Abstract
Free full text

Genetic Modulation of Hormone Levels and Life Span in Hybrids Between Laboratory and Wild-Derived Mice
Abstract
Previously we showed that mouse stocks derived from wild-caught progenitors are long-lived relative to genetically heterogeneous mice derived from laboratory-adapted strains. Here we replicate this life-span effect, and show that F2 hybrids between wild-derived and laboratory-derived stocks have intermediate survival patterns. Moreover, wild-derived mice are small, lean, and slow to mature, and have low serum insulin-like growth factor-I (IGF-I) relative to genetically heterogeneous mice. These traits, too, were at intermediate levels in the F2 hybrids. Furthermore, serum IGF-I at 6 months was a significant predictor of life span in two different populations of F2 hybrid mice. Pooling across stocks, life span was negatively correlated with body weight and serum IGF-I levels, and positively correlated with age at vaginal patency and serum leptin levels. Overall, these finding suggest that wild-derived mice harbor alleles that increase longevity, perhaps through effects on growth, maturation, and early-life hormone levels.
Most common laboratory stocks of mice have been raised under artificial vivarium conditions for hundreds of generations (1). Such conditions select for docility and other behavioral abnormalities, for promiscuity and inattention to seasonal breeding cues, and for large body size, large litter size, and rapid maturation rate. All of these changes occur as the consequence of selection pressures, largely unplanned, imposed by vivarium caretakers. Previously, we hypothesized that these selection pressures may have led to the loss, within laboratory-selected populations, of naturally occurring alleles that act to slow the aging process (2). An initial life-span study of wild-derived mouse stocks supported this notion in two wild-derived populations, one consisting of descendants of mice live-trapped in North-Central Idaho (Id), and the other derived from the South Pacific island of Majuro (Ma) (3).
In addition to being long-lived, female Id and Ma mice take longer to become sexually mature, are significantly smaller, and have lower serum insulin-like growth factor-I (IGF-I) levels at 6 months than do a laboratory-derived, genetically heterogeneous control stock, suggesting that the underlying genetic basis for reproductive maturation rates, body size, and hormone levels could be central to life-span regulation in mice. Indeed, direct selection for reduced growth rates in the first 2 months of life leads to an increase in longevity in genetically heterogeneous populations of laboratory mice (4), and mutations that lead to a reduction in IGF-I signaling pathways extend life span in both invertebrate (5,6) and mouse models of aging (7,8). Delayed reproduction also has been associated with a significant increase in longevity in flies (9,10).
Despite widely divergent phenotypes between laboratory- and wild-derived mice, simple interstrain comparisons do not directly address whether the observed differences between traits of interest (e.g., body weight and life span) are the result of a causal connection, due to genetic alleles with pleiotropic effects, or if instead they are simply the result of random coincidental occurrence that has been retained by natural selection. However, the construction of segregating populations of F2 intercross stocks results in a significant degree of genome reshuffling, thereby producing offspring with variable combinations of alleles (11). Unlike F1 hybrid mice, which are heterozygous at all loci (with the exception of those loci that happen to be identical in the parental stocks), segregating populations of F2 mice have three possible genotypes for each allele: (i) homozygous for parent A; (ii) heterozygous; or (iii) homozygous for parent B. Hence, the co-occurrence of traits in individual members of a segregating population indicates that these traits are regulated to some degree by common, and potentially genetic, factors (12). Models that attribute the superior longevity of Id mice to delayed maturation, low IGF-I levels, or altered growth trajectories would predict cosegregation of each of these traits with life span in an F2 population. Traits that cosegregate with life span might be important to the aging process directly, or might alternately prove to be indices of processes that themselves modify aging and the onset of age-related pathologies.
In this study, life span, life history measures, and hormone levels in young (6-month-old) and middle-aged (14-month-old) females were collected from individuals from each of six populations of genetically distinct mouse stocks: a laboratory-derived “diversity control” stock (DC), two wild-derived stocks (Id and Ma), and three F2 hybrid stocks [(Id × DC)F2, (Id × C57BL/6)F2, and (Ma × C57BL/6)F2]. In addition to documenting interstock differences in patterns of growth, maturation, and endocrine profiles, the ability of each measure to act as a predictor of life span was assessed.
Materials and Methods
Mouse Stocks and Husbandry
The Id and Ma stocks used in this study were derived from wild-caught progenitors as described in (2). Wild-caught animals were used to establish the G0 generation in captivity, from which all subsequent generations were then derived as described in (3). Mice of the G4 generation were used for this study. The genetically heterogeneous DC control stock has been described in detail elsewhere (3); mice in this stock have equal genetic contributions from the laboratory-inbred strains BALB/cJ, C57BL/6J, C3H/HeJ, and DBA/2J. For these studies, DC mice of the G12 generation were used as controls. In addition, three stocks were created as crosses between wild- and laboratory-derived stocks of mice using breeders from the G3 generation of Id and Ma mice, 11th generation DC mice, and C57BL/6J (B6) mice purchased from the Jackson Laboratories (Bar Harbor, ME). In each case, an F1 generation was produced as the result of a mating between Id or Ma males and DC or B6 females. Males and females of the F1 generation were then crossed to produce an F2 generation, i.e., (Id × DC)F2 (=“IdDCF2”), (Id × C57BL/6)F2 (=“IdB6F2”), and (Ma × C57BL/6)F2 (= “MaB6F2”), for use in this study.
