Abstract
Free full text

Endogenous antibodies promote rapid myelin clearance and effective axon regeneration after nerve injury
Abstract
Degenerating myelin inhibits axon regeneration and is rapidly cleared after peripheral (PNS) but not central nervous system (CNS) injury. To better understand mechanisms underlying rapid PNS myelin clearance, we tested the potential role of the humoral immune system. Here, we show that endogenous antibodies are required for rapid and robust PNS myelin clearance and axon regeneration. B-cell knockout JHD mice display a significant delay in macrophage influx, myelin clearance, and axon regeneration. Rapid clearance of myelin debris is restored in mutant JHD mice by passive transfer of antibodies from naïve WT mice or by an anti-PNS myelin antibody, but not by delivery of nonneural antibodies. We demonstrate that degenerating nerve tissue is targeted by preexisting endogenous antibodies that control myelin clearance by promoting macrophage entrance and phagocytic activity. These results demonstrate a role for immunoglobulin (Ig) in clearing damaged self during healing and suggest that the immune-privileged status of the CNS may contribute to failure of CNS myelin clearance and axon regeneration after injury.
During the process of wound healing, rapid and efficient clearance of cellular debris is necessary for tissue regeneration (1). Myelin debris remains in white matter tracks years after an injury to the CNS in humans and primates (2, 3). The prolonged presence of myelin-associated inhibitors of axon regeneration is thought to contribute to the lack of recovery following CNS injury. Although peripheral myelin also contains inhibitors of axon regeneration, PNS myelin is rapidly cleared after injury, thereby permitting rapid axon regeneration (4–6). It is not known why the rates of clearance of PNS and CNS are so different (7).
Antibodies, like other opsonins, coat exogenous debris and pathogens, thereby targeting them for clearance by phagocytes. The recognition of self antigens by natural antibodies produced by B1 cells is well documented (8). Although it is hypothesized that these antibodies may have a physiological role other than immune defense, their role in clearing necrotic cellular debris is not known (8). Therefore, we tested whether endogenous antibodies contribute to rapid removal of degenerating myelin after PNS injury, thereby promoting axon regeneration.
Results
Antibodies Accumulate in Sciatic Nerve After Injury.
To investigate whether endogenous antibodies aid in removal of myelin debris, we first examined whether antibodies accumulate in peripheral nerves following injury. We compared nerve injury responses between WT and JHD mice, which have a targeted deletion of the JH locus that prevents VDJ recombination and the formation of mature B-cells and antibodies (9). Control and crushed sciatic nerves were obtained from WT and JHD mice and stained with anti-mouse Ig antibodies. Six days after crush injury, we observed a strong increase in immunoreactivity for Ig on degenerating myelin sheathes distal to the site of injury in WT but not in JHD nerves (Fig. S1A). To quantify the amount of antibody accumulation, we performed Western blotting of lysates prepared from the degenerating nerves at several time points following injury (Fig. S1B). In uninjured nerves, we detected only low levels of albumin and Ig. These findings indicate that after injury, IgG and IgM antibodies accumulate within injured nerve segments at higher levels than do other serum proteins such as albumin.
JHD Mice Lacking B-Cells Have Delayed Myelin Clearance After Injury.
To assess the normal timing of clearance of myelin proteins after injury, we examined the levels of a major myelin protein, peripheral myelin protein zero (P0), by Western blotting of lysates prepared from the distal segments of crushed murine sciatic nerves. P0 levels decreased steadily following injury and reached very low levels by 10 d after injury (Fig. S1B), a rate similar to that observed in previous studies (10). The rate of myelin clearance, as indicated by loss of P0 protein, correlated with the accumulation of Ig in the degenerating nerve. These results demonstrate that myelin is rapidly cleared at about the same time that antibodies enter and accumulate in the injured nerve, raising the possibility that these antibodies may target myelin for clearance by peripheral macrophages.
