Abstract
Free full text

Molecular Determinants of Adaptation of Highly Pathogenic Avian Influenza H7N7 Viruses to Efficient Replication in the Human Host
Abstract
Two viruses isolated during the highly pathogenic avian influenza (HPAI) H7N7 virus outbreak in The Netherlands in 2003, one isolated from a person with conjunctivitis and one from a person who died as the result of infection, were used for an in vitro study of influenza A virus pathogenicity factors. The two HPAI H7N7 viruses differed in 15 amino acid positions in five gene segments. Assays were designed to investigate the role of each of these substitutions in attachment and entry, transcription and genome replication, and virus production and release as determined by hemagglutinin (HA), polymerase proteins, nonstructural protein 1 (NS1), and neuraminidase (NA). These in vitro studies confirmed the roles of the E627K substitution in basic polymerase 2 (PB2) and the A143T substitution in HA in pathogenicity observed in a mouse model previously. However, the in vitro studies identified a contribution of acidic polymerase (PA) and NA to the efficient replication in human cells of the fatal case virus, despite the fact that these are rarely marked as determinants of pathogenicity in in vivo studies. With the exception of PB2 E627K, all substitutions contributing to enhanced replication of the fatal case virus in vitro were present in poultry viruses prior to transmission to the human fatal case, indicating that viruses with enhanced replication efficiency in the mammalian host can be generated in poultry. Thus, detailed in vitro analyses of mutations facilitating replication of avian influenza viruses in mammalian cells are important to assess the zoonotic risk posed by these viruses and, in addition, highlight the value of in vitro studies to complement animal models.
Wild aquatic birds form the natural reservoir for avian influenza A viruses (60). When low-pathogenic avian influenza A (LPAI) viruses of the H5 or H7 subtype are transmitted from wild birds to poultry, they may mutate into highly pathogenic avian influenza (HPAI) viruses through the introduction of multiple basic amino acid residues at the hemagglutinin (HA) cleavage site (1, 62). These HPAI viruses can then cause outbreaks in poultry with a mortality of up to 100%. HPAI viruses of both the H5 and H7 subtypes have been transmitted directly from poultry to humans on several occasions, with symptoms ranging from conjunctivitis to fatal respiratory disease. The direct transmission of HPAI viruses into humans leading to fatality was first described in 1997 during an outbreak of HPAI H5N1 virus in poultry in Hong Kong, when infection was confirmed in 18 people, of whom 6 died (14, 54). Since 2003, more than 400 humans have been infected with HPAI H5N1 virus, with a case-fatality rate of ~60% (63).
Zoonotic transmission of both LPAI and HPAI H7 viruses has been reported relatively frequently over the past decades. These reports include zoonotic transmission in the United States in 1980 and 2004; in the United Kingdom in 1995, 2006, and 2007; and in Canada in 2004 (7, 20, 33, 34, 43, 45, 55, 61). In 2003, there was an outbreak of HPAI virus of the H7N7 subtype in The Netherlands. In order to control the outbreak, which lasted approximately 3 months, 30 million chickens were culled. Eighty-six people in close contact with infected poultry (primarily cullers, farmers, and veterinarians) became infected with the HPAI H7N7 virus. In addition, there were three probable cases of human-to-human transmission (20, 33). Most of these 89 persons suffered from conjunctivitis and/or mild influenza-like illness, but one individual developed severe pneumonia leading to acute respiratory distress syndrome and ultimately death. Sequence analysis revealed that viruses causing conjunctivitis, such as A/Netherlands/33/03 (from a conjunctivitis case [CC]), were almost identical to the prototype chicken virus, A/chicken/Netherlands/1/03. The virus isolated from the fatal case (FC), A/Netherlands/219/03, harbored 15 amino acid substitutions in basic polymerase 2 (PB2), acidic polymerase (PA), HA, neuraminidase (NA), and nonstructural protein 1 (NS1) compared to the CC virus (20) (Table (Table1).1). Most of the gene segments of the FC virus that harbor amino acid substitutions contribute to pathogenicity in other influenza viruses (reviewed in reference 15). PB2, one of the polymerase complex proteins, plays an important role in pathogenicity of HPAI viruses in mice, efficient replication of avian viruses in mammalian cells, and transmission of viruses in a guinea pig model (21, 24, 25, 37, 38, 42, 46, 48, 51-53). HA is the viral surface glycoprotein responsible for attachment and fusion; avian influenza viruses preferentially bind to α2,3-linked sialic acids (SA), whereas the HA of human influenza viruses preferentially binds to α2,6-linked SA (12). Together with the predominant distribution of α2,6-linked SA in the upper respiratory tract of humans, this indicates that the replication of avian influenza A viruses in humans is restricted at the level of virus attachment (50, 57). NA is involved in facilitating the release of virus particles from the virus-infected cell surface by cleaving SA from the cell membrane and virus particles. To obtain a proper balance between virus entry and release from the host cells, the HA-mediated receptor binding and fusion activity should be functionally compatible with the cleavage specificity and activity of NA of the virus (39). Finally, the NS1 protein contributes to pathogenicity by playing a key role in interference with the innate immune response of the host (8, 10, 22, 29, 48, 49, 56).
TABLE 1.
Amino acid differences in HPAI H7N7 viruses isolated in the Netherlands in 2003
Virus | Amino acid at the indicated position ina: | ||||||||||||||
---|---|---|---|---|---|---|---|---|---|---|---|---|---|---|---|
PB2 | PA 666 | HA | NA | NS | |||||||||||
79 | 297 | 355 | 563 | 627 | 13 | 143 | 416 | 308 | 346 | 442 | 458 | 126 | 137 | ||
A/Ck/NL/1/03 | S | V | R | Q | E | F | I | A | K | N | A | T | P | K | V |
A/NL/33/03 (CC) | S | V | R | Q | E | F | I | A | K | N | A | T | P | R | V |
A/NL/219/03 (FC) | I | I | K | R | K | L | S | T | R | S | V | A | S | K | I |
A/Ck/NL/03010132/03 | I | I | K | R | E | L | S | T | K | S | V | A | S | K | I |
Previously, we have shown that the E627K substitution in PB2 of the FC virus was the main determinant of virus pathogenicity and tissue distribution in a mouse model (42). Although data generated in a mouse model may be very useful, direct extrapolation to humans is not always possible. Using recombinant viruses with reassorted genomes and viruses with single amino acid replacements, we investigated the molecular basis of the differences in virus replication kinetics and specific steps of the virus replication cycle in in vitro model systems. Our data show that the determinants of efficient virus replication were distributed among different gene segments, indicating that H7N7 influenza A virus pathogenicity is a polygenic trait. Moreover, we show that substitutions that enhance replication in human cells were already present in a virus isolated from poultry.