Mice were entered into the study in staggered cohorts from litters born between December 2000 and August 2001. Within 3 days of their birth, all litters were culled to four pups to ensure equal access to maternal milk, and all mice were weaned at 21 days of age. Only females were used in this study, and were housed in individual cages in the University of Michigan Cancer Center and Geriatrics Center Building vivarium until April 2003, when they were transferred to a colony in the Kresge Building. The final study population consisted of 40 DC, 40 Id, 41 Ma, 41 IdDCF2, 50 IdB6F2, and 50 MaB6F2 mice. Mice were inspected at least once daily to record the date of death, and were killed if found to be so severely ill that in the opinion of an experienced caretaker they were thought unlikely to survive more than another few days. All mice were fed commercial rodent chow (Lab Diet 5001; PMI Nutrition International, Brentwood, MO) and were provided with tap water ad libitum. Room temperature was maintained at 72 ± 4°F in both buildings, with 10–15 fresh air changes per hour and a 12-hour light/dark cycle. To evaluate the health status of the mice, groups of sentinel mice were exposed to soiled bedding from the study population on a quarterly basis, and were later evaluated serologically for the presence of specific viral and bacterial pathogens. The animals were also examined for pinworm. In October 2001, a sentinel mouse was found to be infected with pinworm, and all animals in the study were therefore treated first with Ivermectin for 7 days, followed by a 6-week course of fenbendazole in their diet. Quarterly tests for pinworm were negative thereafter. In addition, some mice in the vivarium were found in February 2003 to have titers for mouse hepatitis, including mice on one of the three racks housing the animals reported in this study. The mice therefore cannot be considered to have been specific pathogen-free throughout their lives.
Beginning at 15 days of age mice were examined daily until vaginal patency was observed. Ethanol-sterilized forceps were used to allow optimal observation of the genital area. Beginning at 1 month of age, mice were weighed once per month until 4 months, then weighed again at 6, 8, 12, and 15 months. Beginning at 18 months, all mice were weighed monthly until the time of their death. At 6 months, the total body length (tip of nose to base of tail) of each mouse was also determined.
All mice were bled once at 6 months for the quantification of circulating hormone levels. In addition, each mouse was immunized at the age of 14 months using turkey erythrocytes, and then bled 2 weeks later to perform a hemagglutination assay. Blood from these samples was also used for the quantification of serum hormone levels. Mice were also bled again at 18 months for the determination of T-cell subset distributions, and were subjected to cataract examinations at 18 and 24 months. To minimize the influence of circadian rhythmicity, all blood samples were collected between 9:00 am and 12:00 pm via puncture of the retro-orbital sinus without anesthesia. In addition, fecal pellets (1–2 per mouse) were collected opportunistically for five consecutive days from 17-month-old mice for the determination of fecal corticosteroid level.
Hormone Measures
Blood samples for hormone measurements were taken via retro-orbital venipuncture, and serum was stored at −70°C until the time of assay. Serum T4 levels were determined using a monoclonal solid-phase radioimmunoassay (RIA) kit (MP Biomedicals Inc., Costa Mesa, CA, formerly ICN Biomedicals) run at one-quarter volume according to the manufacturer's instructions. Serum IGF-I levels were quantified via a double-antibody RIA kit (Diagnostic Systems Laboratories, Webster, TX) run at one-quarter volume according to the manufacturer's instructions. Prior to assay, 5–10 μL of serum from each mouse was subjected to an acid–ethanol extraction procedure using the materials provided in the kit. Serum leptin levels were quantified with a double-antibody RIA kit (Linco Research, Inc., St. Charles, MO) according to the manufacturer's instructions except that all volumes were reduced by a factor of 4. In all cases, samples were assayed in duplicate.
Fecal corticosteroid levels were quantified with a double-antibody 125I-corticosterone RIA kit (MP Biomedicals Inc.) after hormone extraction using an ethanol-based procedure as described in (13). This procedure has been validated for use in multiple species of rodent as a specific, precise, and accurate integrated measure of circulating corticosteroid concentrations. All samples were assayed in duplicate, and the assay was performed according to the manufacturer's instructions at one-quarter volume. The 25 ng/mL standard provided with the kit was diluted 1:2 with steroid diluent (provided with the kit) to allow the inclusion of a 12.5 ng/mL standard (the lower limit of detection for this assay). The mean 5-day corticosteroid concentrations, presented as nanograms of corticosteroids per gram of dry weight of feces, were used for analysis. We have previously shown that the day-to-day variation in fecal corticosteroid levels over a typical 5-day sampling period is approximately 36% (14).
Statistical Analyses
The effect of stock on life-span data, life history traits, and hormone measures was evaluated by one-way analysis of variance. Differences between groups were evaluated using Dunnett's test, which compares each group to a predefined control group, i.e., DC. The effect of age on serum hormone measures within each stock was evaluated using a paired t test. The strength of the association between life span and each of the life history traits and hormone measures was evaluated using bivariate linear regression. Survival curves were constructed using the Kaplan–Meier method, and differences between pairs of stocks were evaluated using the log-rank test followed by Holm's Stepdown Bonferroni Procedure.
Results
Survival
Table 1 collects the summary statistics for each stock, and the Kaplan–Meier survival curves are illustrated in Figure 1. As seen in a previous, independent, experiment (3), both the Id and Ma stocks are long-lived relative to the DC stock by log-rank test (p ≤ .02). However, after adjusting for multiple comparisons using Holm's Stepdown Bonferroni Procedure, this difference was only marginally significant for the Ma stock (p = .06), although Id mice were still significantly long-lived (p = .0005). Id mice are also significantly longer-lived than each of the four remaining stocks (p ≤ .05), whereas the life span of the Ma mice is indistinguishable from the IdB6F2, IdDCF2, and MaB6F2 stocks (p > .25) after adjustment for multiple comparisons.