To test whether B-cells are required for rapid PNS myelin clearance during Wallerian degeneration (WD), we compared the rate of myelin protein clearance following sciatic nerve crush in WT and JHD mice. We harvested distal nerves from these mice at several time points after crush and stained nerve sections with the lipophilic dye FluoroMyelin to visually assess the amount of myelin. At 10 d following injury, JHD nerves contained numerous large ovoids of lipid debris, whereas WT nerves had few if any (Fig. 1A). To quantitatively assess the rate of myelin clearance, we assayed myelin basic protein (MBP) and P0 levels by Western blotting lysates of distal sciatic nerves after crush. At 10 d postcrush in WT mice, little or no MBP and P0 protein remained, whereas in the JHD mice, significant amounts of MBP and P0 were still present, indicating a delay in their clearance (Fig. 1B). To better understand the timing of myelin clearance in the JHD mice, we repeated the experiment with a detailed time course and found that JHD mice exhibit a 1-wk delay in MBP and P0 clearance as compared to WT mice (Fig. 1 C and D). To test for the effect of genetic background on the delay of myelin clearance in JHD mice, we backcrossed BALB/c JHD mice into C57BL6 for 6–7 generations. C57BL6 JHD phenocopied BALB/c JHD in their clearance of myelin proteins (Fig. S2).

Myelin clearance is delayed in B-cell–deficient mice. (A) Staining of paraformaldehyde-fixed cryosections obtained from control and crushed sciatic nerves at 6 and 10 d postcrush (DPC) using the FluoroMyelin dye (red) at a dilution of 1:300. Arrowheads mark degenerating myelin ovoids. (B) Western blot of lysates obtained from WT and JHD sciatic nerves probed with anti-IgM, anti-P0, anti-MBP, and anti-FcR common γ chain antibodies. (C) Quantification by Western blotting of MBP levels in WT and JHD sciatic nerve lysates both following sciatic nerve injury and in uncrushed control nerves. (D) Quantification of P0 levels in WT and JHD mice after injury. Total protein was assessed by the BCA assay and each well was loaded with the same amount of total nerve protein. All data are from male mice and are presented as mean ± SEM, n = 4–10 animals per genotype per time point (*P < 0.05; **P < 0.01). (Scale bar, 200 μm.)
Previous studies have shown that myelin is cleared in two distinct phases in WT mice: a rapid phase for the first 6 d, during which about two-thirds of the myelin is cleared, followed by a slower phase during the second week when the remaining third of the myelin is cleared. These phases are mediated by Schwann cells and macrophages, respectively (4, 6, 11). During the first 6 d after injury, the rate of both MBP and P0 clearance did not differ between WT and JHD mice. However, after 6 d following injury, JHD mice exhibited a striking delay in the rate of myelin clearance. Thus the delay in myelin clearance observed in the JHD mice is coincident with the second, slower phase of myelin clearance (6). These data support a model in which two mechanistically distinct processes of myelin debris clearance occur: an initial process mediated by Schwann cells that is B-cell independent and a latter process mediated by macrophages, that is strongly B-cell dependent.
Bone Marrow Transplant Rescues Delayed Myelin Clearance.
To confirm that the delay in myelin clearance in the JHD mice was specifically caused by the lack of humoral immunity and not by development differences that might affect the rate of WD independently of marrow-derived cells, we next performed bone marrow transplants (BMT) to reconstitute the hematopoietic system in JHD mice. We transplanted WT whole bone marrow into lethally irradiated adult JHD and WT control mice. Six weeks following transplantation, we harvested serum samples from the transplanted animals to test for the presence of serum IgG and IgM, indicators of the presence of functional B-cells (Fig. 2A). All JHD mice reconstituted with WT bone marrow had readily detectable levels of serum Ig. We performed sciatic nerve crushes on the reconstituted animals 7 wk after BMT and harvested the nerves 10 d later to assess the timing of myelin clearance. JHD mice reconstituted with WT bone marrow were able to clear myelin proteins as efficiently as WT mice (Fig. 2B). These results demonstrate that slow myelin clearance in JHD mice is due to a hematopoietic defect and not a result of intrinsic nerve or developmental differences. We conclude that mature B-cells are necessary for fast myelin clearance after PNS injury.

