Abstract
Free full text

Opposite roles of apolipoprotein E in normal brains and in Alzheimer’s disease
Abstract
We have characterized the interaction between apolipoprotein E (apoE) and amyloid β peptide (Aβ) in the soluble fraction of the cerebral cortex of Alzheimer’s disease (AD) and control subjects. Western blot analysis with specific antibodies identified in both groups a complex composed of the full-length apoE and Aβ peptides ending at residues 40 and 42. The apoE–Aβ soluble aggregate is less stable in AD brains than in controls, when treated with the anionic detergent SDS. The complex is present in significantly higher quantity in control than in AD brains, whereas in the insoluble fraction an inverse correlation has previously been reported. Moreover, in the AD subjects the Aβ bound to apoE is more sensitive to protease digestion than is the unbound Aβ. Taken together, our results indicate that in normal brains apoE efficiently binds and sequesters Aβ, preventing its aggregation. In AD, the impaired apoE–Aβ binding leads to the critical accumulation of Aβ, facilitating plaque formation.
Apolipoprotein E (apoE) is a polymorphic plasma glycoprotein that binds cholesterol and other lipids, mediating their transport into the cell (1, 2). In brain, apoE is expressed mainly in glial cells, and it is thought to be implicated in neuronal regeneration (3, 4). Although the allele of apoE isoform 4 (apoE 4) is strongly associated with late-onset familial and sporadic Alzheimer’s disease (AD) (5, 6), the role of apoE in the pathogenesis of AD is still unclear. The presence of apoE in amyloid plaques (7, 8), the positive correlation between amyloid burden and the frequency of allele
4 (9, 10), and the in vitro binding of apoE to the amyloid β-peptide (Aβ) (11), the major component of AD amyloid (12, 13), strongly suggest that apoE influences the rate of cerebral amyloidogenesis. However, it is still debated whether apoE
4 promotes or inhibits the aggregation and polymerization of Aβ (14–16).
We have previously proposed that a water-soluble form of Aβ (sAβ) is an early marker of amyloid formation because it is detectable only in brains of subjects with AD or at risk for AD, such as young individuals with Down’s syndrome who still lack plaques or amyloid deposits, whereas it is undetectable in normal brain tissue (17, 18). These findings point to a mechanism that prevents the accumulation of Aβ under normal conditions. Because of its capability to bind Aβ peptides in vitro and its involvement in AD, apoE is the best candidate to play a role in sequestering and clearing sAβ in brain.
In this study, we investigated the interaction between apoE and Aβ in the soluble fraction of AD and control brains associated with various apoE genotypes.
MATERIALS AND METHODS
Case Selection.
Cerebral cortex was obtained at autopsy from 18 patients with AD [National Institute of Neurological and Communicative Disorders and Stroke pathological criteria (19)] and from 15 non-AD patients with other neurological (anoxic encephalopathy, telencephalic leukoencephalopathy, Huntington’s disease, seizure disorder, multiple infarcts, multisystemic atrophy, Parkinson’s disease) and nonneurological conditions (cardiomyopathy, liver degeneration, diabetes mellitus, bronchopneumonia, heroin intoxication, leukemia) in which the presence of amyloid deposits had been excluded by immunocytochemical analysis performed on sections obtained from the tissue sample used for the biochemical study. ApoE genotyping was carried out according to previously described methods (20). The genotypes for AD cases were as follows: 4/
4 = 3;
3/
4 = 6;
3/
3 = 9, and for control brains:
3/
4 = 6;
3/
3 = 6;
2/
3 = 3.
Antibodies.
Immunodetection was carried out with the monoclonal antibodies 6E10 (21) and 4G8 (21) specific for an Aβ epitope between residues 6–10 and 17–21 (Senetek, Napa, CA); the two polyclonal antibodies specific for the Ala-42 (αAβ42) or the Val-40 (αAβ40) residues of Aβ were purchased from Quality Controlled Biochemicals (Hopkinton, MA); the monoclonal antibody MAB1062 and the polyclonal antibody AB947 to apoE, the latter used in immunoprecipitation, were both from Chemicon. The polyclonal antibody R3660 specific for the amino-terminal region of Aβ was previously described (22). The working dilution for all the antibodies used in detection was 1:1000. The reactivity on Western blots was visualized with the enhanced chemiluminescence system (ECL; Amersham). All the chemicals were from Sigma unless otherwise specified.
