Abstract
Free full text

Irradiation alters selection for oncogenic mutations in hematopoietic progenitors
Summary
Exposure to ionizing radiation and other DNA damaging carcinogens is strongly associated with induction of malignancies. Prevailing paradigms attribute this association to the induction of oncogenic mutations, as the incidence of oncogenic events is thought to limit initiation and progression of cancers. On the other hand, random mutagenic and genotoxic effects of irradiation are likely to alter progenitor cell populations and the microenvironment, thus altering the selective effects of oncogenic mutations. Using competitive bone marrow transplantation experiments in mice, we demonstrate that ionizing irradiation leads to a persistent decline in the numbers and fitness of hematopoietic stem cells, in part resulting from persistent induction of reactive oxygen species. Previous irradiation dramatically alters the selective effects of some oncogenic mutations, substantially inhibiting clonal expansion and leukemogenesis driven by Bcr-Abl or activated N-Ras oncogenes, but enhancing the selection for and leukemogenesis driven by the activated Notch1 mutant, ICN. Irradiation-dependent selection for ICN expression occurs in a hematopoietic stem cell enriched pool, which should facilitate the accumulation of additional oncogenic events at a committed T-progenitor stage critical for formation of T-lymphocytic leukemia stem cells. Enhancement of ICN-driven selection and leukemogenesis by previous irradiation is in part non-cell autonomous, as partial restoration of normal hematopoiesis can reverse these effects of irradiation. These studies demonstrate that irradiation substantially alters the adaptive landscape in hematopoietic progenitors and suggest that the causal link between irradiation and carcinogenesis might involve increased selection for particular oncogenic mutations.
Introduction
Exposure to ionizing radiation is strongly associated with the induction of malignancies in humans and in animal models (1, 2). Since ionizing irradiation is a potent mutagen, and cancer development requires the acquisition of mutations in oncogenes and tumor suppressors, prevailing paradigms attribute cancer causation by DNA damaging carcinogens to the direct induction of oncogenic events by these agents (3, 4).
From an evolutionary perspective, to be able to trigger clonal expansion, an initiating oncogenic event will typically need to provide a mutated cell with a gain in fitness relative to other cells competing for the same niches. Therefore, the ability or inability of oncogenic mutations to cause clonal expansion should be dependent on the fitness of cells competing for niches with limited resources and on the state of the microenvironment (5, 6). While mutagenic actions of carcinogens, including γ-irradiation, might increase the frequency of oncogenic events, induction of mutations that reduce cellular fitness is a much more probable outcome of random mutations (7). In addition to the direct induction of mutations that reduce cellular fitness, DNA damage results in altered nuclear architecture which may also contribute to decreased cellular fitness (8). Moreover, irradiation has been shown to cause dramatic alterations in the cellular microenvironment (9), and alterations in the microenvironment are expected to significantly impact cellular fitness as well.
Several groups have proposed that growth inhibitory conditions can select for oncogenic mutations that provide resistance to the inhibitory context (10-15). More specifically, we have previously hypothesized that reductions of fitness caused by accumulated DNA damage should lead to selection for adaptive oncogenic mutations (6). Using the mouse hematopoietic system, we explored the effects of γ-irradiation on the fitness of progenitor cells, as well as the effects of prior irradiation on the selective effects conferred by different oncogenic mutations. Our results suggest that the causal link between irradiation and cancer involves changes in the adaptive landscape, which increase selection for particular oncogenic events.
Materials and Methods
Retroviral constructs and infections
MSCV-ires-GFP (MiG)-ICN (16), MiG-N-Ras12D, MiG-Akt (17) and MiG-p185-Bcr-Abl (18) were the generous gifts of Drs. Warren Pear, Yosef Refaeli and Zhonghan Dai, respectively. MiG-Myc was generated by cloning human c-myc cDNA into MiG. Viral particles were assembled using ψNX-Eco packaging cells as previously described (10). Freshly isolated BM cells were transduced with retrovirus-containing ψNX-Eco supernatants in non-adhesive 6-well plates using the spin-fection technique (centrifugation at 910g for 1.5 hours in the presence 8μg/ml polybrene).
Mice and irradiation
Balb/c mice were purchased from the National Cancer Institute. GFP transgenic mice (19) were backcrossed into the Balb/c background for >11 generations. BM transplant recipients were lethally irradiated with two 5 Gy doses separated by 3-4 hours using an X-ray source (RadSource RS2000 irradiator). Sublethal irradiation was at 5 Gy.
15-F2t-Isoprostane (8-iso-prostaglandin-F2α) analysis
15-F2t-Isoprostane concentrations in mouse plasma were measured using a validated LC/LC-MS/MS method (20). In mouse plasma, the range of reliable response of the assay was from 0.05 to 100ng/mL. Intra- and inter-day accuracies were between 91.1% and 106.2%, intra- and inter-day precisions were ≤ 7%. The absolute recovery of 15-F2t-isoprostane after protein precipitation in plasma was 100.2% ± 9.5%. Quantification was not affected by matrix interferences and there was no carry-over.
Flow cytometry
Antibody staining and flow cytometric analyses were performed as previously described (10). Detection of γH2A.X was performed as previously described (21) using monoclonal antibody from Upstate (clone JBW301).
Statistics
Statistical analyses were performed using Prism4 software from GraphPad. Asterisks in the figure legends represent p-values of 2-tailed t-test: *for p<0.05, **for p value <0.01, and ***for p<0.001. See Supplemental Text for absolute p-values.