MATERIALS AND METHODS
Cells.
Madin-Darby canine kidney (MDCK) cells were cultured in Eagle minimal essential medium (EMEM) (Cambrex, Heerhugowaard, The Netherlands) supplemented with 10% fetal calf serum (FCS), 100 IU/ml penicillin, 100 μg/ml streptomycin, 2 mM glutamine, 1.5 mg/ml sodium bicarbonate, 10 mM HEPES, and nonessential amino acids. 293T cells were cultured in Dulbecco modified Eagle medium (DMEM) (Cambrex) supplemented with 10% FCS, 100 IU/ml penicillin, 100 μg/ml streptomycin, 2 mM glutamine, 1 mM sodium pyruvate, and nonessential amino acids. QT6 cells, a fibrosarcoma cell line obtained from Japanese quail (40), were cultured in medium 199 (Cambrex) supplemented with 5% FCS, 1% chicken serum, 5% tryptose phosphate broth, 100 IU/ml penicillin, 100 μg/ml streptomycin, 2 mM glutamine, 0.75 mg/ml sodium bicarbonate, and nonessential amino acids. A549 cells were cultured in Ham F-12 medium supplemented with 10% FCS (HyClone), 100 IU/ml penicillin, 100 μg/ml streptomycin, and 2 mM glutamine.
Viruses.
H7N7 influenza viruses A/Netherlands/33/03 (CC) and A/Netherlands/219/03 (FC) were isolated from patients during the H7N7 outbreak in The Netherlands in 2003 (20) and passaged twice in embryonated chicken eggs. cDNA of influenza virus A/Chicken/Netherlands/03010132/03 was provided by G. Koch (Central Veterinary Institute at Wageningen UR, The Netherlands). Recombinant H7N7 influenza viruses were generated by reverse genetics as described previously (17). To this end, transient calcium phosphate-mediated transfections of 293T cells were performed as described previously (17) with plasmids carrying gene segments of the CC and FC viruses. The supernatant of the transfected cells was harvested at 48 h after transfection and was used to inoculate embryonated chicken eggs and MDCK cells. Wild-type recombinant FC and CC virus, all 60 possible reassortants thereof, and mutant viruses were produced. For efficient rescue of all recombinant and mutant viruses, 5 μg of a pCAGGS plasmid expressing PB2 of the CC or FC virus was added to the transfection mixture of 293T cells, and Vibrio cholerae neuraminidase (VCNA) (Sigma Aldrich, Zwijndrecht, The Netherlands) was added to the supernatants of 293T and MDCK cells at a concentration of 1 mU/ml. Reassortant and mutant viruses consisting of seven gene segments of influenza virus A/PR/8/34 and one gene segment of the CC or FC virus were produced using a previously described reverse genetics system for influenza virus A/PR/8/34 (17). The genotypes of recombinant viruses were confirmed by sequencing prior to use.
Plasmids.
The gene segments of the CC and FC viruses were amplified by reverse transcription-PCR (RT-PCR) and cloned in the BsmBI site of a modified version of plasmid pHW2000 (17, 27, 42). Gene segments 1, 2, 3, and 5 of the CC and FC viruses were also cloned in the KpnI and NheI sites of the eukaryotic expression plasmid pCAGGS. Plasmid pCAGGS was kindly provided by A. Garcia-Sastre (Mount Sinai School of Medicine, New York, NY).
For the construction of plasmids containing single, double, or triple nucleotide substitutions in PB2, HA, and NA, a QuikChange Multi site-directed mutagenesis kit (Qiagen, Venlo, The Netherlands) was used according to the instructions of the manufacturer.
For construction of a viral RNA-like reporter plasmid, a firefly luciferase open reading frame was PCR amplified using primers extended with the noncoding regions (NCRs) of segment 8 of the CC virus. This insert was cloned in negative sense orientation under the control of a bacteriophage T7 RNA polymerase promoter (18).
All plasmids were sequenced using a Big Dye Terminator v3.1 cycle sequencing kit (Applied Biosystems, Nieuwerkerk a/d Ijssel, The Netherlands) and a 3130XL Genetic Analyser (Applied Biosystems), according to the instructions of the manufacturer.
Replication kinetics.
Multistep replication kinetics were generated by inoculating MDCK, QT6, A549, or 293T cells at a multiplicity of infection (MOI) of 0.01 50% tissue culture infective dose (TCID50) per cell. One hour after inoculation, at time zero, cells were washed once with phosphate-buffered saline (PBS) and fresh infection medium was placed on the cells. Supernatants were sampled at 6, 12, 24, and 48 h after inoculation, and virus titers in these supernatants were determined as described below.
Virus titrations.
Virus titrations were performed by end point titration in MDCK cells as described previously (17). MDCK cells were inoculated with 10-fold serial dilutions of culture supernatants. One hour after inoculation, cells were washed once with PBS and grown in 200 μl of infection medium, consisting of EMEM (Cambrex, Heerhugowaard, the Netherlands) supplemented with 4% bovine serum albumin (BSA), 100 U/ml penicillin, 100 μg/ml streptomycin, 2 mM glutamine, 1.5 mg/ml sodium bicarbonate (Cambrex), 10 mM HEPES (Cambrex), nonessential amino acids (MP Biomedicals), and 20 μg/ml trypsin (Cambrex). Three days after inoculation, the supernatants of infected cell cultures were tested for agglutinating activity using turkey erythrocytes as an indicator of infection of the cells. Infectious titers were calculated from five replicates by the Spearman-Karber method (30).
Virus histochemistry.
Virus histochemistry using the CC-HA 143T and FC-HA 143A viruses was performed as described previously (13, 57). After concentration and purification of virus stocks using sucrose gradients, viruses were inactivated by dialysis against 0.1% formalin and labeled with an equal volume of 0.1 mg/ml of fluorescein isothiocyanate (FITC) (Sigma-Aldrich, Zwijndrecht, The Netherlands).
Formalin-fixed, paraffin-embedded tissues were deparaffinized with xylene and rehydrated with alcohol. FITC-labeled influenza viruses (50 to 100 hemagglutination units) were incubated with the tissues overnight at 4°C. The FITC label was detected with peroxidase-labeled rabbit anti-FITC (Dako, Heverlee, Belgium). Tissues were counterstained with hematoxylin and embedded in glycerol-gelatin (Merck, Amsterdam, The Netherlands). All tissues were from individuals without histological lesions or evidence of respiratory tract infection at the time of death. Tissues from three individuals were analyzed.