Table 1
Summary Statistics for Life-Span Data
Survivorship (d) | ||||||||
---|---|---|---|---|---|---|---|---|
Stock | N | Log-Rank p Value* | Adjusted p Value† | Mean Life Span (d) | 25th Percentile | 50th Percentile | 75th Percentile | 90th Percentile |
DC | 40 | — | — | 787 | 668 | 817 | 896 | 982 |
Id | 40 | .0001 | .0005 | 957 | 738 | 1004 | 1169 | 1230 |
IdB6F2 | 50 | .01 | .04 | 868 | 726 | 864 | 1036 | 1160 |
IdDCF2 | 41 | .07 | .14 | 846 | 678 | 892 | 980 | 1084 |
Ma | 40 | .02 | .06 | 829 | 556 | 871 | 991 | 1189 |
MaB6F2 | 50 | .9 | .9 | 724 | 555 | 678 | 908 | 1047 |
DC = diversity control; Id = North-Central Idaho; Ma = South Pacific Island of Majuro.
Interestingly, both the IdB6F2 and IdDCF2 mice had life spans that were intermediate to those of the DC and Id mice. IdB6F2 mice were significantly long-lived relative to the DC controls by log-rank test (p = .02), whereas the difference in life span was marginally significant (p = .07) for IdDCF2 mice. The life span of MaB6F2 mice was not significantly different from the DC controls (p > .45), and in fact both their mean (−8%) and median (−17%) life span were shorter.
Differences in maximum life span between stocks were analyzed using two different methods. The results of these analyses are summarized in Table 2. In the first, maximum life span was estimated as the mean life span of the longest-lived 10% of each population, and corresponded to increases of 24%, 12%, 7%, 14%, and 10% for the Id, IdB6F2, IdDCF2, Ma, and MaB6F2 mice, respectively. One-way analysis of variance, followed by Dunnett's test, was performed to assess differences between each stock and the DC mice. Using this method, DC mice were found to differ significantly from each of the wild-derived stocks (p < .03), and differences from the IdB6F2 hybrid stock (p = .056) approached significance, but not from IdDCF2 or MaB6F2 mice (p > .1). However, because the validity of this conditional t test (CTT) approach has come into question (15), the analysis of maximum life span was repeated using the Score statistic (the QT or “quantile test” method) as described in (15). Again, both the Id and Ma stocks had significantly greater maximum life spans than the DC mice after adjustment for multiple comparisons using Holm's Stepdown Bonferroni Procedure (p = .04). The QT method also indicated that IdDCF2 mice also had a significantly greater maximum life span (p = .05) than the DC control stock and that the maximum life span of the IdB6F2 stock was marginally greater (p = .06); however, neither of these comparisons approached significance after applying the Stepdown Bonferroni Procedure (p = .15). In addition, the CTT method suggested that Id mice had a higher maximum life span than the IdDCF2 and MaB6F2 hybrid stocks (p ≤ .01), which was confirmed using the QT method (p < .05).
Table 2
Analysis of Maximum Life Span
Quantile Test | |||||||
---|---|---|---|---|---|---|---|
Conditional t Test | |||||||
Life Span of 90th Percentile‡ | Proportion of DC Above 90th Percentile | Proportion of Stock Above 90th Percentile | p Value of Score Statistic§ | Adjusted p Value‖ | |||
Stock | Maximum Life Span* | p Value of Test† | |||||
DC | 1093 ± 94 | — | |||||
Id | 1357 ± 108 | <.001 | 1200 | 0.025 (1/40) | 0.20 (8/40) | .008 | .04 |
IdB6F2 | 1224 ± 89 | .056 | 1081 | 0.05 (2/40) | 0.16 (8/50) | .06 | .15 |
IdDCF2 | 1172 ± 99 | .41 | 1056 | 0.05 (2/40) | 0.17 (7/41) | .05 | .15 |
Ma | 1246 ± 57 | .03 | 1143 | 0.025 (1/40) | 0.20 (8/40) | .008 | .04 |
MaB6F2 | 1199 ± 47 | .10 | 1035 | 0.075 (3/40) | 0.14 (7/50) | .18 | .18 |
DC = diversity control; Id = North-Central Idaho; Ma = South Pacific Island of Majuro.
Life History Traits
DC mice mature earlier, are larger and fatter, and grow more rapidly than each of the wild-derived and F2 hybrid stocks (Table 3). In all but two cases (age at the onset of vaginal patency in IdB6F2 and MaB6F2 mice, p ≥ .1) these differences are significant (p ≤ .05). Moreover, DC mice are significantly heavier than each of the wild-derived and F2 hybrid stocks, beginning at 1 month of age and at all ages thereafter (p ≤ .01; Figure 2). Age at vaginal patency, body size and weight, adiposity (body mass index), and growth rate were intermediate in the F2 hybrids relative to the DC, Id, and Ma stocks. For each measure both the Id and Ma mice differed significantly (p ≤ .03) from each of the F2 hybrids with two exceptions: the age of vaginal patency in Ma mice was different relative only to the MaB6F2 hybrid stock (p < .001), and MaB6F2 did not differ in their degree of adiposity relative to the Id stock (p = .25). Id mice also took longer to mature than the Ma mice (p < .0001).
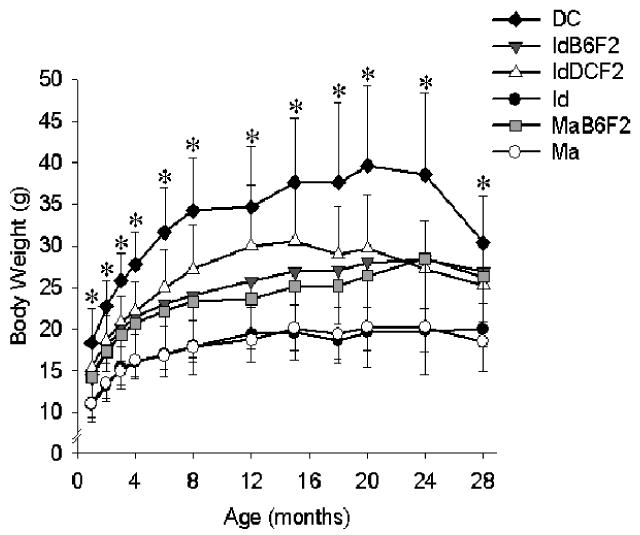
Weight as a function of age in each of the six stocks of mice. Each point represents the mean (± standard deviation) for each stock. N = 40–50 mice at 1 month, but due to natural deaths sample size decreased with time such that N = 17–26 at 28 months dependent upon stock. * Significant difference at p < .02 for the DC mice relative to the other stocks.