Results
Irradiation permanently reduces the fitness of hematopoietic stem and progenitor cells
In order to study the impact of irradiation on the fitness of hematopoietic progenitors, we took advantage of the ability of competitive BM transplantation assays in mice to measure relative hematopoietic reconstitution by early progenitors. Balb/c mice were X-irradiated at 5 Gy and then allowed to recover for 3 months (Fig. 1A). Hematopoiesis appeared fully recovered by 6 weeks post-irradiation (Supplementary Fig. S1). BM was harvested from irradiated mice (or from unirradiated mice as controls), mixed 9:1 with BM from green fluorescent protein transgenic (GFP-Tg) mice, and then transplanted into lethally irradiated recipients. Two months later, BM from these recipient mice was analyzed for GFP expression in the myeloid (GR1+) and B-lymphoid (B220+) lineages (Fig. 1A). GFP expression was observed in the expected minor fraction of myeloid and B-lineage cells in recipients of control unirradiated BM mixed 9:1 with GFP-Tg BM. In contrast, GFP expression in these lineages approached 100% in recipients of BM from irradiated mice mixed with GFP-Tg BM. Note that a small percentage of GFPneg cells are detected even in control recipients receiving 100% GFP-Tg BM (“GFP” group), which could reflect the presence of residual host cells. Strikingly, the representation of GFP+ cells in the BM was indistinguishable between recipients of 90% irradiated BM plus 10% GFP-Tg BM and recipients of 100% GFP-Tg BM, indicating that irradiation causes severe reductions in fitness when measured in competitive assays. By performing similar transplantation experiments with BM from mice irradiated 1 week or 6 weeks prior, we demonstrated that a similar loss of fitness is evident at these timepoints (Supplementary Fig. S2,S3).
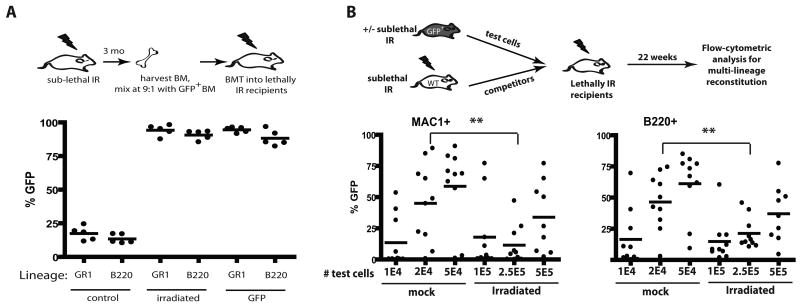
A) BM was isolated from Balb/c mice that had been irradiated 3 months before with 5 Gy (or not as the control), and then mixed with BM from non-irradiated GFP-Tg animals at 9:1 ratios. The mixes (or 100% GFP-Tg BM as the control) were then transplanted into lethally irradiated mice at 107 cells/recipient. At 2 month post-irradiation, peripheral blood was collected, stained with PE-Cy7-anti-GR1 plus PE-anti-B220 antibodies, and analyzed for the percentages of GFP+ cells in the indicated blood lineages by flow cytometry. For this and all other figures, each filled circle represents data from an individual mouse. B) BM was harvested from GFP-Tg mice that had either been irradiated 6 weeks before with 5 Gy or left untreated (the “test” cells). The indicated numbers of viable GFP+ test cells (eg. 1E4 denotes 1×104) were mixed with 106 viable competitor Balb/c (GFPneg) BM cells isolated from donors that were irradiated 6 weeks prior with 5 Gy. The mixes were injected into lethally irradiated Balb/c recipients. At 5.5 month post transplant, peripheral blood was stained with PE-Cy7-anti-Mac1 plus PE-anti-B220 antibodies, and the percentages of GFP+ cells in the MAC1+ and B220+ lineages are indicated for the 10 recipients/group.
Analysis of phenotypically defined HSC, specified by c-Kit+Sca1+LineagenegCD34negFlk2neg or CD150+CD48negLineageneg marker expression (22, 23), in the BM of mice irradiated 3 months prior at 5 Gy, showed no significant differences relative to control mice (Supplementary Fig. S4,S5B). We then determined the effects of irradiation on concentrations of functional HSC using limiting dilution analysis. For these experiments, the “test cells” are BM cells from GFP-Tg mice irradiated or not 8 weeks prior. Different numbers of test BM cells where mixed with set numbers of competitor BM cells, and then transplanted into lethally irradiated recipients (Fig. 1B). Since non-irradiated competitors would mask contributions from irradiated HSC, we used irradiated competitor BM for all experimental groups. After 22 weeks, at which point hematopoiesis should be dependent on long-term HSC activity, we measured the representation of GFP+ cells in peripheral blood (Fig. 1B). Based on the ability of different doses of BM to contribute to hematopoiesis in both the myeloid (Mac1+) and B-cell (B220+) lineages, we calculated an HSC frequency of approximately 1/13,000 for unirradiated BM and 1/133,000 for previously irradiated BM (Supplementary Fig. S5; note that prior irradiation did not affect HSC homing to the BM). Thus, irradiation results in a roughly 10-fold reduction in the numbers of functional HSC, which likely contributes to reduced competitive repopulation.