Minigenome assay.
Minigenome assays were performed by transfecting 293T or QT6 cells with 5 μg of pCAGGS expression plasmids encoding PB2, PB1, PA, and NP; 5 μg of the firefly luciferase reporter; 10 μg of pAR3132 expressing T7 RNA polymerase (19); and 0.2 μg of a Renilla luciferase expression plasmid (pRL; Promega, Leiden, The Netherlands) as an internal control. At 24 h after transfection, luminescence was assayed using a Dual-Glo luciferase assay system (Promega) according to the instructions of the manufacturer. Luminescence was measured in a Lumistar Galaxy apparatus (BMG Labtech GmbH, Offenburg, Germany). Relative light units (RLU) were calculated as the ratio of firefly and Renilla luciferase luminescences. The relative luminescence was calculated as a percentage of the maximum RLU in each experiment. Plasmid pEGFP-N1 (Clontech, BD Biosciences, Amsterdam, The Netherlands) was transfected in parallel, and the percentage of fluorescent cells was measured in a FACSCalibur (Becton Dickinson) flow cytometer, confirming that the transfection efficiency ranged from 95 to 100% for 293T and QT6 cells in all experiments.
Real-time RT-PCR.
RNA was isolated using a Magnapure LC system with the Magnapure LC total nucleic acid isolation kit (Roche Diagnostics, Almere, The Netherlands), and influenza A virus was detected using a real time RT-PCR assay targeting the matrix gene as described previously (41).
EM.
Transfected 293T cells were fixed for transmission electron microscopy (EM) in 4% formaldehyde and 1% glutar(di)aldehyde and postfixed in 1% osmium tetroxide. After embedding in epoxy resin, thin sections were prepared, stained with 6% saturated uranyl acetate and lead citrate, and examined with a Philips Morgagni 268D electron microscope.
Nucleotide sequence accession numbers.
Sequence data are available from GenBank under accession numbers EF015551 to EF015558.
RESULTS
Different replication kinetics of CC and FC viruses, independent of cell type.
The replication kinetics of the CC and FC viruses were studied in cells of different host species: quail QT6 cells, canine MDCK cells, and human A549 cells. In all three cell lines, the FC virus replicated to higher titers than the CC virus (Fig. (Fig.1).1). This difference was most pronounced in mammalian cells. In A549 cells, the CC virus replicated poorly. In QT6 cells, the differences in maximum virus titers after 48 h were modest, with 25-fold-higher titers for the FC virus than for the CC virus. Thus, although some differences in the susceptibilities of the three cell lines to the two influenza A viruses were observed, the faster replication kinetics of the FC virus compared to the CC virus were consistent among different cell lines. Since five gene segments of the FC virus contained substitutions compared to those of the CC virus, we investigated the role of these substitutions in different steps of the replication cycle: attachment and entry, transcription and genome replication, and virus production and release as determined by HA, the polymerase proteins, NS1, and NA.
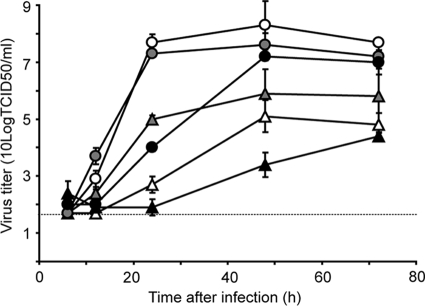
Replication kinetics of the CC (triangles) and FC (circles) viruses in MDCK (white symbols), QT6 (gray symbols), and A549 (black symbols) cells. Supernatants of cells inoculated at an MOI of 0.01 TCID50/cell were harvested at 6, 12, 24, and 48 h after inoculation and titrated by end point dilution in MDCK cells. Geometric mean titers were calculated from two independent experiments; the cutoff value was used for negative samples. Error bars indicate standard deviations. The cutoff value of the virus titration assay is indicated by a dotted line.
A single substitution in HA affects replication and attachment.
There were three amino acid differences between the HAs of the CC and FC viruses: I13S, A143T, and K416R (Table (Table1).1). The contribution of HA of the FC virus to more efficient virus replication than for HA of the CC virus was studied by comparing the FC virus and FC-CC HA virus replication kinetics. The FC-CC HA virus replicated less efficiently than the FC virus (Fig. (Fig.2),2), indicating a contribution of the amino acid substitutions in FC HA to the efficient virus replication of the FC virus compared to the CC virus. This finding was confirmed using the CC virus genome as the backbone (data not shown). To determine which of the three substitutions in HA of the FC virus was responsible for enhanced replication, three mutant HA genes were generated, consisting of the CC HA gene with either the I13S, A143T, or K416R substitution. We produced FC viruses with each of these three HAs (FC-CC HA I13S, FC-CC HA A143T, and FC-CC HA K416R) and studied the replication kinetics of these mutant viruses. As shown in Fig. Fig.2,2, the A143T substitution was solely responsible for the enhanced replication kinetics observed with HA of the FC virus. This observation was confirmed using recombinant A/PR/8/34 viruses with CC HA I13S, CC HA A143T, and CC HA K416R (data not shown). Thus, although differences in replication kinetics were small, the FC HA gene, and in particular the A143T mutation in HA, increased virus replication, irrespective of the genetic backbone of the virus.
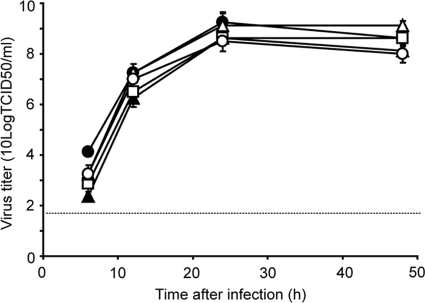
Replication kinetics of viruses with the H7 HAs in MDCK cells. MDCK cells were inoculated at an MOI of 0.01 TCID50/cell with the FC (•), FC-CC HA (), FC-CC HA I13S (□), FC-CC HA A143T (
), and FC-CC HA K416R (○) viruses. Supernatants were harvested at 6, 12, 24, and 48 h after inoculation and titrated by end point dilution in MDCK cells. Geometric mean titers were calculated from two independent experiments; the cutoff value was used for negative samples. Error bars indicate standard deviations. The cutoff value of the virus titration assay is indicated by a dotted line.
