Abstract
Free full text

siah-1 Protein Is Necessary for High Glucose-induced Glyceraldehyde-3-phosphate Dehydrogenase Nuclear Accumulation and Cell Death in Müller Cells*
Abstract
The translocation and accumulation of glyceraldehyde-3-phosphate dehydrogenase (GAPDH) in the nucleus has closely been associated with cell death induction. However, the mechanism of this process has not been completely understood. The E3 ubiquitin ligase siah-1 (seven in absentia homolog 1) has recently been identified as a potential shuttle protein to transport GAPDH from the cytosol to the nucleus. Previously, we have demonstrated that elevated glucose levels induce GAPDH nuclear accumulation in retinal Müller cells. Therefore, this study investigated the role of siah-1 in high glucose-induced GAPDH nuclear translocation and subsequent cell death in retinal Müller cells. High glucose significantly increased siah-1 expression within 12 h. Under hyperglycemic conditions, siah-1 formed a complex with GAPDH and was predominantly localized in the nucleus of Müller cells. siah-1 knockdown using 50 nm siah-1 small interfering RNA significantly decreased high glucose-induced GAPDH nuclear accumulation at 24 h by 43.8 ± 4.0%. Further, knockdown of siah-1 prevented high glucose-induced cell death of Müller cells potentially by inhibiting p53 phosphorylation consistent with previous observations, indicating that nuclear GAPDH induces cell death via p53 activation. Therefore, inhibition of GAPDH nuclear translocation and accumulation by targeting siah-1 promotes Müller cell survival under hyperglycemic conditions.
Introduction
Several reports have demonstrated that the nuclear translocation and accumulation of the glycolytic enzyme glyceraldehyde-3-phosphate dehydrogenase (GAPDH)2 play important roles in early events leading to cell death initiation and execution (1, 2). Consequently, GAPDH nuclear accumulation has been suggested to participate in the development of various degenerative diseases including diabetic retinopathy (1, 3,–9, 11). Induction of GAPDH nuclear translocation and accumulation has been linked to the formation of oxidative stress and production of pro-inflammatory stimuli, such as nitric oxide and cytokines (2, 11,–16).
Although some events and stimuli leading to GAPDH nuclear accumulation have been identified, the mechanism of movement of GAPDH from the cytosol to the nucleus is unclear. GAPDH lacks a common nuclear localization signal (NLS) and therefore cannot enter the nucleus by itself because of its size. A recent study has identified the E3 ubiquitin ligase siah-1 (seven in absentia homolog 1) as a potential carrier/shuttle protein (13). According to this study, GAPDH binds the NLS-bearing siah-1, forming a complex subsequently promoting translocation of GAPDH from the cytosol to the nucleus. It is postulated that the NLS on siah-1 facilitates the nuclear movement of the complex.
siah-1 proteins are human homologs of the evolutionarily conserved Drosophila, E3 ubiquitin ligase sina (seven in absentia) protein (17). sina was first identified as a key protein during R7 photoreceptor development in the Drosophila fruit fly whereby it facilitates the degradation of the transcriptional repressor of neuronal fate tramtrack (TTK88) (18,–20). Follow-up studies have identified functions for siah-1 in various cellular processes including mitosis, neuronal plasticity, development, angiogenesis, inflammation, and cell death (13, 17, 20,–29). Two siah genes, siah-1 and siah-2 are present in humans and rats, whereas mice have three siah genes: siah-1a, siah-1b, and siah-2 (17, 30, 31). siah-1a and siah-1b have 98% sequence homology. Structurally, siah-1 contains an N-terminal RING domain that facilitates the ligase function, two cysteine-rich zinc finger domains, and a substrate-binding domain, which is localized on the C-terminal of the protein (27, 32, 33). The last 12 amino acids on the C-terminal in the substrate-binding domain are necessary for GAPDH-siah-1 interaction (13). The NLS motif is also located in the substrate-binding domain.
We have recently identified that elevated glucose levels act as a stimulus for GAPDH nuclear accumulation in retinal Müller (glia) cells via high glucose-induced activation of a caspase-1/interleukin-1β signaling pathway (16). We have demonstrated that hyperglycemia-induced GAPDH nuclear accumulation not only occurs in retinal Müller cells in vitro but also in vivo during the development of diabetic retinopathy in rodents (4). Because Müller cells maintain the retinal environment (34,–39), death processes within these cells can potentially compromise their function leading to disease. A better understanding of mechanisms leading to GAPDH translocation from the cytosol to the nucleus is needed to develop more target-specific treatments. Some studies have already indicated the potential of inhibiting this event by showing increased cell survival in vivo and in vitro (4, 9, 14, 40). Therefore, the purpose of this study was to determine whether high glucose regulates siah-1 in Müller cells and to examine the potential of this protein to bind and regulate GAPDH nuclear movement and cell death under hyperglycemic conditions.
EXPERIMENTAL PROCEDURES
Materials
Goat anti-siah-1 antibody and goat anti-mouse antibody conjugated to horseradish peroxidase was from Santa Cruz Biotechnology Inc. (Santa Cruz, CA), whereas rabbit anti-goat conjugated to horseradish peroxidase was from R & D Systems (Minneapolis, MN). Mouse anti-GAPDH antibody was purchased from Chemicon International (Temecula, CA). Mouse anti-histone 2B was from MBL laboratories (Naka-ku Nagoya, Japan). Rabbit anti-lactate dehydrogenase (LDH) was from Abcam (Cambridge, MA). Rabbit anti-Phospho-p53-s15 and rabbit anti-p53 were purchased from Cell Signaling (Danvers, MA). Rabbit anti-Bax was from BD Biosciences (San Jose, CA). Rabbit anti-goat antibody conjugated to Alexa 594 and goat anti-mouse IgG conjugated to Texas Red were from Invitrogen. siah-1 targeting siRNA and control nontargeting scrambled and control risk-free siRNA oligonucleotides were from Dharmacon (Lafayette, CO). The Amaxa nucleofection kit L was purchased from Lonza (Cologne, Germany).
Methods
Tissue Culture-Rat Retinal Müller Cell Line (rMC-1)
rMC-1 have previously been characterized and established by others and us as a useful tool for retinal Müller cell studies (4, 41, 42). rMC-1 were maintained in normal (5 mm) glucose Dulbecco's modified Eagle's medium supplemented with 10% fetal bovine serum and 1% penicillin/streptomycin at 37 °C and 5% CO2 in a humidified incubator. The experiments were done with passages lower than 25.
Human Retinal Müller Cells (hMC)
Handling of human tissue conformed to the tenets of Declaration of Helsinki for research involving human tissue. hMC were isolated from the retinas of nondiabetic donors as previously described by us (4, 16). After three passages, hMC cultures were 95% pure and were characterized as described previously (4, 43). Only hMCs from passages 3–6 were used for the experiments.
