2282. [Abstract] [Google Scholar]
- Rosenblatt J, Cramer LP, Baum B, McGee KM. Myosin II-dependent cortical movement is required for centrosome separation and positioning during mitotic spindle assembly. Cell. 2004;117:361–372. [Abstract] [Google Scholar]
- Simizu S, Osada H. Mutations in the Plk gene lead to instability of Plk protein in human tumour cell lines. Nat Cell Biol. 2000;2:852–854. [Abstract] [Google Scholar]
- Spankuch-Schmitt B, Bereiter-Hahn J, Kaufmann M, Strebhardt K. Effect of RNA silencing of polo-like kinase-1 (PLK1) on apoptosis and spindle formation in human cancer cells. J Natl Cancer Inst. 2002;94:1863–1877. [Abstract] [Google Scholar]
- Sumara I, Gimenez-Abian JF, Gerlich D, Hirota T, Kraft C, De La Torre C, Ellenberg J, Peters JM. Roles of Polo-like Kinase 1 in the Assembly of Functional Mitotic Spindles. Curr Biol. 2004;14:1712–1722. [Abstract] [Google Scholar]
- Tan C, Stronach B, Perrimon N. Roles of myosin phosphatase during Drosophila development. Development. 2003;130:671–681. [Abstract] [Google Scholar]
- Totsukawa G, Yamakita Y, Yamashiro S, Hartshorne DJ, Sasaki Y, Matsumura F. Distinct roles of ROCK (Rho-kinase) and MLCK in spatial regulation of MLC phosphorylation for assembly of stress fibers and focal adhesions in 3T3 fibroblasts. J Cell Biol. 2000;150:797–806. [Europe PMC free article] [Abstract] [Google Scholar]
- Totsukawa G, Yamakita Y, Yamashiro S, Hosoya H, Hartshorne DJ, Matsumura F. Activation of myosin phosphatase targeting subunit by mitosis-specific phosphorylation. J Cell Biol. 1999;144:735–744. [Europe PMC free article] [Abstract] [Google Scholar]
- Trinkle-Mulcahy L, Andersen J, Lam YW, Moorhead G, Mann M, Lamond AI. Repo-Man recruits PP1 gamma to chromatin and is essential for cell viability. J Cell Biol. 2006;172:679–692. [Europe PMC free article] [Abstract] [Google Scholar]
- Vagnarelli P, Hudson DF, Ribeiro SA, Trinkle-Mulcahy L, Spence JM, Lai F, Farr CJ, Lamond AI, Earnshaw WC. Condensin and Repo-Man-PP1 co-operate in the regulation of chromosome architecture during mitosis. Nat Cell Biol. 2006;8:1133–1142. [Europe PMC free article] [Abstract] [Google Scholar]
- van Vugt MA, van de Weerdt BC, Vader G, Janssen H, Calafat J, Klompmaker R, Wolthuis RM, Medema RH. Polo-like kinase-1 is required for bipolar spindle formation but is dispensable for anaphase promoting complex/Cdc20 activation and initiation of cytokinesis. J Biol Chem. 2004;279:36841–36854. [Abstract] [Google Scholar]
- Vasiliev JM, Omelchenko T, Gelfand IM, Feder HH, Bonder EM. Rho overexpression leads to mitosis-associated detachment of cells from epithelial sheets: a link to the mechanism of cancer dissemination. Proc Natl Acad Sci U S A. 2004;101:12526–12530. [Europe PMC free article] [Abstract] [Google Scholar]
- Wong OK, Fang G. Plx1 is the 3F3/2 kinase responsible for targeting spindle checkpoint proteins to kinetochores. J Cell Biol. 2005;170:709–719. [Europe PMC free article] [Abstract] [Google Scholar]
- Wu Y, Muranyi A, Erdodi F, Hartshorne DJ. Localization of Myosin phosphatase target subunit and its mutants. J Muscle Res Cell Motil. 2005;26:123–134. [Abstract] [Google Scholar]
- Yamakita Y, Yamashiro S, Matsumura F. In vivo phosphorylation of regulatory light chain of myosin II during mitosis of cultured cells. J Cell Biol. 1994;124:129–137. [Europe PMC free article] [Abstract] [Google Scholar]
Full text links
Read article at publisher's site: https://doi.org/10.1016/j.devcel.2008.02.013
Read article for free, from open access legal sources, via Unpaywall:
http://www.cell.com/article/S153458070800107X/pdf
Citations & impact
Impact metrics
Citations of article over time
Alternative metrics
Smart citations by scite.ai
Explore citation contexts and check if this article has been
supported or disputed.