The A143T substitution introduces a potential glycosylation site at position 141, near the receptor binding site, that may affect the receptor binding specificity or affinity of HA. To determine whether the A143T substitution altered receptor binding of HA, two viruses with mutant HAs were generated: the CC virus with a T at position 143 of HA (CC-HA143T) and the FC virus with an A at position 143 of HA (FC-HA143A). The patterns of attachment of these viruses to human lung tissues were studied and compared to the attachment patterns of the CC and FC viruses using virus histochemistry methods described previously (13, 42, 57). As shown in Fig. Fig.3,3, the attachment pattern of the FC-HA143A virus was comparable to that of the CC virus, and the attachment pattern of the CC-HA143T virus was similar to that described previously for the FC virus (42). In the bronchioles, the CC-HA143T virus attached more abundantly to nonciliated cuboidal epithelial cells than the FC-HA143A virus. In the alveoli, both the FC-HA143A and CC-HA143T viruses attached to type II pneumocytes, but only the CC-HA143T virus attached to alveolar macrophages. The FC-HA143A virus also attached to type I pneumocytes, whereas the CC-HA143T virus hardly did (Fig. (Fig.3).3). Thus, the A143T substitution in HA of the FC virus caused a subtle change in virus attachment pattern to human lung tissue and increased the replication efficiency of this virus in MDCK cells.
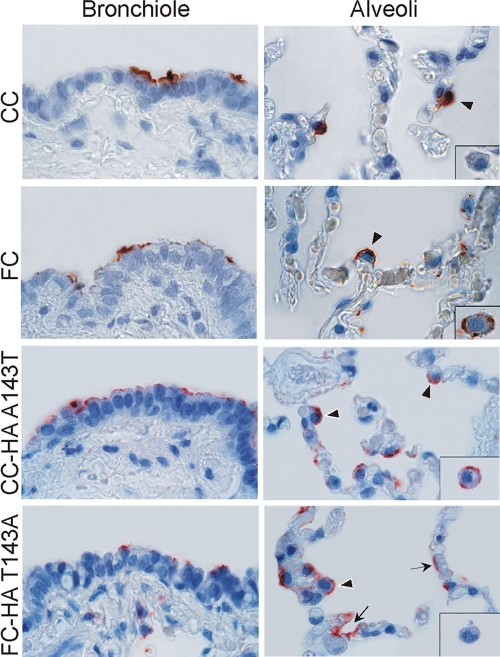
Patterns of attachment of the CC-HA143T and FC-HA143A viruses to tissues of the human lower respiratory tract. Attachment to bronchioles (left panels) and alveoli (right panels) is shown. For comparison, attachment of the CC and FC viruses to these tissues is displayed, as shown before (42). Virus attachment is shown in red. The insets in the right panels show the pattern of virus attachment to alveolar macrophages. The depicted panels reflect the attachment pattern in the whole tissue section as much as possible, but small differences between the single panels and the overall view may exist. Arrows indicate attachment to type I pneumocytes; arrowheads indicate attachment to type II pneumocytes. (Reprinted from reference 42 with permission of the publisher.)
Substitutions in PB2 and PA affect polymerase activity in human and avian cells.
To compare the polymerase activities of the CC and FC viruses, we used a T7 RNA polymerase promoter-based minigenome assay to study the effect of amino acid substitutions on polymerase activity in cells of mammalian and avian origin (18). The reporter minigenome consisted of the firefly luciferase open reading frame flanked by the NCRs of gene segment 8 of the CC virus. Furthermore, we used a plasmid constitutively expressing Renilla luciferase as an internal control to standardize transfection efficiency and overall protein expression levels.
In 293T cells, the polymerase complex of the FC virus induced higher levels of expression of the luciferase reporter than the CC virus (Fig. (Fig.4A).4A). A CC virus polymerase complex with PB2 of the FC virus yielded higher luciferase reporter levels than the CC virus polymerase complex (Fig. (Fig.4A).4A). Conversely, a polymerase complex of the FC virus with PB2 of the CC virus yielded lower luciferase expression than the wild-type FC virus polymerase complex, demonstrating that PB2 was largely responsible for the differences in polymerase activity between the CC and FC viruses. One of the five amino acid differences between the PB2s of the CC and FC viruses, glutamic acid to lysine at position 627 (E627K), was shown to be the main determinant of pathogenicity of the FC virus in a mouse model (42) and was described in in vitro studies as a determinant of host range (53) and to enable transcription at a low temperature (38). Therefore, two mutant PB2 genes were generated: CC PB2 with a lysine at position 627 (CC E627K) and FC PB2 with a glutamic acid at position 627 (FC K627E). The expression levels of the luciferase minigenome reporter induced by a polymerase complex with CC E627K were comparable to those induced by the wild-type FC PB2 protein, regardless of the origin of the rest of the polymerase complex (Fig. (Fig.4A).4A). When the polymerase complexes contained the FC K627E, the reverse pattern was observed and the expression levels of the luciferase minigenome reporter were comparable to those induced by the wild-type CC PB2 protein (Fig. (Fig.4A).4A). Thus, a lysine residue at position 627 of PB2 had a large positive effect on the polymerase activity in 293T cells.
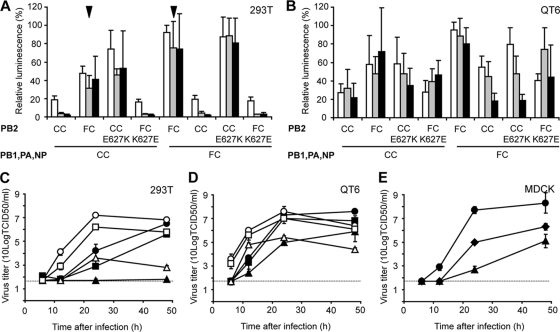
Polymerase activities and replication kinetics of H7N7 viruses. (A and B) Minigenome assays were performed by transfecting 293T (A) or QT6 (B) cells with plasmids encoding the polymerase proteins and NP of the CC and FC viruses or mutants thereof. After transfection, cells were incubated 24 h at 33°C (white bars), 37°C (gray bars), or 41°C (black bars). Luminescence of a firefly luciferase reporter was standardized using a plasmid constitutively expressing Renilla luciferase protein. Relative luminescence was calculated as the percentage relative light units (firefly luciferase/Renilla luciferase) of the maximum in each experiment. Averages and standard deviations from three independent experiments are shown. Arrowheads indicate the effect of PA on polymerase activity in the context of the FC virus PB2, as explained in the text. (C to E) Replication kinetics were determined by infecting 293T (C), QT6 (D), or MDCK (E) cells at an MOI of 0.01 TCID50/cell with the CC (triangles), FC (circles), and CC-FC PB2 (squares in panels C and D) or CC-FC PA (diamonds in panel E) virus. Supernatants were collected at 6, 12, 24, and 48 h after inoculation and titrated by end point dilution in MDCK cells. Filled symbols, 37°C; open symbols, 33°C. Geometric mean titers were calculated from two independent experiments; the cutoff value was used for negative samples. Error bars indicate standard deviations. The cutoff value of the virus titration assay is indicated by a dotted line. The replication kinetics shown for the CC and FC viruses in QT6 and MDCK cells are identical to those shown in Fig. Fig.11.