Normal and High Glucose Treatment
rMC-1 were treated in experimental Dulbecco's modified Eagle's medium supplemented with 2% fetal bovine serum and 1% penicillin/streptomycin containing either normal (5 mm) glucose or high (25 mm) glucose.
hMC were treated in experimental Dulbecco's modified Eagle's medium/Ham's F-12 (1:1 ratio) medium supplemented with 2% fetal bovine serum and 1% penicillin/streptomycin containing either normal (7.8 mm) glucose or high (25 mm) glucose. For long term treatments, the media were changed every 24 h.
Cytosolic and Whole Cell Lysate
rMC-1 (1.0 × 106) and hMC (5.0 × 105) were treated as described above. Following treatment, cytosolic lysates were generated as previously described by us (4, 16, 44). Whole cell lysates were generated in 200 μl of whole cell lysis buffer (50 mm HEPES, pH 7.5, 1% Triton X-100, 150 mm NaCl, 1 mm EDTA, and protease inhibitors 0.2 mm phenylmethylsulfonyl fluoride and 1 μm leupeptin) and sonicated for 15 s. The protein concentrations were determined using the Bradford assay.
Western Blot Analysis
Lysates (20 μg) were separated on SDS gel by electrophoresis and blotted onto nitrocellulose membrane. The membranes were blocked in either filtered 5% bovine serum albumin in PBS-T (phosphate-buffered saline containing 1% Tween 20) (siah-1) or 5% milk in PBS-T (actin, GAPDH, phospho-p53-s15, bax, p53) and incubated for 24 h with the goat polyclonal antibody against either siah-1 (1:500), mouse monoclonal antibody against GAPDH (1:5000), mouse monoclonal against β-actin (1:10,000), rabbit polyclonal against phospho-p53-s15(1:1000), rabbit polyclonal against bax (1:1000), or rabbit polyclonal against total p53 (1:1000). The antibodies were diluted in respective blocking solutions. The membranes were incubated with horseradish peroxidase-conjugated secondary antibodies and developed using enhanced chemiluminescence horseradish peroxidase detection reagent (Pierce). The bands were quantified via densitometry analysis using the Bio-Rad Quantity One program and expressed as the ratio between proteins of interest to actin. Phospho-p53-s15 bands were normalized to total p53. Histone 2B (1:1000) and lactate dehydrogenase (1:1000) were used to demonstrate purity of nuclear or cytosolic fractions, respectively.
Reverse Transcription and Real Time Quantitative PCR
Total RNA from treated cells was isolated using TRIzol reagent (Invitrogen) and treated with Purelink DNase treatment (Invitrogen) to digest DNA. RNA (2 μg) was reverse transcribed using Applied Biosystem high capacity cDNA reverse transcription kit (Foster City, CA). siah-1 mRNA levels were determined through quantitative real time PCR assays using the TaqMan® gene expression assays and the Applied Biosystems PRISM 7900HT sequence detection system. 18 S RNA was used to normalize for the starting amount of cDNA, and the assays were performed in triplicate. Fold changes relative to control treatment were quantified. Context sequences provided by the manufacturer for amplified genes are follows: rat siah-1, CTTCACAGAGAATAAGGCACCCATC); rat 18s, TGGAGGGCAAGTCTGGTGCCAGCAG; human siah-1, CTCTCCGCCCACAGAAATGAGCCGT; and human 18s, TGGAGGGCAAGTCTGGTGCCAGCAG.
Immunofluorescence Analysis of siah-1 and GAPDH
rMC-1 (5.0 × 104) or hMC (3.0 × 104) plated on glass coverslips were treated with normal or high glucose for 24 (rMC-1) or 48 (hMC) h. Following treatment, the cells were fixed in a solution of freshly prepared 4% paraformaldehyde for 10 min at room temperature and rinsed twice with PBS. The cells were permeabilized with ice-cold acetone for 10 min, blocked with 1% bovine serum albumin in PBS-T and incubated overnight at 4 °C with commercially available antibodies against GAPDH (1:800 dilution). For siah-1 primary antibody (1:200 dilution), the cells were incubated at 4 °C for at least 48 h. The cells were then rinsed twice with PBS and incubated in either 5% rabbit serum (siah-1) or 5% goat serum (GAPDH) in 1% bovine serum albumin/PBS for 30 min, followed by 1 h of incubation with the appropriate secondary antibody (anti-goat secondary antibody conjugated to Alexa Fluor 594–1:1000 dilution for siah-1-hMC or anti-mouse secondary antibody conjugated to Texas Red-1:200 dilution for GAPDH-rMC-1) at room temperature. Coverslips were rinsed extensively in PBS-T and mounted on glass slides using Vectashield anti-fade fluorescence mounting medium (Vector Laboratories, Burlingame, CA). Blinded samples were examined for siah-1 and GAPDH nuclear accumulation using a fluorescent microscope (40× magnification; excitation, 540 nm; emission, 600 nm). Digital images were acquired on a Leica DMI 6000 B inverted microscope using a Retiga EXI camera (Q-imaging, Vancouver, Canada) at 40× magnification. The percentage of cells that were positive for nuclear siah-1 and GAPDH in four different fields/sample was established. The samples were then unblinded, and the average values of several individual experiments were presented.
Subcellular Fractionation
rMC-1 (2.5 × 106) were treated as described above with normal or high glucose for 24 h. Following treatment, the nuclear fractions were generated as previously described (16). Briefly, treated cells were rinsed twice with ice-cold Hanks' buffered saline solution, scraped in ice-cold homogenization buffer, and resuspended in 200 μl of homogenization buffer. Suspension was homogenized with 10 strokes of a homogenizer, and nuclear fractions were collected by a low speed spin (1000 × g) for 5 min. Nuclear fractions were washed in homogenization buffer twice and resuspended in low stringency ice-cold radio immunoprecipitation buffer (50 mm Tris, pH 7.5, 1% Triton X-100, 0.25% sodium deoxycholate, 0.1% SDS, 150 mm sodium chloride, 1 mm EDTA, 1 mm phenylmethylsulfonyl fluoride, 1 μg/ml leupeptin) for co-immunoprecipitation analysis. Cytosolic fraction-containing supernatant was retained for analysis of fraction purity. The protein concentrations were determined via Bio-Rad protein assay.