Table 3
Summary Statistics for Life History Traits
Stock | N | Vaginal Patency (d) | Body Length (cm)* | Body Mass Index† | Growth (g/mo)‡ |
---|---|---|---|---|---|
DC | 40 | 28.9 ± 5.5 | 10.2 ± 0.6 | 30.6 ± 5.3 | 3.1 ± 1.2 |
Id | 40 | 58.8 ± 23.6§ | 8.4 ± 0.4§ | 23.9 ± 2.6§ | 1.7 ± 0.6§ |
IdB6F2 | 50 | 34.5 ± 6.2 | 9.5 ± 0.5§ | 25.9 ± 3.2§ | 2.5 ± 0.9§ |
IdDCF2 | 41 | 35.9 ± 10.2§ | 9.4 ± 0.5§ | 28.5 ± 3.9§ | 2.3 ± 0.8§ |
Ma | 40 | 38.6 ± 14.6§ | 8.6 ± 0.6§ | 22.8 ± 3.4§ | 1.7 ± 0.7§ |
MaB6F2 | 50 | 30.1 ± 8.2 | 9.3 ± 0.5§ | 24.9 ± 3.5§ | 2.2 ± 1.2§ |
Values given are mean ± standard deviation.
DC = diversity control; Id = North-Central Idaho; Ma = South Pacific Island of Majuro.
Hormones
Table 4 summarizes interstock and age-related variation in IGF-I, T4, leptin, and corticosteroid levels. Most notably, serum IGF-I levels were significantly higher in the DC mice relative to each of the wild-derived and the MaB6F2 hybrid stocks at 6 months (p ≤ .04). At 14 months of age, serum IGF-I level was significantly higher in DC mice relative to the Id, IdB6F2, and IdDCF2 stocks (p < .001). As was true for the life span and life history data, the 6-month IGF-I levels in the F2 hybrid mice were intermediate between those seen in the wild-derived and DC stocks; these differences were significant (p < .01) for both the IdB6F2 and IdDCF2 mice relative to the Id parental stock.
Table 4
Summary Statistics for Hormone Levels
IGF-I (ng/mL) | Thyroxine (μg/dL) | Leptin (ng/mL) | Corticosteroid (ng/g feces) | |||||
---|---|---|---|---|---|---|---|---|
Stock | N | 6 Months | 14 Months | 6 Months | 14 Months | 6 Months | 14 Months | 17 Months |
DC | 27–37 | 646 ± 196 | 650 ± 155 | 5.9 ± 1.6 | 4.3 ± 1.8† | 10.0 ± 6.5 | 10.8 ± 7.3 | 45.4 ± 56.5 |
Id | 25–34 | 345 ± 149* | 474 ± 183*,† | 5.6 ± 1.7 | 4.2 ± 1.1† | 3.8 ± 1.7* | 6.3 ± 4.1*,† | 69.4 ± 60.9 |
IdB6F2 | 27–46 | 555 ± 211 | 481 ± 180* | 5.9 ± 1.9 | 3.9 ± 1.6† | 4.9 ± 2.8* | 8.9 ± 6.5† | 51.0 ± 27.6 |
IdDCF2 | 26–39 | 557 ± 188 | 439 ± 105*,† | 3.8 ± 1.8* | 5.9 ± 2.1*,† | 10.6 ± 10.1 | 12.2 ± 10.6 | 32.0 ± 12.0 |
Ma | 21–36 | 377 ± 110* | 581 ± 235† | 3.3 ± 1.1* | 2.4 ± 0.7* | 11.1 ± 9.4 | 11.4 ± 9.6 | 57.3 ± 34.2 |
MaB6F2 | 21–36 | 453 ± 164* | 625 ± 225† | 3.5 ± 1.3* | 2.7 ± 1.2* | 7.0 ± 7.4 | 8.4 ± 6.4 | 42.7 ± 24.2 |
All values given are mean ± standard deviation.
IGF-I = insulin-like growth factor I; DC = diversity control; Id = North-Central Idaho; Ma = South Pacific Island of Majuro.
In addition, both Ma and MaB6F2 mice were hypothyroid relative to each of the other stocks at both 6 (p < .0001) and 14 months (p < .001). Interestingly, T4 levels declined with age in all but the IdDCF2 mice, and were significantly lower at 14 months in the DC, Id, and IdB6F2 mice (p < .03). In contrast, serum leptin and fecal corticosterone levels differed little among stocks or with age except that Id mice had significantly lower leptin levels (p < .02) relative to the DC mice. However, there is clearly a high level of variation in these measures within most of the stocks, which may obscure differences in mean levels among stocks.
Hormones and Life History Traits as Predictors of Life Span
Because these mouse stocks differ greatly in size, maturation rate, and hormone levels in addition to longevity, strain effects would seriously confound standard regression methods testing the dependence of life span on predictor variables. Therefore, we tested the relationship between predictor variables and life span using a bivariate regression model, whereby each of the phenotypic measures was used as a continuous covariate and mouse stock was used as a categorical covariate. Initially, a full factorial model was constructed and evaluated, and if the interaction term (i.e., life history or hormone measure × mouse stock) was not significant (p > .05), a second regression model was constructed that used only the continuous and categorical variables as simultaneous predictors of life span. The results of these regression analyses are summarized in Table 5. Mouse life span was predicted to a significant degree by age at vaginal patency, and by body weight at 2, 3, or 4 months after controlling for the effect of mouse stock. We also noted a relationship between life span and the 6-month IGF-I and 14-month leptin level that did not quite meet our prespecified significance criterion (p ≤ .065). Figure 3 illustrates these associations in the form of scatterplots. For the age at vaginal patency, examination of the scatterplot would suggest that Id mice are largely responsible for the ability of this trait to act as a predictor of life span; however age at vaginal patency remains a significant predictor of life span even after removing the data for the Id mice from the model (p = .048). Thus, long life span is associated with slower reproductive maturation, lower body weight, and perhaps lower IGF-I and higher leptin levels, even after adjustment for stock among these groups of mice.