Because the effect of PB2 E627K has previously been suggested to be dependent on temperature, the minigenome assay was performed at two additional temperatures: 33°C, representing the temperature in the upper respiratory tract of humans, and 41°C, the temperature of the intestinal tract of birds. At 33°C, the overall expression levels of the luciferase minigenome reporter were higher than those at 37°C, irrespective of the polymerase complex used. At 41°C, expression levels of the luciferase minigenome reporter were comparable to those at 37°C (Fig. (Fig.4A).4A). Regardless of the temperature at which the assay was performed, the luciferase minigenome reporter expression induced by a polymerase complex with a lysine at position 627 of PB2 was higher than that induced by a polymerase complex with a glutamic acid at this position.
In QT6 cells, differences in expression of the luciferase reporter by the CC or FC virus polymerase complex were relatively small compared to those in 293T cells. The expression level of the reporter minigenome was slightly but significantly higher with the FC virus polymerase complex than with the CC virus polymerase complex, again due to PB2 of the FC virus. However, there were no significant differences in reporter expression levels with PB2 with either a glutamic acid or lysine at position 627 (Fig. (Fig.4B).4B). Also, there was no clear effect of temperature on expression levels of the minigenome reporter (Fig. (Fig.4B4B).
To confirm these results with the minigenome assays, in particular the unexpected lack of an effect of temperature on the polymerase activity, replication kinetics of the CC, FC, and CC-FC PB2 viruses were determined in 293T and QT6 cells at 33°C and 37°C. In 293T and QT6 cells, all three viruses replicated faster at 33°C than at 37°C (Fig. 4C and D). In all cases, the FC virus replicated faster than the CC virus and the CC-FC PB2 virus displayed kinetics approaching those of the FC virus. As observed in the minigenome assay, differences in replication efficiency between the three viruses and between the two temperatures were much smaller in QT6 cells than in 293T cells.
Although exchanging PA alone had no effect on the levels of luciferase reporter expressed by the polymerase complexes of the CC and FC viruses (data not shown), there was a difference in the expression of the luciferase reporter with FC PB2 in the context of the FC versus the CC polymerase complex (Fig. (Fig.4A,4A, arrowheads). At the amino acid level, the only difference between these polymerase complexes was in PA. PA F666L increased reporter expression levels in the context of the FC virus polymerase complex but not in the context of the CC virus polymerase complex. In theory, silent nucleotide changes in PB1 (n = 2), PA (n = 4), or NP (n = 1) could also explain the difference in the activities of the CC and FC polymerase complexes in the presence of FC PB2, but replication kinetics of a reassortant CC virus with PA of the FC virus showed an increased replication efficiency compared to the CC virus in MDCK cells (Fig. (Fig.4E),4E), thus excluding the possibility that silent changes in PB1 or NP contributed to the increased polymerase activity seen in the background of the FC polymerase complex.
There are no differences in modulation of the innate immune response by NS1.
There were two amino acid differences (R126K and V137I) in NS1 of the FC virus compared to the CC virus (Table (Table1).1). To test whether these substitutions in NS1 contributed to the efficient replication of the FC virus, two reassortant viruses consisting of seven gene segments of influenza virus A/PR/8/34 and NS of the CC or FC virus (PR8-CC NS and PR8-FC NS) were produced. Of note, there are no differences in NS2 of the CC virus and the FC virus at the nucleotide and protein levels. These two viruses displayed identical growth kinetics in MDCK cells, indicating that the substitutions in NS1 did not have an effect on virus replication (data not shown). To test whether the two NS1 proteins differed in their ability to counteract the induction of type I interferon (IFN) by the host, we used a firefly luciferase reporter construct under the control of an interferon-stimulated response element (ISRE) that is induced in the presence of type I IFN. Supernatants collected 24 h after infection of 293T cells with PR8-CC NS or PR8-FC NS were UV inactivated and subsequently transferred to cells transfected with the ISRE-luciferase reporter (pISRE-Luc; Clontech) and a constitutively expressed Renilla luciferase. No differences in reporter expression were observed between the virus with CC NS or FC NS; both viruses completely blocked the induction of type I IFN (data not shown).
Four substitutions independently affect NA function.
For this study, all 60 possible reassortant viruses consisting of all possible combinations of the gene segments of the CC and FC viruses were produced. By doing so, we found that all viruses containing PB2 or NA of the FC virus, or both, could be rescued without problems, whereas viruses containing PB2 and NA of the CC virus could not be, irrespective of the origin of the other six gene segments. Upon overexpression of PB2 through the addition of an extra plasmid expressing PB2 of the CC virus to the transfection mixture and trans-complementation of NA through the addition of Vibrio cholerae neuraminidase (VCNA) to the culture medium, viruses with PB2 and NA of the CC virus could also be rescued (data not shown). From these data it was concluded that besides PB2, NA also had a large impact on virus production from human 293T cells in vitro. To clarify the role of NA in the replication kinetics of the CC and FC viruses, we constructed a CC virus with NA of the FC virus and vice versa (CC-FC NA and FC-CC NA). The replication kinetics of these reassortant viruses and the wild-type viruses were determined in MDCK cells. The origin of NA had a large effect on the replication efficiency of these H7N7 viruses (Fig. (Fig.5A).5A). The CC-FC NA virus replicated faster and to higher titers than the CC virus. Conversely, the FC-CC NA virus replicated slower and to lower titers than the FC virus. The addition of VCNA to culture medium resulted in a modest increase in replication of the CC and FC-CC NA viruses, but replication was not restored to levels observed for the syngeneic viruses with the FC NA (Fig. (Fig.5B).5B). The increased replication efficiency of the CC and FC-CC NA viruses upon addition of exogenous NA indicated that the neuraminidase activity of NA of the CC virus was low. Low NA activity may result in aggregation of virus particles and hence in a relatively low infectious virus titer despite high genome copy numbers. Therefore, the number of virus genome copies present in the samples used for Fig. 5A and B were determined by quantitative RT-PCR and compared to the infectious titers of the same samples. In CC and FC virus samples with a comparable number of genome copies (depicted as cycle threshold [CT] values in Fig. Fig.5C),5C), infectious virus titers were more than 10-fold lower in CC virus samples than in FC virus samples. When VCNA was added to the culture medium, the infectious virus titer in CC virus samples was higher than that without VCNA for the same CT value. VCNA had no discernible effect on virus titers for the FC virus, suggesting that its NA gene was fully functional.