Co-immunoprecipitation Assays
Equal amounts of protein (1000 μg) were precleared using 30 μl of protein G plus agarose beads at 4 °C for 3 h. The beads were pelleted, and supernatant was transferred to a fresh microcentrifuge tube. 7.5 μg of anti-siah-1 antibody was added to the supernatant and incubated for 2 h with constant rotation. Protein G plus agarose beads (30 μl) were then added to the supernatant and incubated overnight at 4 °C with constant rotation. Antibody and protein bound beads as well as the bead only controls were pelleted by a 14,000 × g spin (1 min). Supernatants lacking siah-1 because of siah-1 protein pulldown by immunoprecipitation were retained. These siah-1-depleted supernatants were subjected to siah-1 Western blot analysis alongside a siah-1 positive control to ascertain efficient siah-1 pulldown. Immunoprecipitates were washed five times in ice-cold high stringency radio immunoprecipitation buffer (50 mm Tris, pH 7.5, 1% Triton X-100, 0.25% sodium deoxycholate, 0.1% SDS, 500 mm sodium chloride, 10 mm sodium fluoride, 1 mm EDTA, 1 mm phenylmethylsulfonyl fluoride, 1 μg/ml leupeptin,), resuspended in 20 μl sample buffer, vortexed, boiled at 100 °C for 5 min, and subjected to Western blot analysis as described above. The membrane was then stripped and reprobed with antibody against GAPDH to determine complex formation. As control, LDH was used to demonstrate that siah-1/GAPDH binding is specific.
siah-1 siRNA Transfection
To knock down siah-1, rMC-1 were transfected with siah-1-specific SMART pool siRNA, which contains a pool of four siRNA duplexes (Table 1) using Amaxa nucleofection electroporation system (Cologne, Germany). For control, the cells were subjected to electroporation only or transfected with 50 nm nonspecific scrambled siRNA or risk-free siRNA. Sequences for control siRNA are patent-protected. Transfection was performed according to the manufacturer's instructions. Briefly, rMC-1 were harvested by centrifugation and resuspended at 3.0 × 106 cells/100 μl in solution L (Amaxa kit). 100 μl of cell suspensions with or without siRNA were dispensed to electroporation cuvettes. The final concentration of siRNA was either 20 or 50 nm. Transfection efficiency by this method was 65%. Following electroporation, the cells were plated on tissue culture plates containing regular growth medium. For experiments, the cells were switched to experimental medium 12 or 24 h post-transfection and treated as described above.
TABLE 1
Rat ON-TARGET SMART pool siah-1 siRNA
The control siRNA sequences are patent-protected.
ON-TARGETplus SMART pool siRNA | Target sequence |
---|---|
J-094413-09, siah-1 | CAACAAUGACUUGGCGAGU |
J-094413-10, siah-1 | GGUCAUGGGCCACCGCUUU |
J-094413-11, siah-1 | CCGAAAAGGCAGAGCACGA |
J-094413-12, siah-1 | GAGAUAACUCUGCCGCACA |
siah-1 Truncation Mutation
siah-1 C-terminal truncation of the last 12 amino acids was generated by site-directed mutagenesis on a siah-1 cDNA, using PCR with stop codon-containing primers. Wild type siah-1 construct was also generated for control. A Myc epitope tag (EQKLISEEDL) was engineered into the N terminus of both proteins. The primer sequences used for construct generation were as follows: siah-1 wt, 5′-TGATGAATTCATGGAACAAAAACTCATCTCAGAAGAGGATCTGAGCCGTCAGACTGCTACAGCATTACC-3′ (outer forward primer); siah-1wt, 5′-GTCAGCGGCCGCTCAACACATGGAAATAGTTACATTGATGC-3′ (outer reverse primer); siah-1Δ amino acids 1–270, 5′-TGATGAATTCATGGAACAAAAACTCATCTCAGAAGAGGATCTGAGCCGTCAGACTGCTACAGCATTACC-3′ (outer forward primer); siah-1Δ amino acids 1–270, 5′-GACTGCGGCCGCCTAATTTTCTGCAAAGAGCTGTGCAATGCTG′ (outer reverse primer). The primer sequences used for sequencing confirmation were as follows: siah-1wt, ATAGCCAAGTTGCGAATG; siah-1Δ amino acids 1–270, CTCAAAGTGTCCACCATCC. The wild type (siah-1wt) and truncated siah-1 (siah-1Δ amino acids 1–270) cDNAs were then cloned downstream of the cytomegalovirus promoter-enhancer in the mammalian expression vector pcDNA3.1 (Invitrogen). Construct generation, cloning, and plasmid amplification services were provided by Seqwright Sequencing Inc. (Houston, TX).
Trypan Blue Cell Death Assay
Following treatment with 5 or 25 mm glucose for 96 h, rMC-1 (1 × 106) transfected with either control scrambled (50 nm) or siah-1(50 nm) siRNA were trypsinized (0.25% trypsin, 2 min). Equal amounts (100 μl) of cell suspension and trypan blue solutions were mixed. Blinded samples were counted using hemocytometer, and cell death was quantified as the number of blue cells/total cell number. The samples were then unblinded, and the values from independent experiments were averaged.
Caspase Activity Assay
Caspase activities were measured as described previously (4, 44,–47). Briefly, equal amounts of cytosolic lysates (15 μg) from rMC-1 cells treated for 96 h were incubated in lysate buffer containing the fluorogenic caspase substrate (2.5 μm) in a total volume of 100 μl at 32 °C for 1 h. Cleavage of the substrate emits a fluorescence signal that was quantified by Tecan Spectra FluorPlus fluorescence plate reader (excitation, 400 nm; emission, 505 nm). Caspase activities were calculated against an 7 amino-4-trifluoromethylcoumarin standard curve and expressed as pmol of 7 amino-4-trifluoromethylcoumarin/mg of protein/min.
Statistical Analysis
The data were analyzed using one-way analysis of variance (correlated samples, p < 0.05) followed by Tukey's post-analysis to determine statistical significance among groups. The ordinal data were analyzed using a Kruskal-Wallis test (p < 0.05) followed by Dunn's post analysis to determine the statistical significance among groups. For details in statistical analysis see the VasserStats statistical computation web site.
RESULTS
Effect of High Glucose on siah-1 Expression in rMC-1
We have recently shown that hyperglycemia leads to cell death of retinal Müller cells via GAPDH nuclear accumulation (4, 16). However, the mechanism of GAPDH translocation from the cytosol to the nucleus is unknown. Because a recent report has suggested that siah-1 can act as a carrier protein during this process (13), we determined whether high glucose can regulate expression of this protein. High (25 mm) glucose significantly increased siah-1 mRNA levels within 12 h by 1.9 ± 0.1-fold in rMC-1 compared with control cells cultured under normal (5 mm) glucose conditions (Fig. 1A). In addition, a significant, 1.4 ± 0.1-fold increase in siah-1 protein was detected after 12 h of treatment (Fig. 1B). Increased siah-1 protein levels were sustained throughout 24 h, the time point at which GAPDH nuclear accumulation can significantly be determined (16).