Rapid myelin clearance in JHD mice is restored by endogenous antibodies. (A and B), JHD and WT mice underwent bone marrow transplantation (BMT) with WT whole bone marrow. Sciatic nerve crush was performed 7 wk following BMT. (A) Western blot of serum from WT and JHD mice collected before and after BMT, probed with anti-IgG, anti-IgM, and anti-albumin antibodies. (10 μg total protein per lane) (B) Western blot of sciatic nerve lysates from WT and JHD mice at 10 d postcrush probed with anti-MBP, anti-P0, and anti-β-actin antibodies (500 ng total protein per lane). (C) Western blot of sciatic nerve lysate samples from JHD mice probed with MBP and albumin antibodies. Samples were taken from uncrushed nerves, crushed JHD nerves, and nerves harvested from crushed JHD mice after passive transfer of serum, purified IgM and IgG, anti-P0, anti-GFP, or anti-firefly luciferase antibody (1 μg total protein per lane). (D) Average level of MBP protein relative to JHD control nerve at 10 d following injury in JHD mice that received passive transfer of serum, purified IgM or IgG, or monoclonal antibody [via i.p. injection at 2 and 6 d postcrush (DPC)]. All data are from male mice and are presented as mean ± SEM, n = 8–9 animals (*P < 0.05; **P < 0.01).
Passive Transfer of Serum, Purified IgG, and Purified IgM Reconstitutes Rapid Myelin Clearance in JHD Mice.
Delayed myelin clearance in JHD mice, which lack both mature B-cells and antibodies, could be due to several factors. B-cells might modulate the activity of macrophages locally at the injured nerve; alternatively, antibodies produced by B-cells might promote clearance of myelin debris by opsonization. To determine whether antibodies alone are sufficient to speed WD in the PNS of JHD mice, we performed passive antibody transfer experiments with whole sera, purified IgG, or purified IgM from naïve WT mice. IgM isotype Igs constitute the majority of natural antibodies, making them likely candidates if natural antibodies proved to be sufficient to promote clearance. Serum or purified Ig was injected into JHD mice via i.p. injection at 2 and 6 d after injury. At 10 d following injury, a time corresponding to when myelin protein clearance is complete in WT mice but delayed in JHD mice, we harvested nerve lysates and assessed MBP levels via Western blotting. We also collected serum samples to confirm the presence of antibodies in the blood (Fig. S3). We found that passive transfer with whole sera, purified IgG, or purified IgM from naïve WT mice was sufficient to rescue myelin clearance in JHD mice (Fig. 2 C and D). These results demonstrate that passive transfer of either whole serum or purified Ig in the absence of mature B-cells is sufficient to rescue rapid myelin clearance.
To determine whether there is also a B-cell mediated adaptive immune-response component in promoting rapid myelin clearance, we repeated the passive transfer experiments with purified IgG collected from WT mice that experienced a sciatic nerve crush 14 d earlier. JHD mice receiving purified IgG from postcrush WT mice cleared myelin as rapidly as JHD mice receiving precrush serum and Ig (Fig. 2 C and D). Together these experiments indicate that endogenous preexisting IgG and IgM antibodies present in naïve WT mice can rescue defects in myelin clearance in JHD mice.
To determine the fine specificity of the endogenous antibodies to myelin, we harvested serum samples taken from mice before and 14 d after crush injury and used these samples to probe a myelin proteome microarray (12). Even before crush, we found antibodies specific to several myelin antigens such as myelin associated glycoprotein (MAG) and 2,3-Cyclic Nucleotide 3 Phosphodiesterase (CNPase) present in sera and did not detect increased titers of myelin-specific antibodies or epitope spreading after injury (Fig. S4). These experiments further indicate that preexisting IgG and IgM present in naïve WT mice help to promote rapid PNS myelin clearance.
Passive Transfer of Anti-Myelin Antibodies Rescues Rapid Myelin Clearance in JHD Mice.