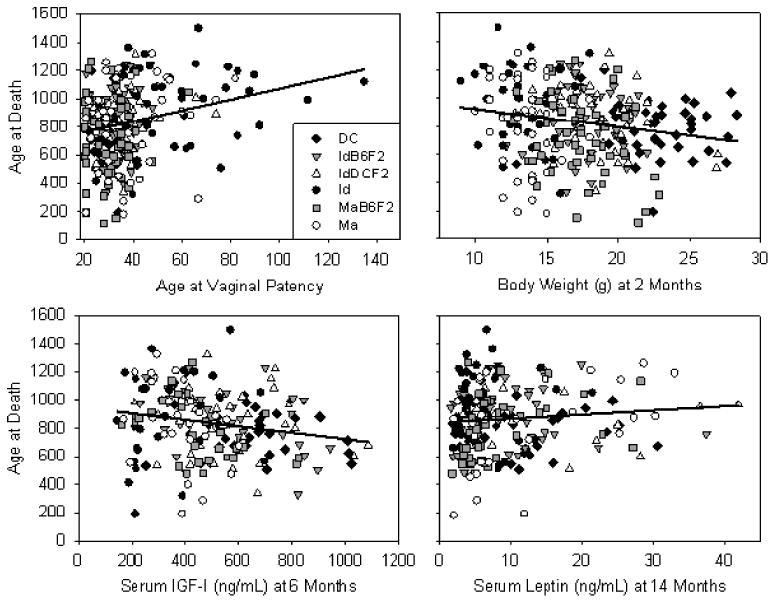
Life span as a function of: age at vaginal patency (top left); 2 month body weight (top right); serum insulin-like growth factor-I (IGF-I) at 6 months (bottom left); and leptin at 14 months (bottom right). See Table 5 for regression summary. Data for individual mouse stocks are as indicated in the legend in the top left panel. Each point represents an individual mouse. Regression lines were calculated by least-squares regression for all mouse stocks combined.
Table 5
Life History Traits and Hormone Levels as Predictors of Life Span
Bivariate Regression | |||||
---|---|---|---|---|---|
Trait | Age (Months) | N | p(Stock)* | p(Trait) | p(I) |
Life history | |||||
![]() | — | 261 | .11 | .02† | |
![]() | 6 | 255 | .10 | .16 | |
![]() | — | 261 | .005† | .13 | |
![]() | 1 | 260 | .006† | .27 | |
2 | 261 | .005† | .01† | ||
3 | 260 | .004† | .02† | ||
4 | 260 | .01† | .02† | ||
6 | 255 | .04† | .10 | ||
Hormone | |||||
![]() | 6 | 191 | .40 | .065‡ | |
14 | 222 | .21 | .34 | .05† | |
![]() | 6 | 165 | .18 | .12 | |
14 | 213 | <.01† | .06‡ |
Interestingly, the strength of the association between body weight and life span steadily decreased with increasing age (Figure 4), such that by 24 months of age the partial regression coefficient (β) for the effect of body weight was close to zero. In addition, mouse stock was a significant predictor of life span after controlling for body weight at any age tested (1–28 months), or after adjustment for rate of growth or for leptin levels in 14-month-old mice (Table 5).
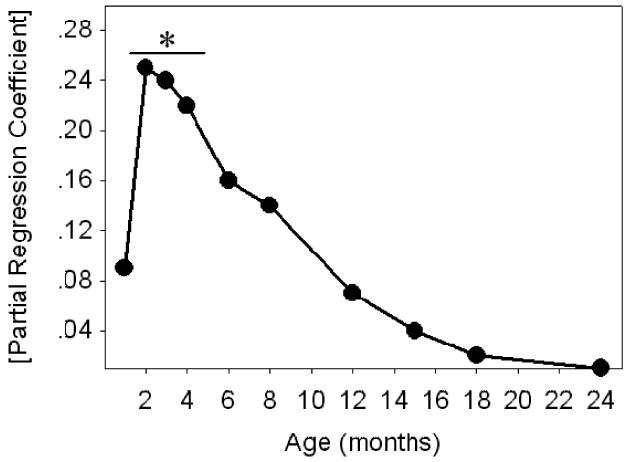
The association between life span and body weight declines with increasing age. Values on the y-axis are absolute partial correlation coefficients for body weight as a predictor of life span generated by bivariate linear regression as described. *Body weight is a statistically significant predictor of life span at each of the indicated ages (p < .03).
In 14-month-old mice, the interaction term for the full factorial model involving serum IGF-I level and strain was significant (p = .05), although there were no significant effects for either stock or IGF-I level. Figure 5 shows the scatterplot for IGF-I levels in 14-month-old mice against life span, and it is clear from the regression lines that the direction of the association may vary among the different stocks. Although the numbers of mice tested provides only limited statistical power, examination of Figure 5 suggests that higher mid-life IGF-I values may be associated with longer life spans in Id and IdDCF2 mice, but with shorter life spans in MaB6F2 and DC mice.