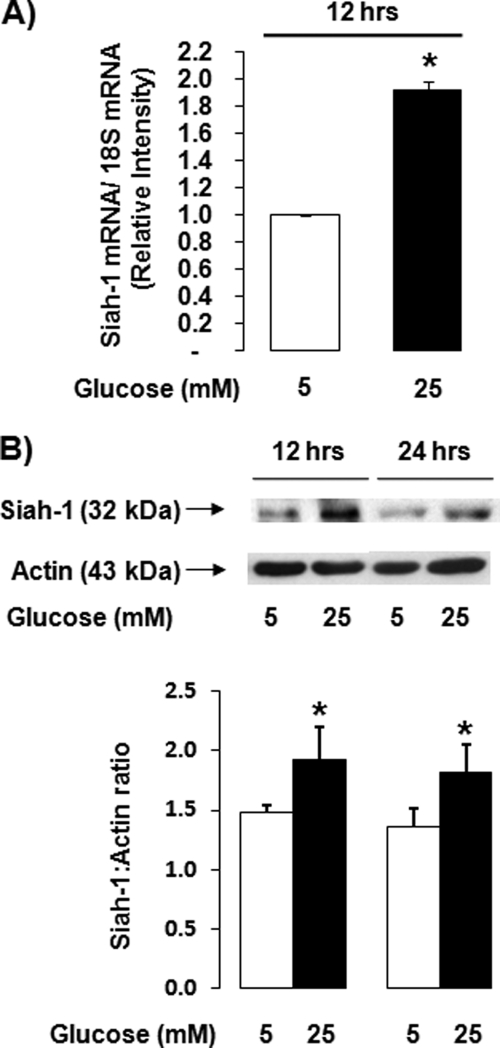
siah-1 is regulated by high glucose in transformed rat retinal Müller cells. rMC-1 were cultured in normal (5 mm) glucose or high (25 mm) glucose for up to 24 h. A, siah-1 mRNA levels were quantified using real time quantitative PCR analysis following treatment for 12 h. The values from independent experiments were normalized and graphed as means ± S.E. (n = 5; *, p < 0.05). B, changes in siah-1 protein levels were determined using Western blot analysis. siah-1 Western blots were quantified using densitometry analysis, normalized to actin, and graphed as the means ± S.E. (n = 4; *, p < 0.05).
Determination of siah-1 Protein Levels and Localization in hMCs
To confirm that high glucose-induced changes in siah-1 were not the result of transformation in the rMC-1 cell line, the effect of elevated glucose levels on isolated human Müller cells (hMC) was determined. High glucose (25 mm) treatment induced a modest but significant increase in siah-1 mRNA levels by 1.4 ± 0.03-fold compared with control (Fig. 2A) and a significant 1.7 ± 0.2-fold increase in siah-1 protein levels as determined by Western blot analysis (Fig. 2B). The antibody against human siah-1 allowed for determination of siah-1 localization within hMC under normal and high glucose conditions. A significant increase in the number of cells positive for nuclear siah-1 was observed in cells cultured under high glucose (69.7 ± 9.4%) conditions compared with control cells cultured in normal glucose (24.5 ± 4.0%) (Fig. 2C).
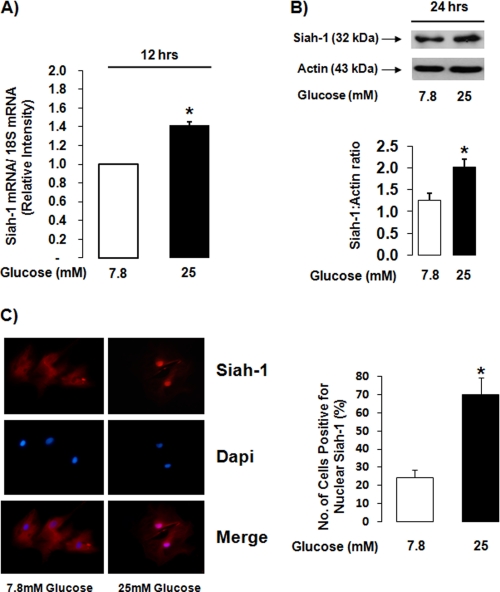
siah-1 levels are increased in high glucose-treated isolated human Müller cells and are localized in the nucleus. hMC were cultured in normal (7.8 mm) glucose or high (25 mm) glucose for up to 48 h. A, siah-1 mRNA levels were quantified using real time quantitative PCR analysis following treatment for 12 h. The values from independent experiments were normalized and graphed as the means ± S.E. (n = 3; *, p < 0.05). B, changes in siah-1 protein levels were examined using Western blot analysis following treatment for 24 h. siah-1 Western blots were quantified using densitometry analysis, normalized to actin, and graphed as the means ± S.E. (n = 4; *, p < 0.05). C, siah-1 fluorescence microscopy analysis was used to determine siah-1 subcellular localization following treatment for 48 h. The number of cells positive for nuclear siah-1 were quantified, and the values from independent experiments were graphed as the means ± S.E. (n = 4; *, p < 0.05). Dapi, 4′,6′-diamino-2-phenylindole.
Detection of High Glucose-induced siah-1-GAPDH Complex in the Nucleus of rMC-1
A recent study suggested that formation of a complex between siah-1 and GAPDH is essential in the process of GAPDH nuclear accumulation (13). Because we have demonstrated that high glucose up-regulates siah-1, we were interested in examining whether such a complex can be detected in the nucleus of Müller cells, further identifying a potential role for siah-1 as a shuttle protein for GAPDH to allow for translocation to the nucleus.
First, several immunoprecipitation control experiments were performed. Fig. 3A shows that immunoprecipitation using goat serum did not pull down siah-1 or GAPDH. Immunoprecipitation of beads only also did not show any unspecific pulldown of siah-1 and GAPDH (data not shown). The siah-1 antibody used for immunoprecipitation assays was specific for siah-1, because pulldown of siah-1 and subsequent analysis of the immunoprecipitates for siah-1 using Western blot analysis detected only the siah-1 protein. To confirm that GAPDH does not just bind to proteins in an unspecific manner, immunoprecipitation assays using LDH were performed, because retinal Müller cells highly express LDH. Fig. 3A shows that GAPDH binds to siah-1 but not to the abundant LDH protein. Input levels of siah-1 and GAPDH are presented as control. Finally, the siah-1 Western blot analysis in the right panel of Fig. 3A demonstrates that lysates subjected to siah-1 immunoprecipitation are devoid of the protein, indicating that pulldown of siah-1 was efficient and complete under our experimental conditions.