Isolation and Quantification of Soluble Aβ, apoE, and apoE–Aβ Complexes.
The preparation of soluble brain fractions from brain homogenates, followed by immunoprecipitation, electrophoresis, Western blotting, and densitometric quantification, was carried out as previously described (18, 22). We used 8 μl/ml of the antibody AB947 for the immunoprecipitation of apoE after clearing the crude brain extracts from interfering immunoglobulins with a protein G/A mixture. All the samples were analyzed at least in triplicate for quantitative purposes.
In Vitro apoE–Aβ Complex.
Two different types of artificial aggregates were prepared: 600 ng of human plasma apoE (Calbiochem) was incubated at room temperature for 3 hr with 100 μM Aβ-(1–42) synthetic peptide (Bachem) in PBS, pH 7.4. Alternatively, 1 μg of human plasma apoE (Calbiochem) was incubated for 3 days at 37°C with the Aβ peptides purified by gel filtration (see below) containing the Aβ peptides released from the apoE–Aβ complex.
Chromatography.
Eight grams of gray matter as starting material from AD and non-AD subjects was processed as described before (18, 22) to obtain the soluble fraction. After immunoprecipitation of apoE and apoE–Aβ complexes with the antibody AB947 (8 μl/ml) the purified proteins were dissociated from the agarose beads with 1 M glycine at pH 2, or with 1% SDS and 10-min boiling followed by precipitation with cold acetone. The samples were then applied on a HW50F (TosoHaas, Montgomeryville, PA) column equilibrated with 0.1 M formic acid/ammonium hydroxide, pH 7.8/30% (vol/vol) acetonitrile. The column was calibrated with standard proteins and the fractions were eluted at 0.8 ml/min, monitored by absorbance at 280 nm, and analyzed by dot-blotting using the monoclonal antibody to apoE, MAB1062, and the polyclonal antibody specific for Aβ40, αAβ40, as detecting antibodies. Human plasma apoE (Calbiochem) and synthetic Aβ-(1–40) were used as standard peptides.
Matrix-Assisted Laser Desorption Ionization Time-of-Flight (MALDI-TOF) Mass Spectrometry.
After the gel filtration, the fractions corresponding to Aβ peptides released from the apoE–Aβ complex were pooled, dried under reduced pressure, and resuspended in 50 μl of water. Two microliters of the resulting solution was incubated with 2 μl of a freshly prepared matrix consisting of α-cyano-4-hydroxycinnamic acid (Aldrich) dissolved at 10 mg/ml in 0.05 M HCl and acetonitrile/isopropyl alcohol, 6:1.5 (vol/vol). One microliter of the mixture was placed on the sample plate and dried at room temperature. The analysis was performed in linear positive mode on a Voyager (PerSeptive Biosystems) mass spectrometer, and 250 scans were averaged.
Protease Digestion.
Brain homogenates from AD subjects with an equal protein concentration (measured with the Bradford method), were treated with proteinase K (Boehringer Mannheim) at 37°C for 1 hr. The digestion was terminated by adding phenylmethylsulfonyl fluoride at 3 mM final concentration. The homogenates were then ultracentrifuged at 100,000 × g for 1 hr, and the resulting supernatants were immunoprecipitated by using the antibodies AB947 to apoE and R3660 specific for Aβ peptides. The latter does not recognize the apoE–Aβ complex when used in immunoprecipitation. Immunoblotting with 6E10 (specific for residues 6–10 of Aβ) as detecting antibody was then carried out. Homogenates from the same subjects without protease digestion were processed in parallel.
RESULTS
Immunoprecipitation of Aβ peptides from soluble brain fractions with the polyclonal antibody R3660 to Aβ, followed by immunoblotting and detection with the monoclonal antibody 6E10 (21) (specific for an epitope between residues 6 and 10 in the Aβ sequence), demonstrated two bands of 4.5 kDa and 4.2 kDa in all AD subjects, but not in controls (Fig. (Fig.1).1). These two bands have recently been shown to contain predominantly Aβ-(1–40/42) and pyroglutamate-modified (p) Aβ-(p3–42) (22, 23). A third Aβ band, detected with the monoclonal antibody 4G8 (21) (specific for residues 17–21 of Aβ), contains mostly Aβ-(11–40/42) and pyroglutamate-modified Aβ-(p11–40/42) (22).