To examine whether delivery of an exogenous myelin-specific antibody would be sufficient to promote rapid myelin clearance, a monoclonal antibody directed against P0 was passively transferred into JHD mice and was able to induce robust myelin clearance after nerve injury (Fig. 2 C and D). In contrast, passive transfer of sera from JHD mice or monoclonal antibodies against either GFP or firefly luciferase failed to promote rapid myelin clearance in JHD mice (Fig. 2 C and D). Thus Ig is a crucial serum component required for robust myelin clearance and antibodies directed specifically against myelin epitopes are sufficient to induce rapid clearance of myelin debris. Taken together, these findings provide strong evidence for the existence of endogenous antibodies specific to neural epitopes that assist in removal of damaged self after trauma. Although these endogenous antibodies are likely to be natural preexisting antibodies, we cannot exclude the possibility that early postnatal injuries lead to the development of anti-myelin antibodies before the initiation of these experiments.
Passive Transfer Rescues Delayed Macrophage Entry Injured Sciatic Nerve.
To determine whether B-cells and/or antibodies are needed to promote macrophage influx into the degenerating nerve, we examined the rate of macrophage infiltration into the nerve following injury in WT and JHD mice. We quantified the number of macrophages in the nerve at several time points by immunostaining using the macrophage-specific anti-F4/80 and anti-CD68 antibodies (Fig. 3 A and B). The sciatic nerves of JHD mice contained about 50% fewer macrophages than those of WT mice at 2 and 6 d following injury (Fig. 3B). The number of macrophages in the nerve reached its peak around 6 d after injury in WT mice but did not do so until 10 d after injury in the JHD mice, indicating a significant delay in macrophage influx into the JHD nerves. This delay cannot be explained by an abnormality intrinsic to the macrophages of the JHD mice, as macrophage development in these animals is normal (13). Notably, the macrophages that do invade the sciatic nerves of JHD mice 6 d after injury display diminished phagocytic morphology and lower levels of lysozyme expression than those in WT nerves, suggesting that in the absence of endogenous antibodies, macrophages have decreased phagocytic activity (Figs. S5 and S6). Thus JHD mice exhibit a temporal delay in macrophage influx and activity that can account for their delayed myelin clearance.

B-cell deficient mice have delayed macrophage influx following sciatic nerve injury that is rescued by passive antibody transfer. (A) Cryosections of uninjured and injured sciatic nerves from WT and JHD mice following passive Ig transfer immunostained with the combined macrophage-specific markers CD68 and F4/80 antibodies (shown in red). DAPI was used as nuclear counterstain (blue). (B) Time course of macrophage influx into the nerve after crush. (C) Quantification of macrophage numbers in JHD mice at 6 d postcrush (DPC) following 1 dose of passive transfer of Ig at 2 DPC. (D) Macrophage influx index in JHD nerves. Rate of macrophage influx in JHD nerves between postcrush day 2 and day 6 normalized to WT nerves (as measured from slope of the line between days 2 and 6 in B) in mice following 1 dose of passive transfer of Ig at 2 DPC. All data are from male mice and are presented as mean ± SEM, n = 7 animals per genotype per time point (*P < 0.05; **P < 0.01). (Scale bar, 200 μm.)
Next, we asked whether passive transfer of antibodies that rescue the delay in myelin clearance in injured JHD nerves is also sufficient to rescue the delay in macrophage influx in these mice. Passive transfer of IgM purified from naïve mice and monoclonal anti-P0 antibody rescues the delay of macrophage influx (Fig. 3 C and D). Passive transfer of anti-GFP antibody, however, was unable to increase macrophage numbers within injured JHD nerves (Fig. 3 C and D). This indicates that the delay in macrophage influx is likely explained by the absence of specific anti-myelin antibodies (Fig. S1), which may activate the complement cascade and thereby generate anaphylatoxins such as C3a or C5a that are strongly chemotactic for macrophages (14, 15). In addition, C3b fragments opsonize myelin debris, activating C3 receptors on macrophages that promote phagocytosis synergistically with Ig-mediated activation of Fc receptors on macrophages (16, 17).