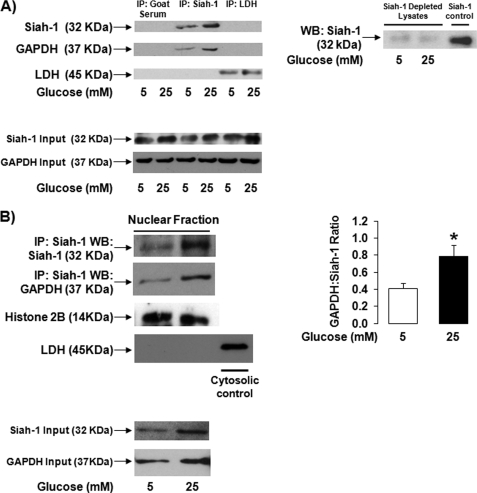
High glucose induces complex formation between GAPDH and siah-1 detectable in the nucleus of Müller cells. A, rMC-1 cells cultured under normal (5 mm) or high (25 mm) glucose conditions for 12 h were subjected to goat serum, siah-1, or LDH immunoprecipitation followed by Western blot analysis. The membranes were probed for siah-1 and LDH to confirm protein pulldown. The membranes were then stripped and reprobed for GAPDH to evaluate the specificity of GAPDH binding to siah-1 (n = 3). As control, input levels of siah-1 and GAPDH are presented. To determine the efficiency of siah-1 protein pulldown, lysates devoid of siah-1 because of siah-1 immunoprecipitation were subjected to siah-1 Western blot analysis (n = 3) (right panel). B, rMC-1 cells were cultured under normal (5 mm) or high (25 mm) glucose conditions for 24 h. Crude nuclear fractions were generated from treated cells, and equal amounts of lysate were assessed for GAPDH-siah-1 complex formation using co-immunoprecipitation analysis. siah-1 immunoprecipitates were first probed against siah-1. Stripped membranes were reprobed for GAPDH. Fraction purity was assessed using nuclear marker histone 2B and cytosolic marker LDH. The images are representative of results from three independent experiments. The ratio of GAPDH to siah-1 binding under treatment conditions was calculated. The results are presented as the means ± S.E. (n = 3; *, p < 0.05). IP, immunoprecipitation; WB, Western blot.
To demonstrate the presence of a siah-1-GAPDH complex in the nucleus of Müller cells, co-immunoprecipitation studies were done using equal amounts of nuclear fractions from rMC-1 cells subjected to normal or high glucose treatment. The 24-h treatment time point was chosen, because this is the time point at which GAPDH nuclear accumulation can significantly be detected (16). High glucose treatment led to a significant increase in the amounts of siah-1 proteins in the nucleus and increased levels of nuclear GAPDH bound to siah-1 (Fig. 3B). To determine whether increased levels of nuclear GAPDH bound to siah-1 were due to a potential increased binding of GAPDH to siah-1, ratios of GAPDH to siah-1 protein levels were calculated based on densitometry analysis of Western blots. High glucose treatment significantly increased the ratio of GAPDH:siah-1 binding by 1.9 ± 0.3-fold based on this analysis (Fig. 3B).
Effect of siah-1 Knockdown by siah-1 siRNA on siah-1 and GAPDH Protein Level
Although the co-immunoprecipitation assays indicated the formation of a complex between siah-1 and GAPDH under hyperglycemic conditions, which was detectable in the nucleus of Müller cells, we opted to use siRNA technology to knock down siah-1 to further confirm the necessity of siah-1 in the process of GAPDH translocation from the cytosol to the nucleus. 50 nm siah- 1 siRNA significantly decreased siah-1 mRNA expression at 20 h by 63.9 ± 8.5% compared with electroporation only (Fig. 4A). Scrambled siRNA had no significant effect on siah-1 mRNA levels. In addition, 50 nm siah-1 siRNA decreased siah-1 protein levels by 48.3 ± 11.8% compared with electroporation only (Fig. 4B). Scrambled and risk-free siRNA had no apparent significant effect on siah-1 protein levels. siah-1 knockdown did not affect GAPDH protein levels (Fig. 4B).
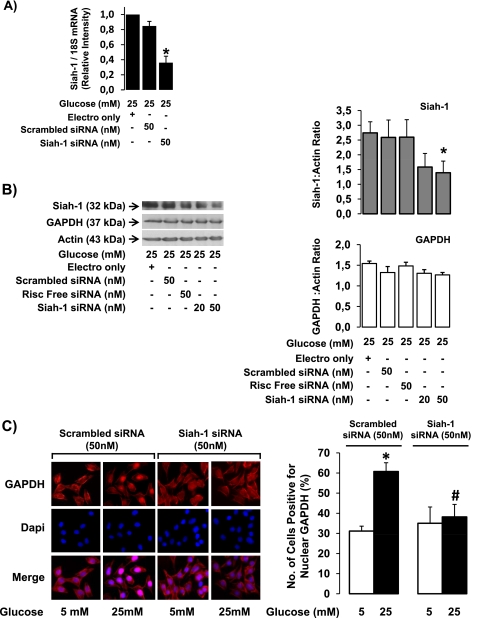
High glucose-induced GAPDH nuclear accumulation is decreased following siah-1 knockdown using siRNA. rMC-1 transfected with 50 nm scrambled control siRNA, 50 nm risk-free control siRNA, 20 nm siah-1 siRNA, or 50 nm siah-1 siRNA were treated in high (25 mm) glucose. A, at 20 h post-transfection, siah-1 mRNA levels were quantified using real time quantitative PCR analysis. The results are presented as the means ± S.E. (n = 4; *, p < 0.05). B, siah-1 protein levels were determined using Western blot analysis at 48 h post-transfection. The membranes were stripped and reprobed for GAPDH and actin. siah-1 and GAPDH were quantified using densitometry analysis, normalized to actin, and graphed as the means ± S.E. (n = 4; *, p < 0.05). C, rMC-1 cells transfected with either scrambled control siRNA (50 nm) or siah-1 siRNA (50 nm) were treated in normal (5 mm) or high (25 mm) glucose for 24 h. Following treatment, the cells were processed for GAPDH immunofluorescence analysis, and the cells positive for nuclear GAPDH were counted. The results represent the means ± S.E. (n = 4; *, p < 0.05 compared with scrambled control siRNA normal glucose; #, p < 0.05 compared with scrambled control siRNA high glucose).
Effect of siah-1 Knockdown on GAPDH Nuclear Accumulation
Because we had established that 50 nm siRNA significantly reduced siah-1 protein levels, this concentration was used for further experiments. To test whether siah-1 knockdown can prevent high glucose-induced GAPDH nuclear accumulation, the levels of nuclear GAPDH were determined following siah-1 knockdown in high glucose-treated retinal Müller cells. The number of rMC-1 cells positive for nuclear GAPDH following high glucose treatment significantly decreased from 60.7 ± 4.3% in scrambled siRNA-transfected control cells to 36.6 ± 5.2% in siah-1 siRNA-transfected cells, strongly indicating that siah-1 is necessary for GAPDH nuclear translocation (Fig. 4C). The number of GAPDH-positive cells in the normal scrambled control siRNA control cells was 31.1 ± 2.5%.