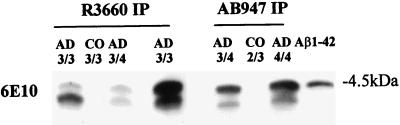
Soluble Aβ is recovered after immunoprecipitation with antibodies to apoE or Aβ in AD brains. Both polyclonal antibodies R3660 (specific for the amino-terminal portion of Aβ) and AB947 (to human apoE) immunoprecipitate (IP) Aβ peptides detected as two bands of 4.5 and 4.2 kDa after immunostaining with the Aβ monoclonal antibody 6E10 in AD brains only. Control (CO) brains are not immunoreactive irrespective of their apoE genotype (3/3, etc.). The presence of Aβ peptides immunoprecipitated with the antibody AB947, specific to apoE, indicates that a portion of the soluble Aβ is bound to apoE and separates in SDS/PAGE. The synthetic peptide Aβ-(1–42) comigrates with the upper band in AD samples.
The same 4.5- and 4.2-kDa Aβ bands were observed only in AD preparations, and not in control subjects, when the immunoprecipitation was carried out with AB947, the antiserum specific for human apoE, and again the monoclonal antibody 6E10 was used for immunoblot detection (Fig. (Fig.1).1). Moreover, samples that had been cleared of unbound soluble Aβ by immunoprecipitation with the antibody R3660 still revealed the presence of Aβ bands in all the AD subjects in the second immunoprecipitation with the antibody AB947, which is specific for the apoE molecule. Combined, these findings suggest that in AD brains a portion of the water-soluble Aβ is bound to apoE and released after treatment with SDS prior to PAGE.
Immunoprecipitation of brain proteins with the apoE antiserum AB947, followed by immunoblotting with the monoclonal antibody MAB1062 to human apoE, demonstrated a broad band in all cases, which is significantly reduced in the AD samples, and whose lower portion comigrated with human apoE (Fig. (Fig.22a). Immunodetection with different antibodies specific for various Aβ regions: the monoclonal antibodies 6E10 (Fig. (Fig.22b) and 4G8 (not shown), and the polyclonal antibody αAβ42 (specific for the carboxyl-terminal Ala in the Aβ sequence, Fig. Fig.33a) revealed a ≈40-kDa band, present in both AD and control preparations, which corresponds to the upper part of the band detected also with the MAB1062 antibody to apoE (Figs. (Figs.22a and and33b). This band comigrated with a complex obtained in vitro by incubating human apoE and the Aβ peptides released from apoE after immunoprecipitation and gel filtration (Fig. (Fig.22b, XX), as well as with another in vitro complex formed by human apoE and synthetic Aβ-(1–42) (not shown). The same MAB1062-reacting complex was also obtained by immunoprecipitating the soluble fraction with the monoclonal antibody 4G8, specific for an epitope between residues 17 and 21 in the Aβ sequence (Fig. (Fig.33b). The gel mobility, the reactivity with various antibodies specific for both proteins, and the comigration with aggregates formed in vitro indicate that the ≈40-kDa band corresponds to a complex formed by the full-length apoE and the water-soluble Aβx-42. Moreover, the aggregate isolated from control cases is completely SDS resistant (Fig. (Fig.4a),4a), whereas in the AD brain extracts the Aβ peptides are easily released from the complex (Figs. (Figs.11 and and44a). The reactivity of the aggregate with the anti-apoE antibody MAB1062 was 40% lower in soluble preparations from AD brains than in control cases (P < 0.005) (Fig. (Fig.44b). No significant differences among apoE genotypes were found, except for a lower reactivity in AD subjects homozygous 4 (Figs. (Figs.22 and and44a).
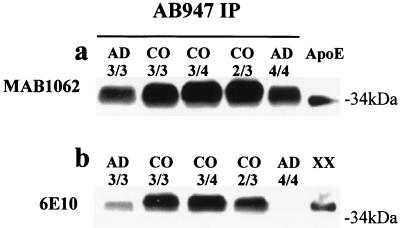
Identification of the apoE–Aβ aggregate. (a) Human brain soluble fractions immunoprecipitated with the anti-apoE antibody AB947 show a broad band of 34–40 kDa in both AD and control (CO) subjects that is immunodetectable with the monoclonal antibody MAB1062, specific for apoE; the lower portion of this band comigrates with human plasma apoE. Note that the 34- to 40-kDa band is less prominent in AD than in control brains. (b) The same samples immunodetected with the antibody 6E10 (specific for Aβ residues 6–10) show an ≈40-kDa band, corresponding to the upper portion of the band detected with the antibody MAB1062, that comigrates with an apoE–Aβ complex formed in vitro (XX) (see Materials and Methods). The ≈40-kDa band is less prominent in all the AD brains, and in the apoE 4 homozygous (4/4) subject it is visible only after a prolonged exposure (not shown).