Effect of NA on replication of H7N7 virus. Supernatants of MDCK cells inoculated with an MOI of 0.01 TCID50/cell of the CC (triangles), FC (circles), CC-FC NA (squares), and FC-CC NA (diamonds) viruses in the absence (open symbols) (A) or presence (closed symbols) (B) of 1 mU/ml VCNA were harvested at 6, 12, 24, and 48 h after inoculation and titrated by end point dilution in MDCK cells. Cycle threshold values of the samples taken at 6, 12, and 24 h were determined using a real-time RT-PCR (C) and plotted against the virus titers shown in panels A and B. Geometric mean titers were calculated from two independent experiments; the cutoff value was used for negative samples. Error bars indicate standard deviations. The cutoff value of the virus titration assay is indicated by a dotted line.
To confirm the aggregation of virus particles with NA of the CC virus, virus budding was analyzed by electron microscopy (EM). To minimize intrinsic differences in virus formation between the CC and FC viruses, the FC virus was compared to an FC-CC NA reassortant. 293T cells transfected with eight plasmids carrying the FC-CC NA or FC virus genome were harvested at 12 h after transfection, when virus particles were budding from these cells. Aggregation of CC virus particles on the cell surface was clearly observed by EM, whereas FC virus particles were dispersed around the cell (Fig. (Fig.6).6). The addition of VCNA to culture medium after transfection resulted in normal release of FC-CC NA virus from 293T cells (data not shown).

Electron microscopy of H7N7 viruses budding from 293T cells. 293T cells were transfected with plasmids encoding the FC-CC NA or FC virus. At 12 h after transfection, cells were fixed and virus budding was assessed using electron microscopy.
To determine which of the four amino acid substitutions in the FC virus NA caused the efficient release of virus particles from the host cell, we produced four mutant NA genes consisting of the CC NA with one of the four substitutions and tried to rescue these in the context of the CC virus. We were unable to rescue the virus containing the T442A substitution (M3) in three independent attempts (Table (Table2).2). The other three mutant viruses could be rescued, but all replicated to lower titers than the wild-type CC virus. Therefore, all possible double and triple mutant NAs were constructed. Two double mutant viruses containing the T442A substitution in combination with N308S (M6) and A346V (M8) could not be rescued in three independent attempts. Only three of the double and triple mutant viruses (M7, M10, and M13) were rescued with similar efficiency as the CC virus, but none replicated to similar titers as the CC-FC NA or FC virus. From the single and triple mutant viruses, it was concluded that each of the four individual substitutions resulted in decreased NA function of both the CC and the FC NAs. Therefore, all four amino acid substitutions appear to be required independently for the efficient functioning of the N7 NA.
TABLE 2.
Effect of amino acid substitutions in NA on rescue of the CC virusa
Virus | Amino acid at positionb: | Rescue successc | Virus titerd (10 log TCID50/ml) | |||
---|---|---|---|---|---|---|
308 | 346 | 442 | 458 | |||
CC | N | A | T | P | +++ | 2.7-5.9 |
Single mutants | ||||||
![]() ![]() ![]() ![]() | S | A | T | P | +++ | 1.7-3.3 |
![]() ![]() ![]() ![]() | N | V | T | P | ++ | 1.9-4.3 |
![]() ![]() ![]() ![]() | N | A | A | P | − | |
![]() ![]() ![]() ![]() | N | A | T | S | +++ | 0.7-3.7 |
Double mutants | ||||||
![]() ![]() ![]() ![]() | S | V | T | P | + | 0.9 |
![]() ![]() ![]() ![]() | S | A | A | P | − | |
![]() ![]() ![]() ![]() | S | A | T | S | +++ | 3.7-5.3 |
![]() ![]() ![]() ![]() | N | V | A | P | − | |
![]() ![]() ![]() ![]() | N | V | T | S | ++ | 3.3-6.1 |
![]() ![]() ![]() ![]() | N | A | A | S | +++ | 2.5-5.9 |
Triple mutants | ||||||
![]() ![]() ![]() ![]() | S | A | A | S | + | 2.1 |
![]() ![]() ![]() ![]() | N | V | A | S | ++ | 1.1-6.1 |
![]() ![]() ![]() ![]() | S | V | T | S | +++ | 3.5-4.1 |
![]() ![]() ![]() ![]() | S | V | A | P | + | 0.7 |
CC-FC NA | S | V | A | S | +++ | 4.5-7.3 |
FC | S | V | A | S | +++ | 7.5-7.9 |
Most virulence-associated mutations were present in viruses of poultry.
The veterinarian who died of infection with the FC virus had visited only a single affected poultry farm during the H7N7 outbreak in The Netherlands. Recently, we obtained cDNA from A/Chicken/Netherlands/03010132/03 (H7N7), which circulated within the poultry of the farm visited by the veterinarian. This provided the opportunity to determine whether the amino acid substitutions in the FC virus evolved after or prior to the zoonosis. Sequence analysis of A/Chicken/Netherlands/03010132/03 revealed that 12 out of 14 amino acid substitutions in the FC virus were already present in this chicken isolate. Only the E627K substitution in PB2 and the K416R substitution in HA were not present in the chicken isolate and thus potentially resulted from virus adaptation to the new host (Table (Table1).1). Sequence analyses revealed that a virus isolated from the veterinarian 9 days after the onset of symptoms and a postmortem isolate collected 14 days after the onset of symptoms were identical, indicating that the PB2 E627K and HA K416R substitutions occurred within the first 9 days after onset of symptoms. Thus, with the exception of PB2 E627K, all substitutions that resulted in improved replication in mammalian cells were generated in poultry.