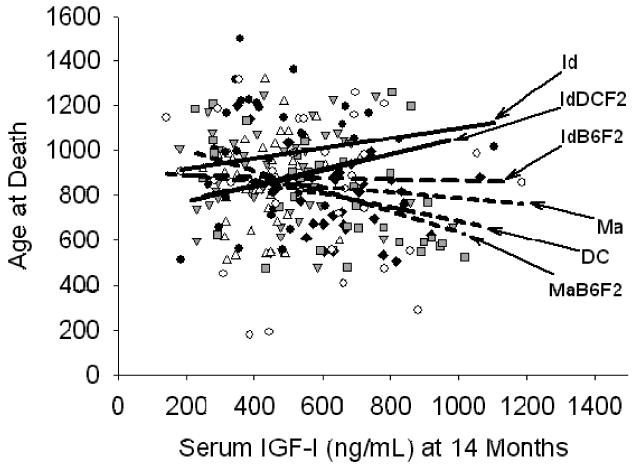
Life span as a function of serum insulin-like growth factor-I (IGF-I) at 14 months. Data for individual mouse stocks are as indicated in Figure 3. Regression lines were calculated for each stock separately by least squares regression. Dashed regression lines indicate a negative association between IGF-I and life span in DC, IdB6F2, Ma, and MaB6F2 mice. Solid regression lines indicate a positive association between IGF-I and life span in Id and IdDCF2 mice. See text for details.
We also evaluated the ability of each of these measures to predict longevity within each stock. Again, the small numbers of animals available (N ~ 40–50 per stock) did not provide a great deal of statistical power. Nonetheless, IGF-I measured at 6 months of age was a significant predictor of life span in both the IdB6F2 and MaB6F2 mice. In each case, mice with low levels of IGF-I at this young adult age lived longer than those mice with higher IGF-I levels. Scatterplots illustrating these relationships are shown in Figure 6.
Covariation of Life Span and Phenotypic Measures With Body Weight
Because many traits vary systematically with body weight, regression analyses that include stocks or species of differing weight are subject to potential confounding. Speakman (16) has emphasized the importance of considering this allometric relationship in analyses among species, in which large size is typically associated with longer life span. It seems possible that similar effects might apply to comparisons, within a species, of stocks that differ in body weight as well as life span. Indeed, this is underscored by the significant ability of stock to act as a predictor of life span in this study, regardless of age, after adjusting for the effect of body weight (Table 5). Therefore a separate set of regression models was constructed to determine whether the residuals of early-life phenotypes (adjusted for body weight) could be used to predict the residual of life span (again adjusted for weight) for each of the traits listed in Table 5. Using simple linear regression we found significant relationships for the age at vaginal patency (r2 = 0.03, p = .003; data not shown) and serum IGF-I and leptin levels at 6 and 14 months (Figure 7). Each of these associations was also significant by Spearman-Rank correlation: age at vaginal patency (R = 0.19, p = .002); IGF-I (6 months, R = −0.15, p = .04; 14 months, R = −0.15, p = .02); and leptin (6 months, R = 0.18, p = .02; 14 months, R = 0.27, p < .0001). Thus even after adjustment for body weight, mice with slow maturation, low IGF-I, and high leptin levels (at 6 or 14 months of age) tend to survive relatively longer than mice with the opposite set of characteristics. This pattern is broadly consistent with the results of the bivariate linear regression modeling described above.
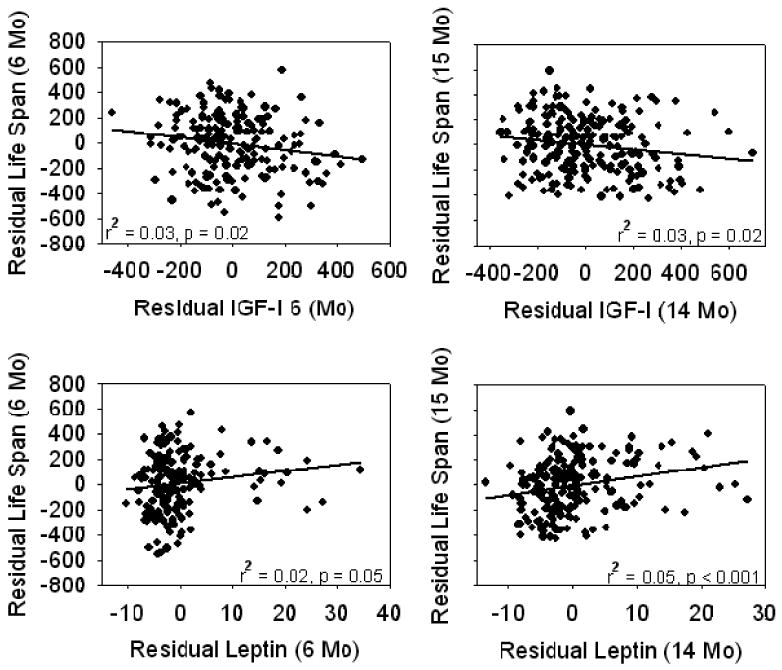
Residuals of life span as a function of serum insulin-like growth factor-I (IGF-I) and leptin levels after adjustment for the effect of body weight at 6 months (6-month measures) or 14 months (14-month measures). Each point represents a single mouse. Each relationship is statistically significant as indicated.
Discussion
This study had several objectives. First, we wanted to replicate our earlier finding that wild-derived mouse stocks are long-lived relative to a laboratory control stock (3), as well as to document differences, among these stocks, in traits believed to be important in life-span regulation. Second, we wanted to test the hypothesis that specific hormonal and developmental traits, measured in young adult mice, could act as significant predictors of life span in mouse stocks in which wild-derived and laboratory-derived genetic alleles were segregating.