Reactivity of the apoE–Aβ aggregate with different antibodies to Aβ. (a) The ≈40-kDa aggregate is detected with the antibody αAβ42 (specific for residue 42 of the Aβ sequence) after immunoprecipitation with the apoE antibody AB947. As expected, the Aβ antibody does not recognize human plasma apoE. (b) The ≈40-kDa band is also detected after immunoprecipitation with the monoclonal antibody 4G8 (specific for residues 17–21 of Aβ) and immunodetection with the antibody MAB1062 to apoE. This band comigrates with the aggregate observed after immunoprecipitation with the AB947 antibody to apoE.
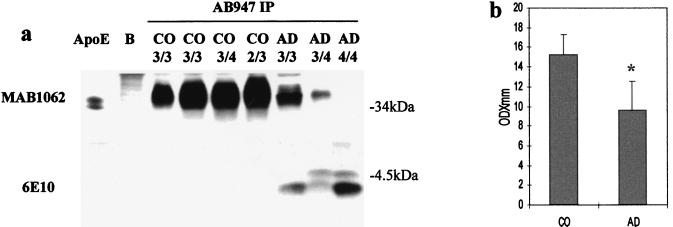
Release of Aβ from the aggregate with apoE in AD brains. (a) Immunoprecipitation with the apoE antibody AB947 and immunodetection with the monoclonal anti-apoE antibody MAB1062 show the 34- to 40-kDa band as in Fig. Fig.22a. This band is underrepresented in AD subjects and in the subject homozygous 4 is visible only after a longer exposure. The polyclonal antibody AB947 alone (B) does not detect any band in the region of the apoE–Aβ complex. The lower portion of the gel, immunostained with the monoclonal anti-Aβ antibody 6E10, reveals the typical Aβ pattern for the AD cases, indicating that a portion of Aβ peptides is released from the complex with apoE after SDS/PAGE, only in AD samples. (b) Quantification of the 34- to 40-kDa band detected in immunoblotting after immunoprecipitation with the antibody to apoE AB947 demonstrates a 40% lower reactivity in AD than in control (CO) subjects.
, P < 0.005.
Immunoprecipitation of soluble brain fractions with the apoE antibody AB947, followed by gel filtration, led to the separation of an high molecular mass protein fraction that immunoreacted in dot-blot analysis with the anti-apoE antibody MAB1062, and with the antibody αAβ40, which is specific for the carboxyl-terminal Val-40 of Aβ, in both AD and control tissues (Fig. (Fig.55a). This finding is consistent with the presence of high molecular mass aggregates (24, 25) in addition to the monomeric aggregate present in the ≈40-kDa region (Fig. (Fig.5,5, fractions 6 and 7) equally immunoreactive with both antibodies. Moreover, the reactivity with the antibodies αAβ40 and αAβ42 (Fig. (Fig.33a) indicates that both Aβ40 and Aβ42 aggregate with apoE. In contrast, the fractions below a molecular mass of 8 kDa (Fig. (Fig.5,5, fractions 10–12) react with the antibody αAβ40 in AD cases only. To verify whether the instability of the complex in AD brains was reversible in vitro and whether, in our conditions, the instability was because of an impaired binding capacity of apoE, these fractions were incubated with human plasma apoE to produce an in vitro aggregate that would mimic the complex purified from the brain. In SDS/PAGE this in vitro complex was resistant to the detergent treatment and comigrated at ≈40 kDa with the apoE–Aβ aggregates extracted from both AD and control brains (Fig. (Fig.22b, XX). To further investigate the composition of the pool of Aβ bound to apoE, the same fractions purified in gel filtration were analyzed by MALDI-TOF mass spectrometry. This analysis demonstrated a substantial amino- and carboxyl-terminal heterogeneity of Aβ peptides (Fig. (Fig.55b), with a similar pattern for the apoE 3 and
4 homozygous AD subjects. No Aβ peptides were detected in the brain extracts purified from non-AD subjects. To clarify whether the Aβ bound to apoE and the unbound pool of water-soluble Aβ could be characterized and differentiated also on the basis of their resistance to proteases, we treated the AD brain homogenates with proteinase K. The Aβ peptides present in the complex with apoE (Fig. (Fig.6,6, white bars) were completely digested when treated with proteinase K at 50 μg/ml, whereas the unbound, soluble Aβ (Fig. (Fig.6,6, gray bars) was resistant to proteinase K at 300 μg/ml.