DISCUSSION
When avian influenza viruses are transmitted to humans, virus replication is often very inefficient, resulting in an asymptomatic or subclinical course of infection (3). However, avian influenza viruses may on occasion cause severe disease, and even death, in humans. In recent years, numerous studies have identified genetic markers in several influenza virus genomes, most notably Spanish influenza (H1N1) and HPAI H5N1 viruses, that were responsible for increased virulence or pathogenicity, using a variety of animal model systems. As in the Dutch H7N7 viruses, the PB2, HA, and NA genes were frequently associated with changes in virulence (reviewed in references 2, 15, and 16). Although some of these mutations determine pathogenicity indirectly, e.g., through modulation of host immune responses (8, 10, 22, 29, 48, 49, 56), most genetic markers have been linked directly to more efficient virus replication, potentially due to virus adaptation to cells of the new host. Efficient virus replication per se thus appears to be an important determinant of influenza virus pathogenicity, as was, for instance, demonstrated recently for influenza A virus laboratory strains in mice (23, 47). In the laboratory, influenza virus pathogenicity is generally characterized using animal models, including chickens, mice, ferrets, and macaques. Unfortunately, animal model systems are imperfect for studies of influenza virus pathogenicity in humans, due to, for instance, differences in expression of viral receptors or other host-specific factors required for virus replication, host immune responses, or even very basic characteristics of the model system such as anatomy or body temperature. Therefore, in vitro assays to assess adaptation to replication in the mammalian host can be of great additional value.
In the present study, a number of in vitro assay systems were used to identify genetic differences between the virus genes of human H7N7 influenza viruses causing fatal and nonfatal disease that could be responsible for differences in pathogenicity. Previously, we have shown that an E627K substitution in PB2 was the main determinant of pathogenicity of the FC virus in mice and that there was, perhaps, a modest contribution of HA (42). Others have shown that the FC virus was also more pathogenic than the CC virus in ferrets (5) and upon ocular inoculation of mice (6), but the molecular basis of the differences in pathogenicity was not determined in these models. In the present study, the contribution of each of the amino acid differences between the FC and CC viruses to differences in replication efficiency was determined, as well as the underlying mechanism. Four of the five gene segments of the FC virus with amino acid substitutions were shown to be involved in its efficient replication, which could thus explain why the FC virus was more pathogenic than the CC virus in humans (5, 42). These four gene segments, HA, PB2, PA, and NA, play roles at different stages of the viral replication cycle, and these results therefore provide strong arguments that efficient virus replication, and by extrapolation also pathogenicity, of avian influenza virus in humans is a truly polygenic trait. The determinants of efficient virus replication of H7N7 viruses in mammalian cells as determined here share many features with those described previously for viruses as divergent as the H1N1 Spanish influenza virus and the HPAI H5N1 viruses that emerged from Asia since 1996.
The increased replication efficiency of a virus with HA of the FC virus was caused by a single amino acid substitution from alanine to threonine at position 143 that introduces a potential N-linked glycosylation site at position 141 of HA. This substitution was solely responsible for the difference in attachment pattern between the CC and FC viruses described earlier (42), with more abundant attachment of the FC virus to nonciliated cuboidal epithelial cells in the bronchioles and attachment to alveolar macrophages and a more abundant binding of the CC virus to type I pneumocytes in the alveoli. Hemagglutination assays using modified turkey erythrocytes containing α2,3-linked SA or α2,6-linked SA exclusively revealed that the FC virus binds efficiently to α2,3-linked SA and weakly to α2,6-linked SA, whereas the CC virus binds only to α2,3-linked SA (data not shown). Although recently published glycan array data for the FC virus did not reveal significant binding of the FC virus HA to α2,6-linked SA (4), the results of hemagglutination assays with resialylated turkey erythrocytes, the virus histochemistry experiments (Fig. (Fig.3),3), and experimental infections of mice (42) all point to a subtle change in receptor affinity, which is of importance for efficient replication in vitro and possibly also in humans and mice.
The contribution of the polymerase proteins PB2 and PA to the enhanced replication of the FC virus was demonstrated in minigenome assays and confirmed using reassortant viruses. PB2 of the FC virus caused a large increase in expression levels of the luciferase minigenome reporter in human cells and a smaller increase in avian cells. This increase was determined by the E627K substitution in PB2 of the FC virus, in agreement with previous observations for other influenza A viruses (38), and could explain the dramatic effect of E627K on virulence of HPAI H5N1 and H7N7 viruses in mice (24, 25, 37, 38, 42, 46, 48, 51, 53). The E627K substitution in PB2 has been suggested to represent an adaptation upon influenza virus zoonoses, as it was shown to result in increased polymerase activity in mammalian cells and at relatively low temperatures (38, 53). This issue, however, remains controversial, as the effect of E627K appears to be virus specific (35) and variable in different cell types (25). The polymerase complexes of both the CC and FC viruses were active at 33°C in minigenome assays, and luciferase reporter expression was higher at 33°C than at 37°C, independent of the residue at position 627 of PB2 (Fig. (Fig.4A).4A). The FC virus replicated more efficiently than the CC virus in 293T, QT6, and MDCK cells, and both viruses replicated more efficiently at 33°C than at 37°C (Fig. 4C and D and data not shown). Our data thus indicate that the E627K substitution in the HPAI H7N7 virus was merely a determinant of increased replication, not directly related to temperature optimum or host specific factors. The observation that polymerase complexes with the “human-like” lysine at position 627 still replicate efficiently in cells of avian origin (QT6) (Fig. (Fig.4B)4B) could explain why some HPAI H5N1 viruses with 627K have continued to circulate in wild birds and poultry since 2005 (9). Moreover, it was shown that the E627K substitution in the HPAI H5N1 virus A/HongKong/156/97 also had little effect on reporter expression in a minigenome assay in avian cells (35), as shown here for the HPAI H7N7 virus.
The exchange of PAs of the CC and FC viruses alone did not affect expression levels of the luciferase minigenome reporter in minigenome assays (data not shown). However, the FC virus PA gene resulted in higher reporter expression than the CC virus PA gene when PB2 was also derived from the FC virus. Such a synergism between PB2 and PA was also described for PB2 and PA of A/HongKong/156/97 (H5N1) (35). Moreover, PB2 of a human H3N2 virus with an equine PA gene resulted in a lack of reporter expression in minigenome assays (36), also indicating a relationship between PB2 and PA. The mechanism behind this synergism may lie in the interaction that was recently observed between PB2 and PA (26), but since the interaction was determined to be in the N-terminal 100 amino acids of PA, whereas the CC virus and FC virus PAs differ at amino acid position 666, this is unlikely. Another possibility could be a lack of functional polymerase complex formation (36).