Notably, reductions in numbers of HSC in previously irradiated BM are insufficient to explain the extent of dominance of non-irradiated competitors seen in competitive transplantation experiments. Using the calculated frequency of HSC, each recipient shown in Figure 1A transplanted with 106 non-irradiated GFP+ and 9×106 irradiated BM cells should contain 78 non-irradiated GFP+ (106 × 1/13,000) and 67 irradiated GFPneg (106 × 1/133,000) HSC. Assuming no differences in fitness per HSC, one would expect ~46% of cells to be GFPneg (67/(67+78)=0.46). Even if we assume the highest value for HSC concentrations in unirradiated BM and the lowest value for irradiated BM (based on the 95% confidence interval), transplantation of the estimated 128 unirradiated and 40 irradiated HSC should lead to ~24% GFPneg cells in recipients (40/(40+128)=0.24), and yet we observe no detectable contributions to hematopoiesis from irradiated donors (Fig. 1A). Also, while based on the calculated HSC frequencies, non-irradiated “test” cells are expected to contain an average of 1.5 HSC/2×104 cells and irradiated “test” cells are expected to contain 1.87 HSC/2.5×105 cells, recipients of 2×104 non-irradiated cells show significantly greater GFP percentages in MAC+ and B220+ lineages compared to recipients of 2.5×105 irradiated cells (Fig. 1B). Therefore, we conclude that irradiation not only decreases the numbers of functional HSC, but also substantially decreases the fitness per HSC.
Reactive Oxygen Species contribute to irradiation induced impairment of hematopoietic progenitor fitness
The majority of γ-irradiation-induced DNA damage, resulting both from direct hits to DNA molecules and transiently induced reactive oxygen species (ROS), occurs within milliseconds (24). However, even long after irradiation, persistent ROS are thought to contribute to continued DNA damage, even in cells not directly exposed to radiation (the “bystander effect”) (1). We therefore assayed plasma levels of 15-F2t-Isoprostane, which is produced by the random oxidation of tissue phospholipids by oxygen radicals and serves as a reliable biomarker of oxidative stress (25). Plasma levels of 15-F2t-isoprostane were substantially elevated by irradiation (IR), and the increase persisted for at least 3 weeks post-irradiation (Fig. 2A).
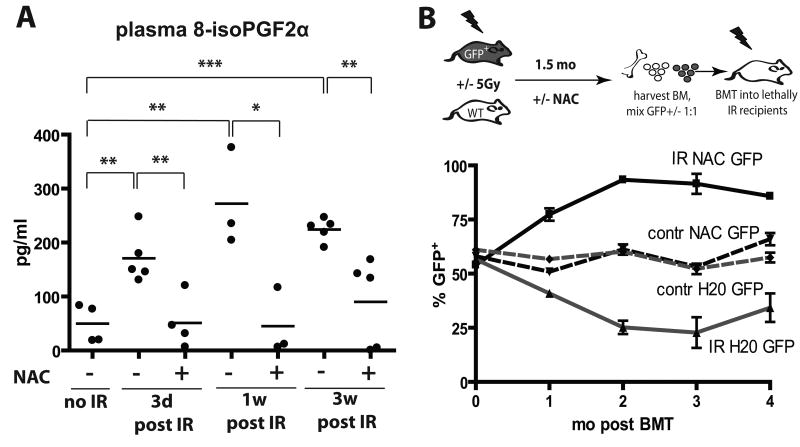
A) Mice were irradiated at 5 Gy and then transferred to cages with water +/- 40μM NAC. 8-isoPGF2α levels in plasma were determined as described in Materials and Methods. B) GFP-Tg and Balb/c mice were irradiated with 5 Gy (IR) or left untreated (contr) and transferred into cages with water containing 40μM NAC or plain water (“H20”). Six weeks later, BM was isolated, cells were mixed 50:50 in the following groups (from top to bottom in the graph): IR-NAC-GFP:IR-H20-Balb/c, contr-NAC-GFP:contr-H20-Balb/c, contr-H20-GFP:contr-NAC-Balb/c, and IR-H20-GFP:IR-NAC-WT (labeled by the GFP-Tg partner). Percentages of GFP+ cells in the initial mixes were confirmed by flow cytometry (0 time-point). The mixes were then transplanted into lethally irradiated recipients with 5 mice/group. Peripheral blood was collected at 1, 2, 3 and 4 months post transplantation and analyzed for GFP expression in the MAC1+ lineage.
We next asked whether induction of secondary ROS contributes to reduced fitness of hematopoietic progenitors, by using administration of the ROS scavenger N-acetyl cysteine (NAC). As expected, providing NAC before irradiation, which should reduce irradiation-induced generation of free radicals, resulted in substantially improved maintenance of long-term hematopoietic fitness (Supplementary Fig. S3), as judged by competitive BM transplantation assays. In contrast, NAC treatment started after irradiation resulted in only a partial improvement of hematopoietic fitness as assessed by competition with unirradiated progenitors, in that NAC treatment post-irradiation significantly improved contributions to short-term lymphopoiesis and myelopoiesis, but not long-term myelopoiesis (which is dependent on HSC activity). Notably, administration of NAC post-irradiation was capable of substantially reversing irradiation-induced ROS (Fig. 2A).