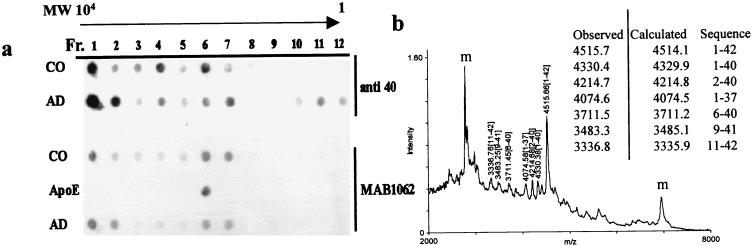
Gel filtration and mass spectrometric analysis of Aβ peptides released from the apoE–Aβ complex. (a) Dot-blot analysis of the fractions collected after gel filtration of brain soluble preparation immunoprecipitated with the AB947 antibody to apoE from AD and control (CO) subjects. The immunodetection was carried out with the antibodies MAB1062 (to apoE) and αAβ40 (to Aβ40). Both antibodies detected reactive proteins in the high molecular mass region (fractions 1 and 2) as well as in the 30- to 40-kDa region (fractions 6 and 7), the latter co-eluting with human plasma apoE. The antibody αAβ40 detects positive fractions also in the low molecular mass region (fractions 10–12) only in AD preparations; these fractions correspond to the Aβ peptides released from the complex with apoE. (b) After immunoprecipitation with AB947 (to apoE) and gel filtration on a HW50 column the fractions 10–12 from AD and control subjects were analyzed by MALDI-TOF mass spectrometry. The AD samples reveal various carboxyl- and amino-terminally truncated Aβ peptides, whereas in control brains no Aβ peptides are detectable (data not shown). The Aβ peaks are identified by their size in Da, while “m” identifies nonspecific signals due to the matrix.
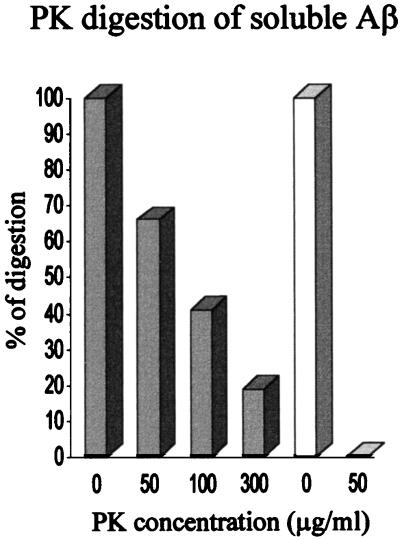
Proteinase K treatment of free and apoE-bound soluble Aβ in AD subjects. The total brain homogenate from AD subjects was treated with increasing concentrations of proteinase K (PK) for 1 hr at 37°C. The soluble fraction was then analyzed in SDS/PAGE. The unbound pool of Aβ (gray bars) and the apoE-bound Aβ (white bars), detected and quantified with the monoclonal antibody 6E10, show different sensitivities to proteinase K. The Aβ present in the complex with apoE is completely digested after treatment with proteinase K at 50 μg/ml, whereas the unbound soluble Aβ is resistant to proteinase K at concentrations up to 300 μg/ml.
DISCUSSION
This study demonstrates that Aβ is bound to apoE, with which it forms a soluble complex of approximately 40 kDa present in both AD and control brains. The Aβ present in plaque-free control brains was found to be entirely bound to apoE in soluble complexes that are resistant to dissociation when treated with SDS. Therefore, it is likely that the undetectability of water-soluble Aβ peptides in normal brains originally reported by us (17) is due to their binding and sequestration by apoE. The higher sensitivity to proteinase K of the Aβ pool complexed with apoE suggests that apoE maintains the Aβ peptides in a soluble aggregate that is sensitive to proteases (26) or might be removed by cell receptors as previously suggested (27). Surprisingly, when Aβ peptides are not bound to apoE, they are more resistant to the proteolytic activity. This finding implies that apoE facilitates the degradation of Aβ peptides and that the unbound Aβ, although soluble, is in an aggregated state. Nevertheless, we cannot rule out the possibility that another molecule(s) protects the soluble Aβ from the protease action when it is not bound to the apoE.