GAPDH-siah-1 Interaction Is Necessary for Nuclear Accumulation under High Glucose Conditions
A previous study has shown that a 12-amino acid truncation of the siah-1 C terminus prevented complex formation with GAPDH (13). These 12 amino acids are part of the substrate-binding domain. Using a truncated form of siah-1, we confirmed the necessity of GAPDH-siah-1 interaction for high glucose-induced GAPDH nuclear accumulation. Cells transfected with truncated siah-1 lacking residues 271–282 (siah-1 Δ amino acids 1–270) did not show any increase in the number of cells positive for nuclear GAPDH following high glucose stimulation compared with control. In contrast, cells transfected with vector only showed a significant increase of 55.6 ± 10.5% in cells positive for nuclear GAPDH when exposed to high glucose compared with control (Fig. 5A). Transfection of Müller cells with wild type siah-1 leads to a similar increase of cells positive for nuclear GAPDH as observed in cells transfected with vector only following high glucose stimulation (data not shown). As control, Fig. 5B shows that GAPDH does not increasingly bind to truncated siah-1 in hyperglycemic conditions as determined by Western blot, although overall unspecific binding of GAPDH to siah-1 seemed to have been elevated. High glucose led to a significant 1.65 ± 0.36-fold increase in the ratio of GAPDH binding to siah-1 in vector only treated cells compared with cells transfected with truncated siah-1 (0.06 ± 0.02). These data provide further evidence that GAPDH siah-1 interaction is crucial for high glucose-induced GAPDH nuclear translocation in retinal Müller cells.
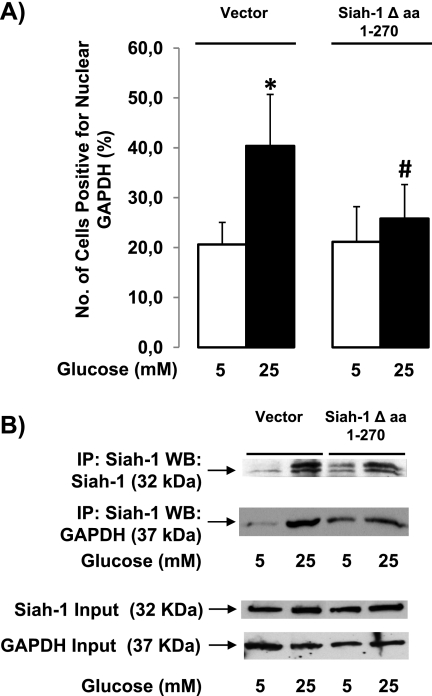
Truncation of siah-1 prevents GAPDH binding and translocation to the nucleus. A, rMC-1 cells transfected with either control vector (10 μg) or siah-1Δ amino acids 1–270 (10 μg) were treated in normal (5 mm) or high (25 mm) glucose for 24 h. Following treatment, the cells were processed for GAPDH immunofluorescence analysis, and the cells positive for nuclear GAPDH were counted. The results represent the means ± S.E. (n = 8; *, p < 0.05 compared with cells transfected with vector only in normal glucose; #, p < 0.05 compared with high glucose treated cells transfected with vector only). B, rMC-1 cells transfected with control vector (10 μg) or siah-1Δ amino acids 1–270 (10 μg) were treated in normal (5 mm) or high (25 mm) glucose for 12 h. The cells were subjected siah-1 immunoprecipitation followed by siah-1 Western blot analysis after treatment. The membranes were then stripped and reprobed for GAPDH to evaluate the effect of siah-1 truncation on GAPDH siah-1 complex formation (n = 3). The Western blot is representative of four independent experiments. IP, immunoprecipitation; WB, Western blot.
The Role of siah-1 Knockdown on p53 Phosphorylation under High Glucose Conditions
So far, we have demonstrated that siah-1 is necessary for GAPDH nuclear translocation. Although nuclear GAPDH has strongly been linked to cell death induction, the function of GAPDH in the nucleus is unknown and has only been speculated about. To date, only one study has proposed a mechanism for cell death induction by nuclear GAPDH. This recent report suggested that GAPDH plays an integral role in the acetylation of p53, a well known protein involved in a variety of cell death processes, connecting nuclear siah-1 and GAPDH to cell death induction (15). Because transcriptional activity of p53 is also heavily regulated by phosphorylation, we examined the effect of siah-1 knockdown on p53 phosphorylation at serine 15. Serine 15 is the most common phosphorylation site of p53 leading to p53 transcriptional activity during cell death. High glucose increased phosphorylation of serine 15 by 17.5 ± 3.1% compared with control cells. siah-1 knockdown, which prevents GAPDH from entering the nucleus, inhibited p53 phosphorylation at serine 15, indicating that nuclear GAPDH might regulate p53 transcriptional activity (Fig. 6A). siah-1 knockdown also prevented high glucose-induced acetylation of p53 (data not shown). To test whether changes in the phosphorylation state of p53 at serine 15 indeed induce transcriptional activity of p53 and whether siah-1 knockdown can prevent p53 transcriptional activity, we determined protein expression levels for the well known p53-driven target gene bax. A significant 21.2 ± 0.6% increase in bax protein was observed following high glucose treatment compared with normal glucose in the control scrambled siRNA-treated cells (Fig. 6B), whereas siah-1 knockdown using siRNA reduced high glucose-induced bax levels by 75.2 ± 3.8%.
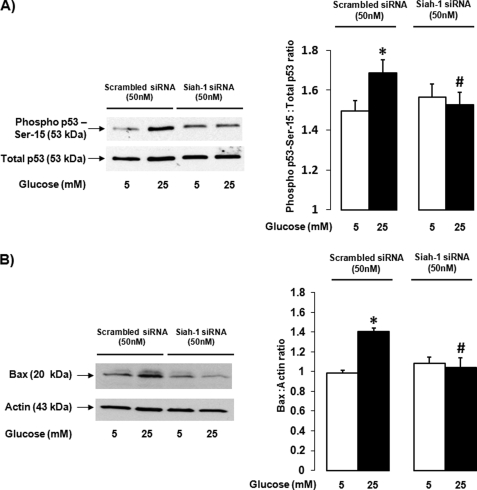
siah-1 knockdown decreases high glucose-induced p53 phosphorylation and bax up-regulation. rMC-1 cells transfected with scrambled control siRNA (50 nm) or siah-1 siRNA (50 nm) were treated in normal (5 mm) or high (25 mm) glucose for 24 h. A, following treatment, p53 phosphorylation at serine 15 was determined using Western blot analysis of total lysates. The membranes were stripped and reprobed for total p53. Phospho-p53 Western blots were quantified using densitometry analysis, normalized to total p53, and graphed as the means ± S.E. (n = 5; *, p < 0.05 compared with cells transfected with scrambled siRNA in normal glucose; #, p < 0.05 compared with cells transfected with scrambled siRNA in high glucose). B, total lysates generated from treated cells were assessed for bax protein levels using Western blot analysis. The membranes were stripped and reprobed for actin. Bax Western blots were quantified using densitometry analysis, normalized to actin and graphed as the means ± S.E. (n = 3; *, p < 0.05 compared with cells transfected with scrambled siRNA in normal glucose; #, p < 0.05 compared with cells transfected with scrambled siRNA in high glucose).