Since unirradiated BM enjoys a dramatic competitive advantage over irradiated BM, partial effects of NAC treatment post-irradiation could be masked. Therefore, to better assess the impact of NAC treatment on the fitness of hematopoietic progenitors, we performed transplantation experiments where BM from irradiated NAC-treated donors compete with BM from irradiated donors not treated with NAC (mixed 1:1; donor BM harvested 6 weeks post-irradiation). As controls, similar transplants were performed with BM from unirradiated donors, with or without NAC treatment. We used two sets of transplantations, where either NAC treated or control donors were on the GFP-Tg background. Relative contributions to the myeloid lineage (Mac1+) were followed over time (Fig. 2B). NAC treatment post-irradiation provided clear protection from fitness loss, as BM from NAC treated irradiated mice out-competed BM from untreated irradiated mice irrespective of the GFP genotype of NAC treated donors (Fig. 2B). Given that the effects of NAC treatment were evident in the myeloid lineage 4 months post-transplant (26), NAC treatment post-irradiation appears to protect the fitness of HSC. In contrast, NAC treatment had no impact on competitive repopulation using BM from unirradiated donors.
Since ROS are expected to cause DNA damage, we monitored the effects of irradiation and NAC treatment on levels of phosphorylated H2AX (γH2AX), a commonly used marker for DNA strand breaks(27), in BM cells. We found that almost all nucleated BM cells exhibited high γH2AX staining within 2 hours of irradiation and about a third of cells exhibited γH2AX staining 7 days after irradiation (Fig. 3A,B). Treatment of mice with NAC post-irradiation significantly reduced the persistence of DNA damage at 7 days. Irradiation caused a clear increase in the fraction of reticulocytes with micronuclei, an established readout for chromosomal strand breaks in vivo, although in contrast to γH2AX staining, the reticulocyte micronuclei assay did not reveal any alleviation of DNA breaks by NAC treatment (Supplementary Fig. S6A). Induction of massive cell death in the BM and initial disruption of hematopoiesis were insensitive to NAC treatment, although peripheral leukocyte counts trend a little higher in the NAC group on day 7 (Fig. 3C and Supplementary Fig. 6B).
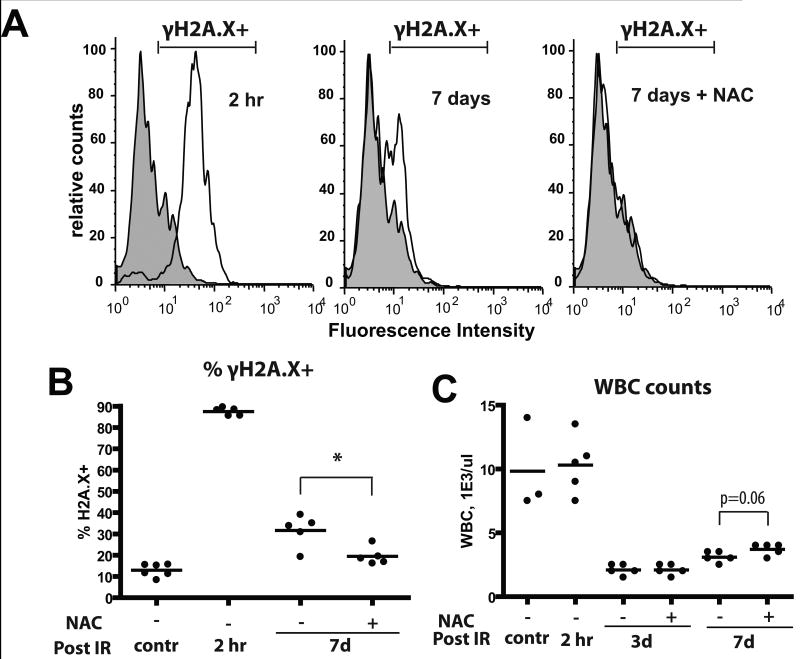
Mice were irradiated at 5 Gy and transferred into cages with water +/- 40μM NAC. At the indicated time-points, mice were sacrificed and BM harvested. A) BM cells were permeabilized and stained with a fluorescent-linked antibody to γH2AX. Representative flow profiles are shown (gray overlay plot: analysis of untreated/control BM). B) Percentages of γH2AX+ cells at the indicated time-points are plotted (5-6 mice/group). C) Leukocyte counts from peripheral blood were determined using automated CBC analysis.
Thus, inhibiting the prolonged induction of ROS after irradiation can partially alleviate the persistent fitness loss of hematopoietic progenitors, likely by partially limiting persistent DNA damage. Notably, by 6 weeks after irradiation, BM progenitors exhibit cell cycle profiles indistinguishable from BM from unirradiated mice. Still, a small but consistent increase in the fraction of cells with sub-G1 DNA content (indicative of apoptosis) and modestly increased p53 protein levels were evident, irrespective of NAC treatment (Supplementary Fig. S6C,S6D). Nonetheless, the exact mechanisms responsible for irradiation-induced loss of hematopoietic progenitor cell fitness are not clear.
Irradiation leads to long-lasting alterations of the adaptive landscape, changing the selective effects of oncogenic mutations
We previously demonstrated that conditions that impair DNA replication in hematopoietic progenitor cells dramatically increase the selective advantage conferred by Bcr-Abl, leading to enhanced leukemogenesis (10). Since irradiation causes a dramatic and persistent impairment of hematopoietic fitness, we decided to test if prior irradiation can impact the ability of different oncogenes to drive clonal expansion of hematopoietic cells and cause leukemias. BM was harvested from mice irradiated 2 months prior at 5 Gy, transduced with MSCV-ires-GFP (MiG) retroviruses encoding several different oncogenes at a sufficient efficiency to induce leukemias in recipients of non-irradiated BM, and then transplanted into recipient mice. The recipients of vector-transduced BM exhibited similar contributions of GFP-expressing cells to the myeloid and B-cell lineage, independent of prior irradiation, and thus previous irradiation does not appear to affect retroviral transduction (Fig. 4).