Delayed Myelin Clearance Results in Diminished Axon Regeneration in JHD Mice.
Finally, the extent to which degenerating PNS myelin is inhibitory to regenerating axons has been controversial. To determine whether the absence of mature B-cells delays axon regeneration, we crossed the JHD mice to YFP-H mice in which 3% of myelinated axons in sciatic nerve are YFP positive (18, 19). These mice enable us to examine the regenerative rates of a specific subset of myelinated axons with high spatial resolution. We counted the number of YFP positive regenerating axons at 8 and 22 d following injury in JHD mice and littermate controls. Eight days after injury, the sciatic nerves of JHD mice contained 50% fewer YFP positive axons at 16 mm from the crush site than those of their WT littermates (Fig. 4 A–C). At 22 d following crush, the nerves of JHD mice still contained 9% fewer axons than WT nerves. To examine whether the absence of mature B-cells results in delays in axon regeneration across the entire population of axons in the sciatic nerve, we recorded evoked compound action potentials (CAP) from WT and JHD sciatic nerves at various times following sciatic nerve crush (Fig. 4D). CAP size is an approximate physiological measure of the number of axons present in the sciatic nerve. JHD mice exhibit significantly delayed regeneration of axons at 16 and 22 d after injury (Fig. 4E), with an approximate 50% decrease in CAP magnitude. In contrast, the velocity of axon conduction is not impaired, indicating that persisting myelin debris does not impair remyelination of axons that regenerate (Fig. 4F). The timing of the axon regeneration delay in the JHD mice closely parallels the delay in myelin clearance, consistent with the causal relationship that would be expected if the persisting myelin debris is inhibitory to axon regrowth.
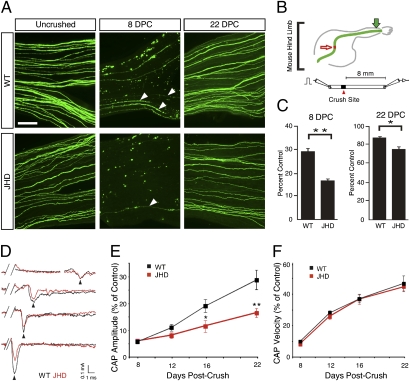
B-cell–deficient mice exhibit impaired axon regeneration after sciatic nerve injury. (A) Whole mount sciatic nerves from YFP positive JHD−/− and JHD+/− littermate control mice from uncrushed, 8, and 22 d postcrush (DPC). Arrowheads indicate regenerating axons. (B) Schematic of YFP positive sciatic nerve in mouse hind limb and electrophysiological recording. Red arrow indicates crush site. Green arrow indicates imaging site. A bipolar stimulating electrode was placed on the proximal end of the nerve and the recording electrode was placed on the distal end. (C) Ratio of the number of regenerating YFP positive axons to the number of uncrushed YFP positive nerves at 8 and 22 DPC (presented as mean ± SEM, n = 8–11 animals per genotype per time point). (D) Example traces of CAP recordings from WT (black) and JHD (red) nerves 8, 12, and 22 DPC. Note that the peak amplitude occurs at approximately the same time, but the CAPs from JHD nerves have smaller areas under the curve at 16 and 22 DPC. (E) Average CAP areas under the curve recorded from sciatic nerves of WT and JHD mice 8, 12, 16, and 22 DPC. The values are normalized to CAP area of uncrushed nerves (0.68 ± 0.06 mÅ*ms, n = 46). The CAP areas are statistically different between WT and JHD at 16 and 22 DPC. (F) CAP velocities recorded from sciatic nerves of WT and JHD mice 8, 12, 16, and 22 DPC. The values are normalized to the CAP velocity of uncrushed nerves (11.6 ± 0.4 m/s, n = 46). All data are from male mice and are presented as mean ± SEM. n = 4–18 animals per genotype per time point (*P < 0.05; **P < 0.01). (Scale bar, 200 μm.)
Discussion
Natural Antibodies Accumulate in Injury Site and Promote Repair.