In contrast, in AD brains most of the Aβ peptides that are bound to apoE are released by SDS treatment (Figs. (Figs.11 and and44a). Consistent with this finding, the apoE–Aβ stable complexes are present in significantly lesser amount in soluble extracts of AD brains than in controls. This result is in agreement with a previous study (24), which has demonstrated a reversed equilibrium between soluble and insoluble Aβ–apoE complexes in AD brain. The unstable binding between the two molecules might increase the Aβ concentration and trigger the accumulation of Aβ up to the critical point of nucleation (28) and subsequent polymerization. Polymerized Aβ would also interact with and incorporate the apoE–Aβ complex. At this stage, most of the apoE–Aβ aggregate would shift to the insoluble fraction and participate in the formation of amyloid fibers (7, 8, 24). The above-mentioned high levels of insoluble apoE–Aβ complexes in AD (24), their low level in control subjects, and the direct identification of apoE in amyloid fibers (6, 24) argue in favor of this hypothesis. An opposite role of apoE in normal and pathological conditions is consistent with the apparently discordant data obtained from experiments in vitro in which apoE was shown to inhibit Aβ aggregation at nanomolar (physiological) concentrations of Aβ (16), whereas a reverse effect was observed with micromolar Aβ doses (14). Moreover, the presence of apoE in diffuse plaques in young subjects with Down’s syndrome (29, 30) is consistent with the role of the apoE–Aβ complex in promoting plaque formation. An unbalanced equilibrium between soluble and insoluble complex may have further consequences for neurodegeneration. The engagement of apoE in the formation of amyloid fibrils is likely to affect its overall normal function. A decreased level of soluble apoE has been reported in the frontal cortex of AD subjects (31), and the absence of functional apoE in apoE-knockout mice results in synaptic and dendritic abnormalities (32), learning deficits (33, 34) and decreased levels of choline acetyltransferase (34). These changes can be reverted with the introduction of recombinant apoE (35), which suggests that apoE plays a critical role in the stability of the neural network and processes of memory.
Our hypothesis would also explain the recent finding of the lack of plaque formation in an apoE-knockout transgenic mouse that over-expresses mutant amyloid precursor protein (36). In this model, the lack of apoE would block the formation of the apoE–Aβ complex hindering amyloid deposition.
The stability of the aggregate formed by apoE and Aβ can be reduced by changes in either Aβ or apoE molecules (37–39). The latter possibility is supported by the finding that the Aβ released from the complex with apoE forms an SDS-stable aggregate in vitro with human plasma apoE (Fig. (Fig.22b, XX). The aim of our experiment was to verify if the instability of the complex in AD brains was because of a defective apoE and if the process was reversible (at least in vitro). The apoE used in our experiments is a mixture of the three major human isoforms, and the contribution of each subtype to the reaggregation process is unknown. With reference to previous published work (40) the contribution of the apoE 3 should be more significant. On the other hand, if an impaired binding is the critical step in the failure of apoE in sequestering and clearing Aβ peptides, the genetic predisposition related to the
4 allele might be easily explained by a constitutively weaker affinity of apoE
4 for Aβ. An isoform-specific binding has been described in vitro (11, 27, 40), and a decreased amount of apoE
4 was detected in the plasma and in the cerebrospinal fluid of
4 homozygous subjects (41–43), suggesting that the total apoE
4 content might be insufficient to clear and sequester an increased amount of soluble Aβ. In fact, in our analysis the presence of the complex in the AD
4 homozygous subjects is decreased, although not significantly. However, we did not find any statistically significant variation in the presence, type, and amount of the SDS-stable apoE–Aβ complex in relation to the apoE genotype in control subjects, although control subjects homozygous for the
4 allele were not available for this study. We can only hypothesize that a more relevant effect of the genotype could be present in the early stage of the disease; since our analysis on AD subjects describes the end stage of the pathological process and in the non-AD subjects the pathology never started. Moreover, the mass spectrometric study in the AD subjects indicates that the apoE–Aβ complexes are composed of the entire apoE molecule and different Aβ peptides with both Val-40 and Ala-42 carboxyl termini and that the forms of Aβ peptides released from the complex were identical in AD subjects apoE
3 and
4 homozygous. Taken together, our results indicate that in pathological conditions the apoE–Aβ complex became unstable and insoluble, whereas in normal brains apoE binds and sequesters Aβ, preventing its aggregation.