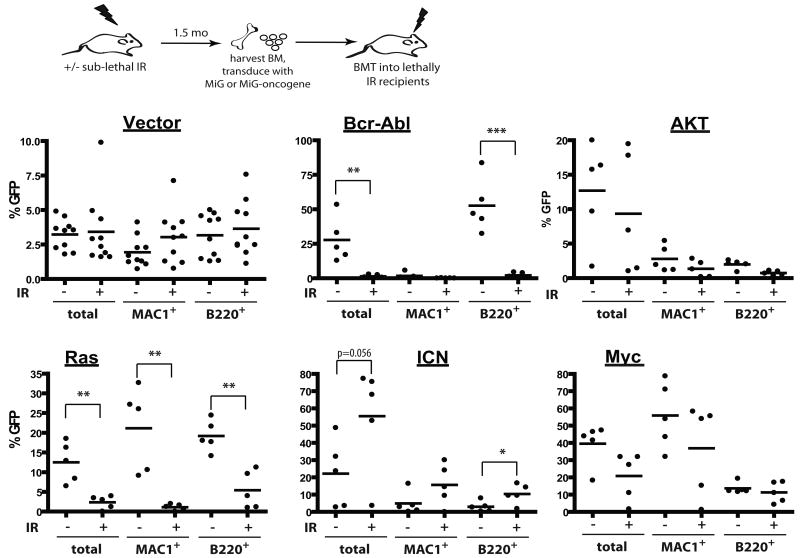
Mice were left untreated (-) or irradiated with 5 Gy (+). Six weeks later, mice were sacrificed, and BM was isolated and transduced with MiG vector or MiG expressing p185-Bcr-Abl, activated Akt, c-Myc, N-Ras12D or ICN, and immediately transplanted into lethally irradiated recipients (5×106 cells/mouse). Initial transduction efficiencies were determined after 3 days of culture under HSC conditions: V (3.80%, 5.08%), Bcr-Abl (0.2, 0.2), Myc (7.77%, 12.43%), Akt (2.25, 2.79), Ras (1.76, 3.48) and ICN (0.92, 1.05), for unirradiated and irradiated BM cells, respectively. At 1 month post transplantation, GFP expression was determined within peripheral blood MAC1+ and B220+ cells, as well as within total nucleated blood cells.
At 1 month post-transplantation, recipients of non-irradiated BM transduced with p185-Bcr-Abl or activated N-Ras12D displayed high percentages of GFP+ cells in peripheral blood (in B-cells for Bcr-Abl and in both myeloid and B-cells for Ras), suggesting a strong selective advantage in BM progenitors (Fig. 4). In striking contrast, percentages of GFP+ cells were quite low in peripheral blood of recipients that were transplanted with BM from previously irradiated recipients which had been transduced with Bcr-Abl or Ras, indicating that previous irradiation blunts the selective advantage conferred by either oncogene (Fig. 4). Note that prior irradiation did not affect the activation of downstream effectors by these oncogenes (Supplementary Fig. S7). Prior irradiation had no clear effect on the expansion of hematopoietic progenitor cells expressing Myc, Bcl2 or activated Akt (Fig. 4 and Supplementary Fig. S8). In contrast, we observed a stronger selection for cells that express ICN, the intracellular domain of Notch1 that possesses constitutive transcriptional activity (28), in previously irradiated BM (Fig. 4).
Observed differences in the expansion of oncogene-expressing cells translated into difference in leukemogenesis: previous irradiation decreased or substantially delayed leukemogenesis driven by Ras or Bcr-Abl, increased leukemogenesis driven by ICN, and had no significant effect on leukemias induced by Akt and Myc (Fig. 5). Thus, prior irradiation can alter the adaptive landscape, either increasing or decreasing the selective effects of particular oncogenic mutations. Of note, previous irradiation had no noticeable effect on the types of leukemias that developed from the introduction of specific oncogenes, with ICN expression promoting CD4+CD8+ T-cell leukemias, Ras or Myc expression promoting mixed B-lineage/myeloid leukemias, and AKT inducing mostly lineageneg leukemias, in each case irrespective of irradiation (data not shown).
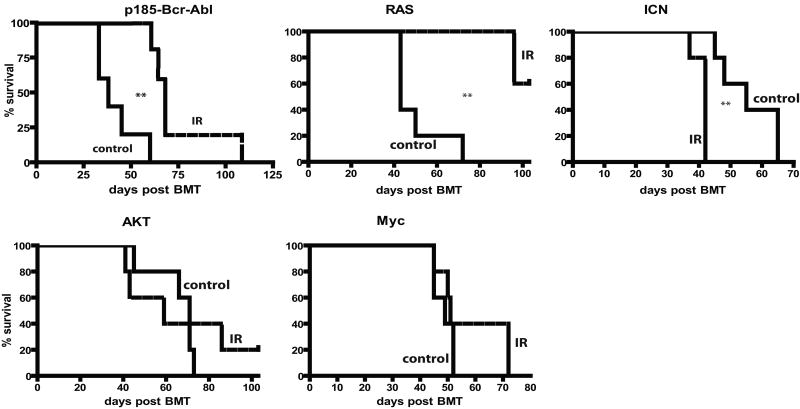
Leukemia-free survival for mice described in Figure 4 is graphed using Kaplan-Meier curves (n=5/group). Spleen weights, CBC profiles and tissue metastases were determined in sacrificed moribund mice. All mice that died succumbed to leukemia, as determined by the presence of GFP+ blasts in multiple tissues at high percentages (>20% in BM), splenomegaly and high peripheral blast counts. No recipients of MiG-transduced BM developed leukemias.