In our previous study (3) we demonstrated that the descendants of wild-caught progenitors (Id, Ma) were significantly longer-lived than a laboratory-derived (DC) control stock. The present study replicates this earlier finding, and shows further that F2 hybrid stocks between Id mice and an inbred (B6) laboratory stock, and Id mice and a genetically heterogeneous control stock (DC), have survival curves that are intermediate between the Id and laboratory-derived parental stocks. We selected the DC stock as our representative laboratory control, rather than evaluating inbred B6 mice themselves, to provide an appropriate degree of genetic heterogeneity; direct comparison with B6 mice would have been less informative, because of the relatively short life span seen in inbred compared to non-inbred mice (17). Gene mapping experiments are now under way to map the loci that contribute to differences in longevity between the Id mice and those of laboratory stocks.
Consistent with the earlier report, the current study also showed significantly higher mean and maximal life span in the Ma mice relative to DC controls. In addition, the survival curve (Figure 1, bottom) suggests that, as in the previous study, Ma mice have a higher mortality risk in the first third of the life span and a lower risk thereafter, with a crossover point between 800 and 900 days with respect to DC (3).
In addition to being long-lived, each of the wild-derived stocks was significantly slower to reach sexual maturation, grew more slowly, and was smaller and leaner than the DC stock (Table 2; Figure 2). Although associations between maturation rate and life span are well-documented in invertebrates (9,10,18), relationships between life span and early adult development are less well characterized in mammals, although within natural populations the age at sexual maturity is related to life span (19). Our results show a significant positive relationship between life span and the age of vaginal patency in the study populations even after controlling for interstock variation (Table 5; Figure 3) and the influence of body weight (Figure 7), a significant predictor of the onset of puberty in a number of mammalian species (20). We cannot, without further work, distinguish between two classes of hypotheses: (i) the causal claim that slow reproductive maturation leads to delayed aging, and (ii) the alternate idea that both rate of reproductive maturation and rate of aging are modulated by some underlying factor. We think it plausible that both maturation and aging might be influenced by the timing and amplitude of nutrient flux and/or hormonal factors, and are now conducting interventional studies along these lines.
Within mammal species there tends to be an inverse correlation between body size and life span (21). For example, small body size at as early as 2 months of age (22) and artificial selection for reduced growth rates in early life (4) are both associated with a significantly longer life span in mice. Increased longevity is also seen in growth-retarded mice harboring mutations in the growth hormone (GH)/IGF axis, including Snell and Ames dwarf mice (7,23), GH-receptor knock out (GHRKO) mice (24), and mice with a defect in GH-releasing hormone receptor (GHRHR) signaling (23). This phenomenon is also well known in domestic dog breeds (25), and perhaps in humans as well (26). Consistent with these data, low body weight was associated with increased life span in our current study; this relationship, however, was only significant with weights measured during early adulthood (i.e., 2–4 months old). With increasing age (≥ 6 months), the strength of the association between body weight and life span steadily declined such that the regression coefficient was close to zero by 24 months of age (Figure 4). This reduction in the ability of body weight to act as a predictor of life span parallels the results of an earlier study using a genetically heterogeneous stock of mice (22). Overall, these data suggest that body weight can act as a measure of events in early life that can have a significant impact on the development and progression of late-life disease, and ultimately life span. The next challenge is to discover the mechanisms underlying these differences in weight gain, which are likely to include genetic modifiers of specific hormones, such as IGF-I, and their associated signaling pathways.
In the rodent, IGF-I has multiple effects on postnatal growth and development (27), and several lines of evidence strongly suggest that a reduction in IGF-I action is central to life-span extension in invertebrate species as well. For example, mutations that result in deficiencies in IGF signaling pathways in Caenorhabitis elegans (5) and Drosophila melanogaster (6) dramatically increase life span. We have listed above the various mouse mutations in which low IGF-I action is associated with extended life span (7,23,24), and note also that a significant reduction of early-life IGF-I levels are seen in both of the dietary manipulations known to extend rodent longevity (28,29). Life span is also significantly increased in female mice heterozygous for a knock-down mutation of the IGF-I receptor (IGF-1R) (8), as well as in transgenic mice overexpressing the klotho protein, recently demonstrated to be an inhibitor of IGF-I action (30).
In this study, the 6-month IGF-I level was 46% and 42% lower in the long-lived Id and Ma stocks, respectively, in comparison to the DC stock (Table 4). IGF-I remained at a lower level at 14 months of age in both stocks (Id, 37%; Ma, 12%); however, this difference was not significant in the Ma mice. There was also a suggestive inverse relationship between 6-month serum IGF-I and life span after adjusting for the effect of stock (Table 5; Figure 3) or body weight (Figure 7). The relationship between 14-month IGF-I and life span seemed to vary from stock to stock using the bivariate regression approach (Table 5, Figure 6); in contrast, regression analyses based on residuals after adjustment for body weight suggested a significant inverse relationship between the IGF-I level at 14 months and life span in the pooled data set, similar to that seen using IGF-I data from 6-month-old mice. Hence, although these data are somewhat ambiguous, they do support the idea that the modulation of IGF-I levels in early life may be important in life-span regulation.
We also noted that young and middle-aged Ma mice were hypothyroid relative to both the Id and DC stocks. In rats, lifelong hypothyroidism has been associated with life-span extension in individuals of both sexes (31,32), and dietary supplementation of T4 during early life shortens life span in rats (32) and can blunt the life-extending effect of the Pit1 mutation in Snell dwarf mice (33). Hence, these data suggest that hypothyroidism in Ma mice may contribute to their increased life span, although the mechanism behind this effect remains unknown.
Little is known about the effect of leptin on life-span regulation, although it has been proposed that lower serum leptin levels promote increased life span in rodents (34). Indeed, in an earlier study we reported a significant inverse relationship between serum leptin levels and life expectancy in 4-month-old genetically heterogeneous female mice (35), and in the current study 6-month leptin levels were relatively low in Id and IdB6F2 mice, both of which were long-lived compared to the DC controls (Table 4). Nonetheless, bivariate linear regression suggested, in contrast, a positive association between leptin levels and life span in 14-month-old mice in this study (Table 5; Figure 3). Moreover, weight-adjusted regression models demonstrated a statistically significant relationship for both 6-month and 14-month serum leptin levels (Figure 7). At present, the reason for these contradictory results is unknown, and will require a more thorough study of the interactions of age, food intake, adiposity, and leptin-dependent signaling pathways in these stocks of mice.