https://scite.ai/reports/10.1016/j.devcel.2008.02.013
Article citations
Insights into the structural and functional activities of forgotten Kinases: PCTAIREs CDKs.
Mol Cancer, 23(1):135, 29 Jun 2024
Cited by: 0 articles | PMID: 38951876 | PMCID: PMC11218289
Review Free full text in Europe PMC
Apurinic/apyrimidinic endodeoxyribonuclease 1 (APE1) promotes stress granule formation via YBX1 phosphorylation in ovarian cancer.
Cell Mol Life Sci, 81(1):113, 04 Mar 2024
Cited by: 0 articles | PMID: 38436697 | PMCID: PMC10912283
Phosphatases modified by LH signaling in ovarian follicles: testing their role in regulating the NPR2 guanylyl cyclase†.
Biol Reprod, 110(1):102-115, 01 Jan 2024
Cited by: 3 articles | PMID: 37774352 | PMCID: PMC10790345
PLK1 and its substrate MISP facilitate intrahepatic cholangiocarcinoma progression by promoting lymphatic invasion and impairing E-cadherin adherens junctions.
Cancer Gene Ther, 31(2):322-333, 06 Dec 2023
Cited by: 2 articles | PMID: 38057358 | PMCID: PMC10874889
Identification of PP1c-PPP1R12A Substrates Using Kinase-Catalyzed Biotinylation to Identify Phosphatase Substrates.
ACS Omega, 8(39):35628-35637, 21 Sep 2023
Cited by: 0 articles | PMID: 37810667 | PMCID: PMC10552495
Go to all (115) article citations
Data
Data behind the article
This data has been text mined from the article, or deposited into data resources.
BioStudies: supplemental material and supporting data
Similar Articles
To arrive at the top five similar articles we use a word-weighted algorithm to compare words from the Title and Abstract of each citation.
Chk2-dependent phosphorylation of myosin phosphatase targeting subunit 1 (MYPT1) regulates centrosome maturation.
Cell Cycle, 18(20):2651-2659, 15 Aug 2019
Cited by: 7 articles | PMID: 31416392 | PMCID: PMC6773232
Chk1 modulates the interaction between myosin phosphatase targeting protein 1 (MYPT1) and protein phosphatase 1cβ (PP1cβ).
Cell Cycle, 17(4):421-427, 19 Mar 2018
Cited by: 7 articles | PMID: 29262732 | PMCID: PMC5927650
O-GlcNAcylation of myosin phosphatase targeting subunit 1 (MYPT1) dictates timely disjunction of centrosomes.
J Biol Chem, 295(21):7341-7349, 15 Apr 2020
Cited by: 11 articles | PMID: 32295844 | PMCID: PMC7247298
Regulating a key mitotic regulator, polo-like kinase 1 (PLK1).
Cytoskeleton (Hoboken), 75(11):481-494, 01 Nov 2018
Cited by: 57 articles | PMID: 30414309 | PMCID: PMC7113694
Review Free full text in Europe PMC
Funding
Funders who supported this work.
NCI NIH HHS (8)
Grant ID: R01 CA042742-21A1
Grant ID: R37 CA042742
Grant ID: CA42742
Grant ID: R01 CA042742-23
Grant ID: R37 CA042742-15
Grant ID: R01 CA042742-24
Grant ID: R01 CA042742
Grant ID: R01 CA042742-22
NHLBI NIH HHS (3)
Grant ID: R01 HL023615
Grant ID: R01 HL023615-29
Grant ID: HL23615
National Cancer Institute (2)
Grant ID: CA42742
Grant ID: HL23615