The results presented in Figures 4 and and55 do not exclude the possibility that irradiation induces specific oncogenic alterations that are capable of cooperating with ICN, but not with other tested oncogenes, in promoting expansion and leukemogenesis. Should this be the case, the effects of irradiation on ICN-driven leukemogenesis would be expected to be cell-autonomous. We therefore examined the effect of non-irradiated competitors on the ability of ICN to drive clonal expansion and leukemogenesis from previously irradiated hematopoietic progenitors. BM was isolated from mice that were irradiated 6 weeks before (IR) or left untreated (C), and then transduced with MiG-ICN (IR/ICN or C/ICN).
Transduced progenitors were then transplanted into lethally irradiated recipients, along with an excess of competitor BM cells. Co-transplantation of unirradiated progenitors (C-comp) with the ICN-transduced irradiated progenitors eliminated the increased competitive expansion observed in the irradiated background (Fig. 6A), leading to substantially reduced leukemogenesis (Fig. 6B). Thus, the acceleration of ICN-driven expansion and leukemogenesis by previous irradiation is in part non-cell autonomous, as restoring the fitness of hematopoiesis by transplantation of non-irradiated progenitors blocks ICN-driven leukemogenesis. Finally, if the observed expansion of ICN-expressing cells is due to random cooperating mutations, it would be expected to be monoclonal. However, analyses of T-cell receptor expression in expanding ICN+ T-cells in the irradiated background at 4 weeks post-transplant reveals that these populations are polyclonal (Supplementary Fig. S9).
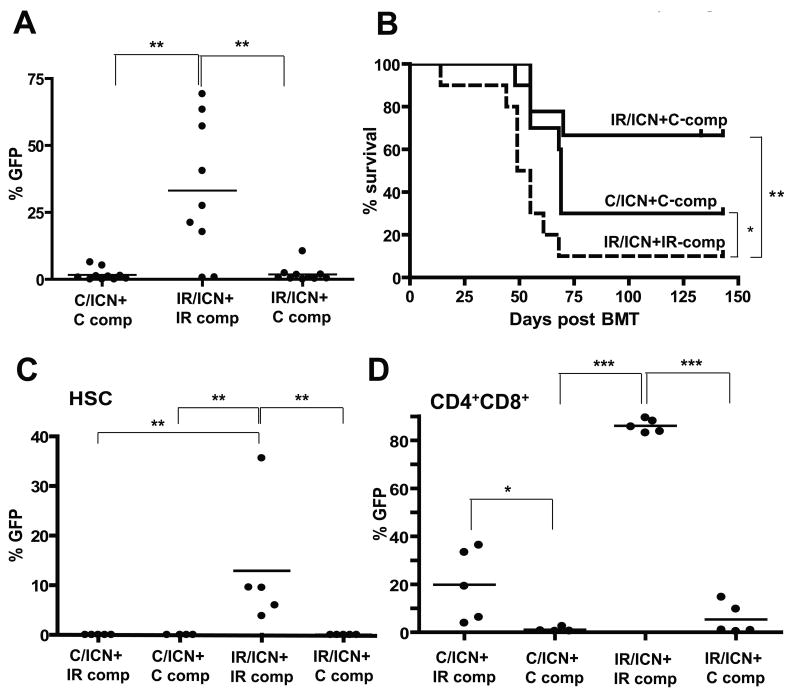
Mice were irradiated with 5 Gy (IR) or left unirradiated (C). Six weeks later, the mice were sacrificed, and isolated BM was transduced with MiG-ICN (initial transduction efficiencies were C:1.3% and IR:0.8%). Transduced cells were mixed with IR or C competitor BM and transplanted into lethally-irradiated recipients with 1×106 transduced cells and 3.5×106 non-transduced competitors per mouse. A) Percentages of GFP+ cells were determined among CD4+ T-cells at 5 weeks post-transplantation. B) Leukemia-free survival was analyzed as in Figure 5. All deaths were attributable to CD4+ or CD4+CD8+ leukemias. C) A similar transduction and transplantation of C and IR BM with MiG-ICN was performed, but with inclusion of a fourth group (C/ICN+IR-comp). BM was harvested 4 weeks post-transplantation and GFP expression within the HSC-enriched CD150+Linneg/CD48neg populations determined. P values are for Fisher exact test. D) Expression of GFP within BM CD4+CD8+ populations was determined.
Since Notch1 has been implicated in self-renewal of HSC (29), we decided to test whether previous irradiation affects selection for ICN expression at the HSC level. We performed similar experiments to those described in Figure 6A, except that the mice were sacrificed 4 weeks post-transplantation. We determined the representation of GFP+ICN+ cells within the HSC-enriched CD150+CD48negLinneg BM compartment (23). Flow cytometric analysis of BM from these recipient mice at 4 weeks post-transplantation revealed dramatic expansion of ICN-expressing cells within HSC-enriched pools only in the irradiated background. Again, the inclusion of unirradiated competitors completely reversed the selective advantage provided by ICN expression (Fig. 6C and Supplementary Fig. S10A,B). Thus, previous irradiation promotes selection for ICN at the level of early progenitors.