Amino acid substitutions in NA of the FC virus had a dramatic effect on the replication kinetics of this virus, pointing to a major contribution of NA as a determinant of efficient replication and, by extrapolation, pathogenicity. The low neuraminidase activity of the CC virus NA resulted in aggregation of virus particles on the cell surface and hence poor release of infectious virus. All four amino acid differences between the FC and CC virus NAs affected NA function. None of the single mutations introduced in the CC virus NA, or any combination thereof, resulted in a phenotype comparable to that of the virus with FC NA. In fact, most mutations and combinations thereof resulted in decreased virus replication. Similarly, it was shown that five amino acid residues were responsible for the higher neuraminidase activity of the A/Singapore/1/57 (H2N2) NA compared to NA of A/England/12/62 (H2N2) (32). Since a proper balance of HA and NA activities is required for efficient virus replication (59), the substitutions in NA may be related to the alterations in binding specificity or affinity in HA of the FC virus. However, the effect of NA on replication is independent of HA of the FC virus, since the CC-FC NA virus, with HA of the CC virus and NA of the FC virus, replicated more efficiently than the CC virus in MDCK and QT6 cells (Fig. (Fig.55 and data not shown). Therefore, the effect appears to be caused by a structural or functional feature of NA itself. Sequence analysis of NA showed that both the CC and FC viruses contain all residues that are conserved between known viral and bacterial neuraminidases and all residues conserved in influenza A virus neuraminidases that make up the enzymatic site of NA (reviewed in reference 11). The same holds true for the residues that indicate the presence of a second sialic acid binding site in NA of avian viruses (58) and residues that changed sialidase activity (32) and substrate specificity (31) of avian NA.
It has been suggested that quail act as an intermediate host in the zoonotic spread of avian influenza viruses from aquatic birds to other species (28, 44). Likewise, the extensive circulation of the H7N7 HPAI virus during the 2003 outbreak may have facilitated adaptation from replication in the original host toward replication in poultry. These replication-enhancing mutations potentially paved the way for the virus to enter the human lower respiratory tract, where it acquired the E627K substitution. The sequence information from the chicken H7N7 virus isolated from the farm visited by the deceased veterinarian implies that mutations that facilitate replication in human cells can already arise in poultry. The current widespread circulation in poultry and wild birds of HPAI H5N1 viruses in Asia, Europe, and Africa, with or without the E627K substitution, is therefore a reason for concern. The identification of mutations that enhance replication in chickens as well as in mammalian cells underlines the need for continuous virus surveillance in wild birds and poultry and assessment of the pathogenic properties of these viruses in mammalian species in animal models but certainly also in in vitro assays.
Acknowledgments
We thank Guus Koch and Sylvia Pritz-Verschuren at CVI, Lelystad, for providing us with A/Chicken/Netherlands/03010132/03; Adolfo Garcia-Sastre for plasmid pCAGGS; and John Dunn for plasmid pAR3132. We thank Ton de Jong, Frank van der Panne, Theo Bestebroer, Monique Spronken, Brigitte van Uden, and Bernadette van den Hoogen for excellent technical assistance.
This study was funded in part by EU FP6 program RiviGene (no. SSPE-CT-2005-022639) and contract NIAID-NIH HHNSN266200700010C.
REFERENCES
Articles from Journal of Virology are provided here courtesy of American Society for Microbiology (ASM)
Full text links
Read article at publisher's site: https://doi.org/10.1128/jvi.01783-09
Read article for free, from open access legal sources, via Unpaywall:
https://jvi.asm.org/content/jvi/84/3/1597.full.pdf
Free to read at jvi.asm.org
http://jvi.asm.org/cgi/content/abstract/84/3/1597
Free after 4 months at jvi.asm.org
http://jvi.asm.org/cgi/content/full/84/3/1597
Free after 4 months at jvi.asm.org
http://jvi.asm.org/cgi/reprint/84/3/1597
Citations & impact
Impact metrics
Citations of article over time
Alternative metrics
Article citations
Vaccination and Antiviral Treatment against Avian Influenza H5Nx Viruses: A Harbinger of Virus Control or Evolution.
Vaccines (Basel), 11(11):1628, 24 Oct 2023
Cited by: 1 article | PMID: 38005960 | PMCID: PMC10675773
Review Free full text in Europe PMC
Avian Influenza Virus Tropism in Humans.
Viruses, 15(4):833, 24 Mar 2023
Cited by: 6 articles | PMID: 37112812 | PMCID: PMC10142937
Review Free full text in Europe PMC
Phenotypic effects of mutations observed in the neuraminidase of human origin H5N1 influenza A viruses.
PLoS Pathog, 19(2):e1011135, 06 Feb 2023
Cited by: 7 articles | PMID: 36745654 | PMCID: PMC9934401
Key amino acid position 272 in neuraminidase determines the replication and virulence of H5N6 avian influenza virus in mammals.
iScience, 25(12):105693, 30 Nov 2022
Cited by: 1 article | PMID: 36567717 | PMCID: PMC9772848
The influenza virus PB2 protein evades antiviral innate immunity by inhibiting JAK1/STAT signalling.
Nat Commun, 13(1):6288, 21 Oct 2022
Cited by: 23 articles | PMID: 36271046 | PMCID: PMC9586965
Go to all (113) article citations
Data
Data behind the article
This data has been text mined from the article, or deposited into data resources.
BioStudies: supplemental material and supporting data
Nucleotide Sequences (2)
- (1 citation) ENA - EF015551
- (1 citation) ENA - EF015558
Similar Articles
To arrive at the top five similar articles we use a word-weighted algorithm to compare words from the Title and Abstract of each citation.
Comparative analysis of avian influenza virus diversity in poultry and humans during a highly pathogenic avian influenza A (H7N7) virus outbreak.
J Virol, 85(20):10598-10604, 17 Aug 2011
Cited by: 30 articles | PMID: 21849451 | PMCID: PMC3187520
Emergence of the virulence-associated PB2 E627K substitution in a fatal human case of highly pathogenic avian influenza virus A(H7N7) infection as determined by Illumina ultra-deep sequencing.
J Virol, 88(3):1694-1702, 20 Nov 2013
Cited by: 47 articles | PMID: 24257603 | PMCID: PMC3911586
Rapid emergence of a virulent PB2 E627K variant during adaptation of highly pathogenic avian influenza H7N7 virus to mice.
Virol J, 10:276, 05 Sep 2013
Cited by: 22 articles | PMID: 24007444 | PMCID: PMC3766704
Avian influenza viruses in humans.
Rev Sci Tech, 28(1):161-173, 01 Apr 2009
Cited by: 49 articles | PMID: 19618624
Review