In summary, our results show that after traumatic peripheral nerve injury, endogenous autoantibodies directed against degenerating PNS myelin are necessary for robust and rapid clearance of inhibitory myelin debris, thereby facilitating axon regeneration in the PNS. These findings have important implications. First, they provide evidence for two mechanistically distinct processes to clear myelin debris rapidly in the PNS: an antibody-independent process mediated by Schwann cells and an antibody-dependent process mediated by hematogenous macrophages. The development of parallel and distinct processes to clear myelin highlights the importance of myelin removal for the functional recovery of the organism. Second, these findings demonstrate that the humoral immune system, via the action of endogenous antibodies, can actively promote wound healing in the absence of an overtly immunogenic stimulus, such as vaccination, by recognizing degenerating tissue as nonself. Interestingly, several weeks after spinal cord injury B-cell numbers increase in spleen and bone marrow suggesting that failure to clear CNS debris may trigger pathological B-cell activation (20, 21). These data suggest the hypothesis that B-cell mediated autoimmune disease in the nervous system may in some cases arise from aberrations in a normally beneficial process—the clearance of myelin debris by endogenous antibodies. Our findings also raise the question of whether antibodies similarly promote healing in other organ systems.
Finally, these findings have important implications for understanding why degenerating CNS myelin is not cleared after injury and how its clearance might be induced. The blood-nerve barrier is rapidly broken down along the entire length of axotomized PNS but not CNS pathways (22, 23). Because Schwann cells are not found in the CNS and the blood–brain barrier prevents the entry of anti-CNS myelin antibodies into the distal white matter tracks, the injured CNS lacks the critical repair mechanisms available to the PNS. Our data therefore suggest the possibility that antibodies directed against CNS myelin, such as exogenously delivered anti-NogoA antibodies (7, 24, 25) or antibodies induced by CNS myelin immunization (26), promote CNS axon regeneration not only by neutralizing myelin inhibitors but, perhaps more importantly, by promoting myelin clearance. Indeed, opsonization by anti-NogoA antibodies could explain the greater apparent ability of the delivery of anti-NogoA antibodies to mediate regeneration than the genetic removal of NogoA (25). If so, promoting rapid myelin clearance in the CNS by delivering antibodies that specifically recognize degenerating CNS myelin may help to promote axon regeneration in patients following CNS injury.
Materials and Methods
Mice.
B-cell–deficient (JHD) mice were obtained from Taconic Animal Models. BALB/c mice were obtained form Charles River. YFP-H mice were obtained from Jackson Laboratories and backcrossed to JHD (BALB/c mice). The resulting F1 mice were intercrossed to generate mice for axon regeneration experiments. JHD mice were backcrossed to C57BL/6 mice for six to seven generations. All mice were used at 5–18 wk of age. Animals were housed and handled in accordance with the guidelines of the Administrative Panel on Laboratory Animal Care of Stanford University. All measurements in this study are presented as means ± SEM. Significance was determined with two-tailed unpaired Student's t test, and P < 0.05 was considered significant.
Reagents and Antibodies.
Anti-IgG (H+L) and IgM (μ chain) antibodies were purchased from Invitrogen. Anti-albumin-HRP (AHP 102P), anti-mouse CD68 (MCA1957), and anti-F4/80 (MCA497) antibodies were purchased from Serotec. Anti-mouse IgM-HRP (μ chain) and anti-mouse IgG-HRP (γ chain) were purchased from Jackson ImmunoResearch Labs. Anti-P0 antibody was obtained from Juan J. Archelos (University of Gratz, Austria). Anti-MBP (MAB-386) antibody was purchased from Chemicon. Anti-GFP (ab38689) and anti-firefly luciferase antibodies were purchased from Abcam.
Bone Marrow Transplantation.
WT and JHD mice were exposed to a split dose of 950 rads for lethal irradiation as previously described (27). WT bone marrow cells (6 × 106) were injected via the retro orbital sinus. Irradiated mice transplanted with WT bone marrow cells were housed in autoclaved cages and treated with antibiotics (0.2 mg/mL trimethoprim and 1 mg/mL sulfamethoxazole in drinking water given for 2 wk after irradiation).