Effect of siah-1 Knockdown on High Glucose-induced Cell Death in Müller Cells
To reinforce the idea that GAPDH nuclear translocation leads to cell death under high glucose conditions, the effect of siah-1 knockdown on high glucose-induced cell death was determined using caspase-6 activity and trypan blue viability assays. High glucose significantly increased the activity of caspase-6, an executioner caspase, to 166.8 ± 4.2 pmol 7 amino-4-trifluoromethylcoumarin/mg/min from 118.3 ± 11.5 in control cells. siah-1 siRNA knockdown reduced high glucose-induced caspase-6 activity to 123.6.±13.6% (Fig. 7A). Further, high glucose treatment increased cell death by 4.5 ± 2.0-fold. High glucose-induced cell death was diminished in cells subjected to siah-1 siRNA, indicating that inhibition of GAPDH nuclear translocation within the first 24 h strongly affects cell death detectable at 96 h (Fig. 7B).
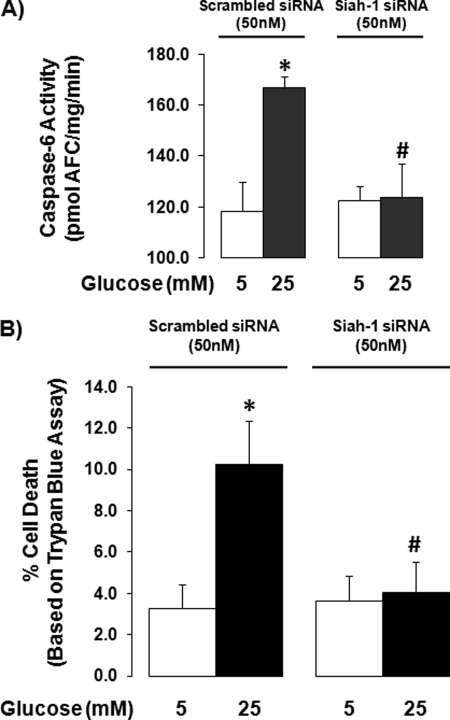
Inhibition of high glucose-induced cell death by siah-1 knockdown. rMC-1 cells transfected with scrambled control siRNA (50 nm) or siah-1 siRNA (50 nm) were treated in normal (5 mm) or high (25 mm) glucose for 96 h. A, following treatment, caspase-6 activity was measured. The results represent the means ± S.E. (n = 8; *, p < 0.05 compared with scrambled siRNA normal glucose; #, p < 0.05 compared with scrambled siRNA high glucose). B, cell viability was determined using trypan blue viability assays. The results represent the means ± S.E. (n = 8; *, p < 0.05 compared with scrambled siRNA normal glucose; #, p < 0.05 compared with scrambled siRNA high glucose).
DISCUSSION
Hyperglycemia causes GAPDH nuclear translocation and accumulation in retinal Müller cells in vivo and in vitro (4, 16). The mechanism underlying the movement of GAPDH from the cytosol to the nucleus under high glucose conditions in these cells is not completely understood. In the present study, we have demonstrated that the E3 ubiquitin ligase siah-1 facilitates GAPDH nuclear translocation via formation of a complex with GAPDH. In contrast to GAPDH, siah-1 carries a NLS motif allowing for transport to the nucleus.
More importantly, siah-1 knockdown studies and studies using a truncated form of siah-1 have confirmed that siah-1 is necessary and crucial for the processes of high glucose-induced GAPDH nuclear accumulation and subsequent cell death in Müller cells. Although the exact function of GAPDH in the nucleus is largely unknown, our results indicate that nuclear GAPDH seems to be involved in the regulation of p53 activation.
Our current studies provide evidence that siah-1 plays a critical role in the process of GAPDH nuclear translocation. However, it is somehow surprising that this type of protein is critically involved in this process. siah-1 is most commonly associated with its E3 ubiquitin ligase function during ubiquitin-dependent proteasomal degradation (24, 26, 28, 48,–50). In diabetic retinopathy, proteins associated with ubiquitination and protein turnover are up-regulated during the development of the disease (51). The glucose transporter GLUT1 and angiotensin II type 1 receptor have been identified as specific targets for protein degradation through this pathway in the diabetic retina. In addition to tagging proteins for proteasomal degradation, post-translational modification via ubiquitination may also act as a signaling mechanism. Increased ubiquitination has been associated with the progression of neurodegenerative diseases. For example, ubiquitination by siah-2 has been shown to induce α- synuclein aggregation and cytotoxicity in neuronal cells during the development of Parkinson disease, and siah-1 auto-ubiquitination has been suggested to occur during complex formation between GAPDH and siah-1 (13, 29).
Other functions of siah-1 involve regulation of cell cycle and pro-survival proteins through protein degradation (22, 24, 49, 52). Whether inhibition of high glucose-induced cell death of Müller cells by siah-1 knockdown results from the inhibition of these alternative functions of siah-1 is not known. Future studies need to be done to determine the role of ubiquitination in this process. The focus of our study was to understand the role of siah-1 in the process of GAPDH nuclear translocation and subsequent cell death induction. Although humans and rats have two siah-1 homologs: siah-1 and siah-2 (17, 30, 31), our studies have focused on siah-1, because siah-2 does not have a NLS motif based on sequence prediction analysis.
Even though GAPDH does not contain a NLS, the protein contains an export signal for protein extrusion from the nucleus (53). It is possible that the absence of GAPDH in Müller cell nuclei under normal glucose conditions results from efficient nuclear to cytosolic export. Impaired protein export in high glucose conditions might also be responsible for accumulation of GAPDH in the nucleus. Conformational changes to GAPDH following siah-1 binding may also affect the ability of GAPDH to interact with the export machinery of the nucleus.
Although the translocation and accumulation of GAPDH has been linked to cell death induction, the function(s) of GAPDH within the nucleus have not fully been identified. Studies in neurons that made use of an NLS-tagged GAPDH construct indicate that simple localization of GAPDH in the nucleus might not be sufficient to initiate cell death (54). Post-translational modifications of GAPDH appear to be necessary for nuclear import and the cell death functions of this protein (11, 13, 14, 55). Several studies suggest that nitric oxide is a key regulator of these post-translational modifications (12,–15, 55, 56). Some of these post-translational modifications of GAPDH have recently been demonstrated in nuclear extracts of retinas obtained from diabetic rats (11). We have previously shown that the caspase-1/interleukin-1β signaling pathway mediates hyperglycemia-induced GAPDH nuclear accumulation in Müller cells (57). Interleukin-1β is very well known to induce NO production, and high glucose-induced NO production has been demonstrated in Müller cells (57), indicating the possibility of NO-mediated post-translational modification of GAPDH during GAPDH nuclear translocation. Interestingly, inhibition of the caspase-1/interleukin-1β pathway prevents high glucose-induced GAPDH-siah-1 complex formation (data not shown).