ICN expression in early progenitors is known to promote T-cell development in the BM (normally restricted to the thymus), and to also inhibit B-cell development (30). Indeed, we found that ICN-expressing progenitors completely dominated CD4+CD8+ T-cell pools in the BM in the irradiated background (IR/ICN+IR-comp), but not when unirradiated competitors were present (Fig. 6D and Supplementary Fig. S10C,D and S11B). B-lymphopoiesis was also significantly impaired in the IR/ICN+IR-comp group of mice (Supplementary Fig. S11A). Unlike for HSC-enriched pools, the inclusion of irradiated competitors did allow for some expansion of ICN-expressing unirradiated cells (C/ICN+IR-comp) within BM CD4+CD8+ pools, again highlighting the non-cell autonomous contribution of irradiated hematopoiesis towards the expansion of ICN-initiated cells (Fig. 6D and Supplementary Fig. S10C). Notably, only a minor fraction (<1%) of control progenitors were transduced with MiG-ICN, and thus even with the inclusion of irradiated competitors, non-transduced unirradiated progenitors eventually dominate hematopoiesis and restore competitiveness.
Discussion
Increased cancer rates that result from exposure to radiation or other carcinogens are generally attributed to the mutagenic actions of these agents via increasing frequencies of mutations in oncogenes and tumor suppressor genes (3, 4). Surprisingly, evidence to support a role for γ-radiation in the direct induction of oncogenic mutations is largely lacking (1, 12). We and others have previously proposed an alternative explanation: DNA damaging carcinogens might induce growth inhibitory conditions which lead to selection for oncogenic mutations that confer at least partial resistance to the defect (6, 10-13). Of course, mutagenic and selective effects of carcinogens need not be mutually exclusive. Ultraviolet light exposure of the skin represents perhaps the best example of a carcinogenic agent that has been shown to both directly result in oncogenic mutations (such as in p53) and to promote the clonal expansion of progenitors with these mutations (31-33). The studies described here tested the long-term effects of X-irradiation on the fitness of hematopoietic progenitor populations and on selection for different oncogenic mutations.
Previous studies have shown that the accumulation of DNA damage, resulting either from γ-irradiation or DNA repair deficiency, leads to reductions in the repopulating ability of HSC (34-36). Here, we show that sub-lethal irradiation impacts on HSC fitness both by the permanent loss of repopulating activity and by reducing the per-cell fitness of HSC that retain some repopulating capacity. We further demonstrate that persistent ROS induction that follows irradiation is partially responsible for this reduction of fitness. These results raise the possibility that people who are exposed to γ-irradiation might benefit from treatment with anti-oxidants, even if treatment is initiated after exposure.
The key finding of our study is that previous irradiation substantially alters the selective impact of certain oncogenic mutations, augmenting (ICN), inhibiting (Ras and Bcr-Abl) or not affecting (Akt and Myc) the ability of oncogenes to drive clonal expansion and leukemogenesis. These results argue against the prevailing paradigm whereby irradiation promotes leukemogenesis by inducing the accumulation of cooperating oncogenic events, as one would need to surmise that irradiation induced oncogenic events cooperated with some oncogenes, while inhibiting leukemia induction by others. Our results instead provide support for the importance of selection in oncogenesis: different oncogenic events should be adaptive for different contexts of reduced cellular fitness (or an otherwise altered environment). Alteration of selective pressures following irradiation is consistent with the idea that cancers arise within a fairly tight trajectory determined by the adaptive landscape specific for a particular tissue, developmental stage and carcinogenic exposure (37), which could underlie the association of particular oncogenic events with cancers initiated by particular carcinogenic contexts.
Activating mutations in Notch1 are very common in human T-cell acute lymphoblastic leukemias (T-ALL) (28, 38). Notch1 mutations that generate N-terminally truncated proteins, predicted to functionally mimic the ICN oncoprotein, are found in over half of T-lymphomas induced in mice by γ-irradiation (39). While the authors suggested that irradiation causes T-lymphomas by generating strand breaks at the Notch1 locus, our data indicate that previous irradiation creates a context that potently selects for activating Notch1 mutations in progenitor pools.
Our experiments indicate that this selection occurs within early multipotent progenitors, since we detect substantial expansion of ICN-expressing cells in HSC-enriched pools of previously irradiated BM, and increased representation of ICN-expressing cells is evident within multiple hematopoietic lineages. Of relevance, a recent study demonstrated that ICN expression is insufficient to confer self-renewal to T-precursors, as transfer of the initial polyclonal ICN-expressing CD4+CD8+ population into secondary recipient mice resulted in loss of these cells within a week (40). In contrast, cells with properties of committed T-progenitors from monoclonal ICN-induced leukemias did efficiently transfer the disease. Thus, should an activating Notch1 mutation occur in a T-precursor, in all likelihood this clone would be lost long before other events could accumulate. But if the Notch1 mutation happens in an HSC and is selected for, a pool of ICN-expressing T-precursors could be maintained, which would then have the potential to accumulate additional events leading to leukemia development.