Sciatic Nerve Crush.
Mice were anesthetized by i.p. injection of ketamine/xylazine (ketamine 100 mg/kg, xylazine 20 mg/kg) and given prophylactic antibiotics after surgery (0.2 mg/mL trimethoprim and 1 mg/mL sulfamethoxazole in drinking water). Upper thigh was shaved and sterilized using isopropanol. A 2-cm incision was made using a scalpel and nerve was visualized via blunt dissection using forceps. The left sciatic nerve was crushed at midthigh for 15 s using forceps marked with sterile graphite to mark the crush site.
Ig Purification.
IgM was purified using the Mannan Binding Protein column (Pierce Biotechnology) and IgG was purified using the Protein A column (GE Healthcare) from serum. Serum was diluted in binding buffer, filtered using 22-μm filter and loaded onto the column. The eluate containing Ig was concentrated using a cutoff spin filter (100 kDa for IgM, 10 kDa for IgG). The concentrate was diluted 20-fold in endotoxin-free PBS and filtered again.
Passive Antibody Transfer.
Antibody was passively transferred to mice via i.p. injections of 400 μL total volume of serum or 120 μg of total protein (purified Ig or monoclonal antibody) in 400 μL of endotoxin-free PBS at 2 and 6 d after crush surgery. Monoclonal antibodies were desalted with endotoxin-free PBS before injection. Mouse monoclonal anti-P0, anti-GFP, and anti-firefly luciferase antibodies used are IgG1 isotype.
Immunohistochemistry.
Sciatic nerves were harvested by perfusing mice with PBS for 10 min and then with 4% paraformaldehyde for 14 min. The nerves were postfixed for 1 h at 4 °C and sunk in 20% sucrose. Frozen sciatic nerves were crysosectioned into 8- to 10-μm sections and stained with the relevant antibody.
Western Blot Analysis.
Sciatic nerves were harvested and homogenized in ice cold RIPA buffer and stored at −80 °C. Lysates were centrifuged at 13,200 rpm for 15 min at 4 °C, and the protein concentration of the supernatant was determined by BCA assay (Pierce). Equal amounts of total protein were resolved by SDS–PAGE and transferred onto PVDF membranes. Blots were probed with relevant antibody and proteins were detected using chemiluminescence (GE Healthcare). Semiquantitative analysis was performed using National Institutes of Health ImageJ.
Electrophysiology.
The sciatic nerve was dissected out in PBS. Once the nerve was removed, it was placed in ACSF [(in mM) 125 NaCl, 2.5 KCl, 25 glucose, 25 NaHCO3, 1.25 NaH2PO4, 2 CaCl2, and 1 MgCl2, pH 7.2 (NaOH) which was saturated with 95% O2/5% CO2] until it was moved into the recording chamber. The sciatic nerve was perfused with ACSF during recording. The sciatic nerve was stimulated on the proximal end of the crush using a bipolar stimulating electrode (1-8 mA, 100 μs). The CAP was recorded from the distal end (approximately 9 mm distal to the site of crush) using a thick-walled glass electrode.
Acknowledgments
We thank Tom R. Clandinin and Richard Reimer for helpful comments and advice and Charles K. Chan and Denise B. Castillo for technical assistance. This work was supported by National Institutes of Health Grant EY11310 (to B.A.B.), Adelson Medical Research Foundation Grant (to B.A.B.), National Institute of General Medical Sciences Medical Scientist Training Program Grant 2T32GM07365 (to M.E.V.), Developmental and Neonatal Biology Training Program Grant 2 T32 HD007249 (to M.E.V.) from the National Institutes of Health, and National Multiple Sclerosis Society Postdoctoral Fellowship FG 1723-A-1 (to J.W.).
Footnotes
The authors declare no conflict of interest.
This article contains supporting information online at www.pnas.org/lookup/suppl/10.1073/pnas.1001948107/-/DCSupplemental.