One newly identified potential function of GAPDH in the nucleus seems to involve the regulation of p53 activation, a well known protein heavily involved in induction of several types of cell death (15). A recent study has suggested that the process involves activation of p53 via acetylation by p300/CBP subsequent to GAPDH-mediated activation of these acetylation enzymes (15). Our studies also suggest that in addition to acetylation of p53, nuclear GAPDH might also regulate phosphorylation of p53, which stabilizes p53. The role of siah-1 in both of these processes, acetylation and phosphorylation of p53, has not been determined and might just be related to transport of GAPDH to the nucleus. On the other hand, a previous study not focused on the interaction between GAPDH and siah-1 has demonstrated a role for siah-1 itself in the process of p53 activation (22). siah-1 and p53 seem to be connected and regulated via a positive feedback mechanism because siah-1 is also regulated by p53 (58). More studies are needed to clearly identify the role of nuclear siah-1 and GAPDH in the process of cell death induction.
Retinal Müller cells maintain the retina and its vasculature (34,–39, 59). Therefore, Müller cell dysfunction and loss in the diabetic retina (60) possibly compromises the integrity of retinal tissue leading to disease. GAPDH nuclear accumulation might play an integral role in the induction of cell loss within the diabetic retina. A recent report has demonstrated that GAPDH nuclear accumulation persists when diabetic animals were brought back from poor to good control of blood glucose levels (11). The study also indicated that in these animals progression of diabetic retinopathy was still present. Although our studies have focused on identifying mechanisms underlying GAPDH nuclear accumulation in Müller cells in vitro and in vivo (4, 16), we do not want to exclude the possibility that other retinal cells are affected by hyperglycemia in a similar fashion. High glucose induces cell death in several other retinal cell types in the diabetic retina (10, 61,–65). Inhibition of GAPDH nuclear translocation is clearly protective. We have previously shown that the mono amino oxidase inhibitor R-deprenyl prevents GAPDH nuclear accumulation and cell death in Müller cells cultured under high glucose conditions (4). Recently, a study has demonstrated that R-deprenyl acts by interfering with GAPDH-siah-1 interaction (9). Taken together, our results indicate the potential of compounds that target the GAPDH/siah-1 interaction as therapies to increase Müller cell survival under hyperglycemic conditions and potentially prevent the progression of diabetic retinopathy.
Acknowledgments
We thank Jason Vincent and Scott Howell for technical assistance.
*This work was supported, in whole or in part, by National Institutes of Health Training Grants 2T32EY007157 and 1F31EY018075 (to E. Y.) and Research Grants: EY-014380 and EY-017206 (to S. M.). This work was also supported by Visual Science Research Center Core Grant 2P30EY011373 (to E. Y. and S. M.) and by American Diabetes Association Grant 7-06-RA-95 (to S. M.).
2The abbreviations used are:
- GAPDH
- glyceraldehyde-3-phosphate dehydrogenase
- rMC-1
- transformed rat retinal Müller cell line
- hMC
- isolated human Müller cells
- LDH
- lactate dehydrogenase
- siRNA
- small interfering RNA
- NLS
- nuclear localization signal
- E3
- ubiquitin-protein isopeptide ligase
- PBS
- phosphate-buffered saline.
REFERENCES
Articles from The Journal of Biological Chemistry are provided here courtesy of American Society for Biochemistry and Molecular Biology
Full text links
Read article at publisher's site: https://doi.org/10.1074/jbc.m109.083907
Read article for free, from open access legal sources, via Unpaywall:
http://www.jbc.org/article/S002192582064813X/pdf
Free after 12 months at intl.jbc.org
http://intl.jbc.org/cgi/reprint/285/5/3181.pdf
Free after 12 months at intl.jbc.org
http://intl.jbc.org/cgi/content/full/285/5/3181
Free to read at intl.jbc.org
http://intl.jbc.org/cgi/content/abstract/285/5/3181
Citations & impact
Impact metrics
Citations of article over time
Article citations
Interleukin-1 receptor-dependent and -independent caspase-1 activity in retinal cells mediated by receptor interacting protein 2.
Front Cell Dev Biol, 12:1467799, 16 Oct 2024
Cited by: 0 articles | PMID: 39483336 | PMCID: PMC11525982
The uncommon function and mechanism of the common enzyme glyceraldehyde-3-phosphate dehydrogenase in the metamorphosis of Helicoverpa armigera.
Front Bioeng Biotechnol, 10:1042867, 18 Oct 2022
Cited by: 2 articles | PMID: 36329701 | PMCID: PMC9623274
The mutual interaction of glycolytic enzymes and RNA in post-transcriptional regulation.
RNA, 28(11):1446-1468, 16 Aug 2022
Cited by: 4 articles | PMID: 35973722 | PMCID: PMC9745834
Review Free full text in Europe PMC
Mechanisms of β-adrenergic receptors agonists in mediating pro and anti-apoptotic pathways in hyperglycemic Müller cells.
Mol Biol Rep, 49(10):9473-9480, 04 Aug 2022
Cited by: 5 articles | PMID: 35925485
Moonlighting Proteins: The Case of the Hexokinases.
Front Mol Biosci, 8:701975, 09 Jun 2021
Cited by: 26 articles | PMID: 34235183 | PMCID: PMC8256278
Review Free full text in Europe PMC
Go to all (37) article citations
Data
Similar Articles
To arrive at the top five similar articles we use a word-weighted algorithm to compare words from the Title and Abstract of each citation.
Nuclear translocation of glyceraldehyde-3-phosphate dehydrogenase: a role in high glucose-induced apoptosis in retinal Müller cells.
Invest Ophthalmol Vis Sci, 45(5):1553-1561, 01 May 2004
Cited by: 69 articles | PMID: 15111614
Paraquat exposure induces nuclear translocation of glyceraldehyde-3-phosphate dehydrogenase (GAPDH) and the activation of the nitric oxide-GAPDH-Siah cell death cascade.
Toxicol Sci, 116(2):614-622, 17 May 2010
Cited by: 15 articles | PMID: 20478973
E3 ubiquitin ligase siah‑1 nuclear accumulation is critical for homocysteine‑induced impairment of C6 astroglioma cells.
Mol Med Rep, 20(3):2227-2235, 01 Jul 2019
Cited by: 5 articles | PMID: 31322210 | PMCID: PMC6691270
Nitric oxide-GAPDH-Siah: a novel cell death cascade.
Cell Mol Neurobiol, 26(4-6):527-538, 22 Apr 2006
Cited by: 114 articles | PMID: 16633896
Review
Funding
Funders who supported this work.
NEI NIH HHS (10)
Grant ID: 2T32EY007157
Grant ID: EY-017206
Grant ID: R03 EY014380
Grant ID: F31 EY018075
Grant ID: R01 EY017206
Grant ID: EY-014380
Grant ID: T32 EY007157
Grant ID: 2P30EY011373
Grant ID: P30 EY011373
Grant ID: 1F31EY018075