We hypothesize that irradiation can promote carcinogenesis by decreasing the fitness of progenitor cells, thereby increasing selection for oncogenic mutations that can provide some adaptation in the face of this defect (6). While the results presented here support this hypothesis, they do not provide definitive proof. Increased selection for ICN-expressing cells could also be influenced by non-cell-autonomous effects of irradiation on hematopoiesis. For example, irradiated hematopoietic cells could produce higher levels of inflammatory cytokines, and the transplanted non-irradiated BM could partially alleviate this environmental change by replacement of irradiated hematopoiesis. Irradiation-induced senescence of fibroblasts dramatically boosts cytokine production (41, 42), which could be envisioned to promote tumorigenesis either by selective pro-proliferative effects of cytokines on initiated cells or by selectively impairing the fitness of non-initiated cells. Regardless, in the irradiated background, ICN mutations clearly confer a greater advantage relative to a normal background. Thus, ICN-expressing progenitors must have a fitness advantage relative to other irradiated progenitors, supporting the idea that irradiation increases cancer predisposition at least in part by altering the environment and thus the adaptive landscape.
In summary, our results argue that irradiation substantially changes the selective impact of different oncogenic mutations, either inhibiting or promoting oncogene-driven expansion. The ability of non-irradiated competitors to limit ICN-driven expansion from irradiated cells supports the idea that protecting or restoring the fitness of a stem cell population might limit the onset of cancer even when oncogenic mutations cannot be avoided.
Acknowledgments
JD is supported by the National Institutes of Health (RO1-CA109657). We would like to thank Drs. Warren Pear and Carlo Maley for helpful advice, Drs. Christopher Porter, Mark Gregory and Francis Rodier for critical review of this manuscript, and Karen Helm and Christine Childs of the Cancer Center Flow Cytometry Core, supported by NIH grant 2P30-CA46934.
References
Full text links
Read article at publisher's site: https://doi.org/10.1158/0008-5472.can-09-0604
Read article for free, from open access legal sources, via Unpaywall:
https://aacrjournals.org/cancerres/article-pdf/69/18/7262/2613503/7262.pdf
Free to read at cancerres.aacrjournals.org
http://cancerres.aacrjournals.org/cgi/content/abstract/69/18/7262
Free after 12 months at cancerres.aacrjournals.org
http://cancerres.aacrjournals.org/cgi/reprint/69/18/7262.pdf
Free after 12 months at cancerres.aacrjournals.org
http://cancerres.aacrjournals.org/cgi/content/full/69/18/7262
Citations & impact
Impact metrics
Citations of article over time
Smart citations by scite.ai
Explore citation contexts and check if this article has been
supported or disputed.
https://scite.ai/reports/10.1158/0008-5472.can-09-0604
Article citations
Genetic Determinants of Somatic Selection of Mutational Processes in 3,566 Human Cancers.
Cancer Res, 81(16):4205-4217, 02 Jul 2021
Cited by: 6 articles | PMID: 34215622 | PMCID: PMC9662923
Chronic interleukin-1 exposure triggers selection for Cebpa-knockout multipotent hematopoietic progenitors.
J Exp Med, 218(6):e20200560, 01 Jun 2021
Cited by: 31 articles | PMID: 33914855 | PMCID: PMC8094119
Whole-Exome Sequencing of Radiation-Induced Thymic Lymphoma in Mouse Models Identifies Notch1 Activation as a Driver of p53 Wild-Type Lymphoma.
Cancer Res, 81(14):3777-3790, 25 May 2021
Cited by: 7 articles | PMID: 34035082 | PMCID: PMC8286346
Effects of yak-activated protein on hematopoiesis and related cytokines in radiation-induced injury in mice.
Exp Ther Med, 14(6):5297-5304, 03 Oct 2017
Cited by: 1 article | PMID: 29285056 | PMCID: PMC5740812
Mice Lacking RIP3 Kinase are not Protected from Acute Radiation Syndrome.
Radiat Res, 189(6):627-633, 10 Apr 2018
Cited by: 3 articles | PMID: 29634408 | PMCID: PMC6020684
Go to all (30) article citations
Data
Data behind the article
This data has been text mined from the article, or deposited into data resources.
BioStudies: supplemental material and supporting data
Similar Articles
To arrive at the top five similar articles we use a word-weighted algorithm to compare words from the Title and Abstract of each citation.
Irradiation selects for p53-deficient hematopoietic progenitors.
PLoS Biol, 8(3):e1000324, 02 Mar 2010
Cited by: 102 articles | PMID: 20208998 | PMCID: PMC2830447
Declining lymphoid progenitor fitness promotes aging-associated leukemogenesis.
Proc Natl Acad Sci U S A, 107(50):21713-21718, 22 Nov 2010
Cited by: 55 articles | PMID: 21098275 | PMCID: PMC3003039
Ionizing radiation and hematopoietic malignancies: altering the adaptive landscape.
Cell Cycle, 9(15):3005-3011, 07 Aug 2010
Cited by: 19 articles | PMID: 20676038 | PMCID: PMC3040925
Review Free full text in Europe PMC
Influence of diet and metabolism on hematopoietic stem cells and leukemia development following ionizing radiation exposure.
Int J Radiat Biol, 95(4):452-479, 24 Sep 2018
Cited by: 5 articles | PMID: 29932783
Review
Funding
Funders who supported this work.
NCI NIH HHS (6)
Grant ID: P30 CA046934
Grant ID: R01 CA109657-04
Grant ID: R01 CA109657
Grant ID: R01 CA109657-02
Grant ID: R01-CA109657
Grant ID: R01 CA109657-03
NIAID NIH HHS (1)
Grant ID: T32 AI007405