Our previous study showed that Id and Ma mice differ from laboratory-derived DC mice in rate of maturation, body size, and multiple hormones, in addition to longevity, and our current data set confirms these observations and provides data on middle-aged mice of each stock. Co-occurrence of a set of traits among stocks can represent either coincidence or the outcome of a set of causal relationships based on pleiotropic effects of polymorphic alleles between wild and laboratory stocks. As a first step towards genetic analysis, we evaluated the level of these traits in F2 hybrid mice, and found that nearly all of the traits, including life span, assumed intermediate levels in the hybrids between Id and laboratory stocks. This result suggests that the traits in question are modulated by one or more codominant loci that distinguish Id mice from the laboratory stocks used, and give a rationale for a formal quantitative trait locus mapping study now in progress in the IdB6F2 model.
The availability of F2 mice, in which alleles from laboratory- and wild-derived stocks are cosegregating, provided a basis for testing the ability of early-life traits to predict life span in individual animals. Serum IGF-I levels, measured at 6 months, were significant predictors of life span in both the IdB6F2 and the MaB6F2 stocks (Figure 4), providing further support for the idea that early-life IGF-I is important in the determination of life span. In the MaB6F2 population, life span was predicted by body weight at 1 and 2 months of age, as well as 14-month serum IGF-I and leptin levels (data not shown), but these associations did not reach significance in the IdB6F2 mice, so we remain uncertain about the generality of the conclusion.
Most biomedical research on rodents is conducted in domesticated, highly inbred stocks of rats and mice, or in their isogenic F1 hybrids. These mouse stocks have experienced many generations of selective pressures for genetic alleles associated with high fecundity, rapid growth, use of invariably high food supplies, significantly altered behavioral patterns, and a predisposition to the development of neoplastic disease, creating experimental animals that differ in many respects from their wild-living ancestors. In addition to its implications for studies of behavior, nutrition, and development, this domestication process may to some extent impair the suitability of laboratory rodents for studies of aging. This set of selective pressures that mold fitness traits in juvenile and young adult animals is likely to affect aging rates as a consequence of secondary or pleiotropic effects on gerontologically important factors. In particular, genetic loci that modulate body size and the rate of sexual maturation are likely to be important in the adaptation to ecological niches that can afford life history deceleration. Indeed, the significant relationships between maturation rate and life span and between body weight and life span seen in this study strongly support this notion. Our results also suggest that IGF-I signaling during early adult life may be an important factor linking these life history traits to life span.
Acknowledgments
This work was supported by National Institutes of Health grants AG08808, AG13711, and AG023122.
We thank Gretchen Buehner, Maggie Vergara, and Jessica Sewald for their husbandry and technical assistance, and Dr. Andrzej Galecki for statistical advice.
References
Full text links
Read article at publisher's site: https://doi.org/10.1093/gerona/61.10.1019
Read article for free, from open access legal sources, via Unpaywall:
https://academic.oup.com/biomedgerontology/article-pdf/61/10/1019/1636921/1019.pdf
Citations & impact
Impact metrics
Citations of article over time
Article citations
Sex- and age-dependent genetics of longevity in a heterogeneous mouse population.
Science, 377(6614):eabo3191, 30 Sep 2022
Cited by: 27 articles | PMID: 36173858 | PMCID: PMC9905652
Loss of the interaction between estradiol and insulin-like growth factor I in brain endothelial cells associates to changes in mood homeostasis during peri-menopause in mice.
Aging (Albany NY), 11(1):174-184, 01 Jan 2019
Cited by: 6 articles | PMID: 30636168 | PMCID: PMC6339786
Reduced growth hormone signaling and methionine restriction: interventions that improve metabolic health and extend life span.
Ann N Y Acad Sci, 1363:40-49, 08 Dec 2015
Cited by: 7 articles | PMID: 26645136 | PMCID: PMC6472264
Review Free full text in Europe PMC
Mouse models of ageing and their relevance to disease.
Mech Ageing Dev, 160:41-53, 04 Oct 2016
Cited by: 58 articles | PMID: 27717883
Review
Nutritional limitation in early postnatal life and its effect on aging and longevity in rodents.
Exp Gerontol, 86:84-89, 07 May 2016
Cited by: 5 articles | PMID: 27167581
Review
Go to all (24) article citations
Similar Articles
To arrive at the top five similar articles we use a word-weighted algorithm to compare words from the Title and Abstract of each citation.
Longer life spans and delayed maturation in wild-derived mice.
Exp Biol Med (Maywood), 227(7):500-508, 01 Jul 2002
Cited by: 104 articles | PMID: 12094015
Big mice die young: early life body weight predicts longevity in genetically heterogeneous mice.
Aging Cell, 1(1):22-29, 01 Oct 2002
Cited by: 140 articles | PMID: 12882350
PohnB6F1: a cross of wild and domestic mice that is a new model of extended female reproductive life span.
J Gerontol A Biol Sci Med Sci, 62(11):1187-1198, 01 Nov 2007
Cited by: 24 articles | PMID: 18000137
Exotic mice as models for aging research: polemic and prospectus.
Neurobiol Aging, 20(2):217-231, 01 Mar 1999
Cited by: 38 articles | PMID: 10537031
Review
Funding
Funders who supported this work.
NIA NIH HHS (8)
Grant ID: P60 AG008808
Grant ID: AG13711
Grant ID: U19 AG023122
Grant ID: AG08808
Grant ID: P60 AG008808-149005
Grant ID: R01 AG013711
Grant ID: R01 AG013711-03
Grant ID: AG023122