Acknowledgments
Brain samples used in this study were kindly provided by the University of Miami Brain and Tissue Bank for Developmental Disorders. This represents a joint effort of the University of Miami and the University of Maryland Brain and Tissue Banks through National Institute of Child Health and Human Development Contract N01-HD-33199. This work was supported by National Institutes of Health Grants AG08155 and AG08992, by the Britton Fund, by Telethon (E. 579), and by the North Atlantic Treaty Organization (CRG.940642).
ABBREVIATIONS
apoE | apolipoprotein E |
AD | Alzheimer’s disease |
Aβ | amyloid β-peptide |
MALDI-TOF | matrix-assisted laser desorption ionization time-of-flight |
Footnotes
This paper was submitted directly (Track II) to the Proceedings Office.
References
Articles from Proceedings of the National Academy of Sciences of the United States of America are provided here courtesy of National Academy of Sciences
Full text links
Read article at publisher's site: https://doi.org/10.1073/pnas.95.26.15598
Read article for free, from open access legal sources, via Unpaywall:
https://www.pnas.org/content/pnas/95/26/15598.full.pdf
Citations & impact
Impact metrics
Article citations
Cognitive synaptopathy: synaptic and dendritic spine dysfunction in age-related cognitive disorders.
Front Aging Neurosci, 16:1476909, 03 Oct 2024
Cited by: 0 articles | PMID: 39420927 | PMCID: PMC11484076
Review Free full text in Europe PMC
Temporal Characterization of the Amyloidogenic APPswe/PS1dE9;hAPOE4 Mouse Model of Alzheimer's Disease.
Int J Mol Sci, 25(11):5754, 25 May 2024
Cited by: 1 article | PMID: 38891941 | PMCID: PMC11172317
Apolipoprotein E imbalance in the cerebrospinal fluid of Alzheimer's disease patients.
Alzheimers Res Ther, 14(1):161, 02 Nov 2022
Cited by: 8 articles | PMID: 36324176 | PMCID: PMC9628034
Cerebral Amyloid Angiopathy and Blood-Brain Barrier Dysfunction.
Neuroscientist, 27(6):668-684, 25 Nov 2020
Cited by: 14 articles | PMID: 33238806 | PMCID: PMC9853919
Apolipoprotein E/Amyloid-β Complex Accumulates in Alzheimer Disease Cortical Synapses via Apolipoprotein E Receptors and Is Enhanced by APOE4.
Am J Pathol, 189(8):1621-1636, 17 May 2019
Cited by: 20 articles | PMID: 31108099 | PMCID: PMC6680253
Go to all (56) article citations
Similar Articles
To arrive at the top five similar articles we use a word-weighted algorithm to compare words from the Title and Abstract of each citation.
Quantitation of apoE domains in Alzheimer disease brain suggests a role for apoE in Abeta aggregation.
J Neuropathol Exp Neurol, 60(4):342-349, 01 Apr 2001
Cited by: 63 articles | PMID: 11305869
Tissue transglutaminase-catalysed cross-linking induces Apolipoprotein E multimers inhibiting Apolipoprotein E's protective effects towards amyloid-beta-induced toxicity.
J Neurochem, 134(6):1116-1128, 07 Jul 2015
Cited by: 9 articles | PMID: 26088696
Apolipoprotein E and beta-amyloid levels in the hippocampus and frontal cortex of Alzheimer's disease subjects are disease-related and apolipoprotein E genotype dependent.
Brain Res, 843(1-2):87-94, 01 Oct 1999
Cited by: 86 articles | PMID: 10528114
ApoE and Aβ in Alzheimer's disease: accidental encounters or partners?
Neuron, 81(4):740-754, 01 Feb 2014
Cited by: 342 articles | PMID: 24559670 | PMCID: PMC3983361
Review Free full text in Europe PMC
Funding
Funders who supported this work.
NIA NIH HHS (2)
Grant ID: AG08992
Grant ID: AG08155
NICHD NIH HHS (1)
Grant ID: HD-33199