References
Articles from Proceedings of the National Academy of Sciences of the United States of America are provided here courtesy of National Academy of Sciences
Full text links
Read article at publisher's site: https://doi.org/10.1073/pnas.1001948107
Read article for free, from open access legal sources, via Unpaywall:
https://europepmc.org/articles/pmc2900702?pdf=render
Free after 6 months at www.pnas.org
http://www.pnas.org/cgi/reprint/107/26/11993.pdf
Free after 6 months at www.pnas.org
http://www.pnas.org/cgi/content/abstract/107/26/11993
Free after 6 months at www.pnas.org
http://www.pnas.org/cgi/content/full/107/26/11993
Citations & impact
Impact metrics
Citations of article over time
Alternative metrics
Smart citations by scite.ai
Explore citation contexts and check if this article has been
supported or disputed.
https://scite.ai/reports/10.1073/pnas.1001948107
Article citations
BAFF neutralization impairs the autoantibody-mediated clearance of dead adipocytes and aggravates obesity-induced insulin resistance.
Front Immunol, 15:1436900, 09 Aug 2024
Cited by: 0 articles | PMID: 39185417 | PMCID: PMC11341376
Neither injury induced macrophages within the nerve, nor the environment created by Wallerian degeneration is necessary for enhanced in vivo axon regeneration after peripheral nerve injury.
J Neuroinflammation, 21(1):134, 27 May 2024
Cited by: 0 articles | PMID: 38802868 | PMCID: PMC11131297
Ropivacaine Promotes Axon Regeneration by Regulating Nav1.8-mediated Macrophage Signaling after Sciatic Nerve Injury in Rats.
Anesthesiology, 139(6):782-800, 01 Dec 2023
Cited by: 1 article | PMID: 37669448 | PMCID: PMC10723771
Inhibition of HDAC6 promotes microvascular endothelial cells to phagocytize myelin debris and reduces inflammatory response to accelerate the repair of spinal cord injury.
CNS Neurosci Ther, 30(3):e14439, 29 Aug 2023
Cited by: 1 article | PMID: 37641882 | PMCID: PMC10916453
Unresolved Excess Accumulation of Myelin-Derived Cholesterol Contributes to Scar Formation after Spinal Cord Injury.
Research (Wash D C), 6:0135, 04 May 2023
Cited by: 4 articles | PMID: 37223476 | PMCID: PMC10202378
Go to all (98) article citations
Data
Data behind the article
This data has been text mined from the article, or deposited into data resources.
BioStudies: supplemental material and supporting data
Similar Articles
To arrive at the top five similar articles we use a word-weighted algorithm to compare words from the Title and Abstract of each citation.
Passive immunization with anti-ganglioside antibodies directly inhibits axon regeneration in an animal model.
J Neurosci, 27(1):27-34, 01 Jan 2007
Cited by: 62 articles | PMID: 17202469 | PMCID: PMC6672271
Role of IL-10 in Resolution of Inflammation and Functional Recovery after Peripheral Nerve Injury.
J Neurosci, 35(50):16431-16442, 01 Dec 2015
Cited by: 75 articles | PMID: 26674868 | PMCID: PMC6605511
Differential macrophage responses in the peripheral and central nervous system during wallerian degeneration of axons.
Exp Neurol, 136(2):183-198, 01 Dec 1995
Cited by: 109 articles | PMID: 7498408
White matter inhibitors in CNS axon regeneration failure.
Exp Neurol, 209(2):302-312, 17 Jul 2007
Cited by: 58 articles | PMID: 17706966 | PMCID: PMC2259386
Review Free full text in Europe PMC
Funding
Funders who supported this work.
NEI NIH HHS (2)
Grant ID: R01 EY011310
Grant ID: EY11310
NICHD NIH HHS (2)
Grant ID: T32 HD007249
Grant ID: 2 T32 HD007249
NIGMS NIH HHS (2)
Grant ID: 2T32GM07365
Grant ID: T32 GM007365
PHS HHS (1)
Grant ID: FG 1723-A-1