Abstract
Free full text

Interaction of retinitis pigmentosa GTPase regulator (RPGR) with RAB8A GTPase: implications for cilia dysfunction and photoreceptor degeneration
Abstract
Defects in biogenesis or function(s) of primary cilia are associated with numerous inherited disorders (called ciliopathies) that may include retinal degeneration phenotype. The cilia-expressed gene RPGR (retinitis pigmentosa GTPase regulator) is mutated in patients with X-linked retinitis pigmentosa (XLRP) and encodes multiple protein isoforms with a common N-terminal domain homologous to regulator of chromosome condensation 1 (RCC1), a guanine nucleotide exchange factor (GEF) for Ran GTPase. RPGR interacts with several ciliopathy proteins, such as RPGRIP1L and CEP290; however, its physiological role in cilia-associated functions has not been delineated. Here, we report that RPGR interacts with the small GTPase RAB8A, which participates in cilia biogenesis and maintenance. We show that RPGR primarily associates with the GDP-bound form of RAB8A and stimulates GDP/GTP nucleotide exchange. Disease-causing mutations in RPGR diminish its interaction with RAB8A and reduce the GEF activity. Depletion of RPGR in hTERT-RPE1 cells interferes with ciliary localization of RAB8A and results in shorter primary cilia. Our data suggest that RPGR modulates intracellular localization and function of RAB8A. We propose that perturbation of RPGR–RAB8A interaction, at least in part, underlies the pathogenesis of photoreceptor degeneration in XLRP caused by RPGR mutations.
INTRODUCTION
Primary cilia are microtubule-based membranous extensions that carry out distinct and specialized functions including regulation of cellular homeostasis, signal transduction, cell polarity and protein trafficking (1–6). Cilia are generated from basal bodies that originate from the mother centriole (7–9) by a conserved mechanism called intraflagellar transport (IFT) (7,10,11). Owing to their involvement in diverse cellular processes, defects in cilia assembly or function may result in multisystemic and/or neonatally lethal disorders (also called ciliopathies), such as Bardet–Biedl syndrome (BBS), Joubert syndrome and Meckel–Gruber syndrome (12–14).
Syndromic ciliopathy phenotypes frequently include degeneration of retinal photoreceptors, probably because of the presence of a unique primary cilium that is modified to produce outer segment (OS) membrane discs for capturing light quanta (15–17). As ~10% of the photoreceptor discs are renewed daily (18–21), photoreceptors have high demands for the synthesis and transport of opsins and other phototransduction proteins from inner segments (the site of protein synthesis) to OS discs; any perturbation in cilia assembly or function can therefore lead to dysfunction or death of photoreceptors (22–26).
Retinitis pigmentosa (RP) is a clinically and genetically heterogeneous progressive neurodegenerative disease that involves retinal photoreceptors (27). X-linked forms of RP (XLRP) are among the most severe and account for 10–15% of inherited retinal degeneration (28–31). Though multiple genetic loci exist, mutations in the RPGR (RP GTPase regulator) gene account for >70% of XLRP and ~20% of RP cases with no family history (30,32). XLRP patients with RPGR mutations show extensive phenotypic variations, with some exhibiting even atrophic macular degeneration, respiratory tract infections and primary cilia dyskinesia (33–37). Multiple isoforms of RPGR are expressed in the retina (38,39). The two major isoforms are RPGR1–19 (exons 1–19) and RPGRORF15 (exons 1–15 + a part of intron 15 ORF15). Mutations in ORF15 isoform are found for 60–80% of XLRP (30,32,40).
RPGR is predominantly a cilia-centrosomal protein (41–43) that interacts with many ciliary proteins, such as RPGRIP1, IQCB1 (NPHP5), CEP290 (NPHP6) and RPGRIP1L (NPHP8) (22,44–46). Mutations in proteins that cause syndromic ciliopathies with retinal degeneration are shown to alter their interaction with RPGR (22,45). Though RPGR is suggested to regulate cilia function and facilitate the trafficking of proteins along the photoreceptor cilium (42,47–49), how its altered localization and/or function lead to photoreceptor degeneration has not been elucidated.
RPGR isoforms share a common N-terminal domain, called RLD (RCC1-like domain), which is homologous to regulator of chromosome condensation (RCC1) (50,51). RCC1 is a guanine nucleotide exchange factor (GEF) for Ran GTPase and regulates nucleocytoplasmic trafficking (52). It contains seven-bladed propeller repeats, a structural motif also found in p532, an exchange factor for RAB and ADP-ribosylation factor (ARF) proteins (53). The RLD of RPGR is shown to interact with delta subunit of rod cyclic cGMP phosphodiesterase in vitro (54), which in turn associates with ARF-like 3 GTPase (55). Based on these observations, RPGR is proposed to have a guanine nucleotide exchange function.
Recent studies have revealed an important role for the small GTPase RAB8A in cilia assembly and function (56). Moreover, RAB8A regulates the trafficking of rhodopsin in photoreceptors (57). We therefore hypothesized that RPGR functions as a GEF for RAB8A and RPGR–RAB8A association may facilitate ciliary trafficking. Here, we demonstrate a physiologically relevant interaction of RPGR with RAB8A and suggest a possible mechanism of photoreceptor degeneration associated with RPGR mutations.
RESULTS
RPGR interacts with RAB8A
To test whether RPGR associates with RAB8A in mammalian retina, we first performed co-immunoprecipitation (co-IP) experiments using bovine retinal extract. We observed that either anti-RAB8A antibody or anti-RPGR antibody could specifically pulldown RPGR isoforms or RAB8A, respectively, from the retinal extracts. We also detected higher RPGR–RAB8A association in the presence of GDP (Fig. 1A). No association was detected when normal immunoglobulin (IgG) or pre-immune serum were used for IP. We then used RPGR1–15, which spans the RLD, as a bait and RAB8A as a prey in yeast two-hybrid analysis and found that RPGR1–15 could directly interact with RAB8A (Fig. 1B). As a negative control, Laminin and T-antigen did not show an interaction in this assay. To further examine the physical interaction of RPGR and RAB8A in vitro, we used purified GST-RPGR1–15 (Fig. 1C) and 35S-labeled wild-type (WT) and mutant RAB8A proteins. RPGR preferentially associated with the GDP-locked form of RAB8A (T22N mutant) (Fig. 1D), and lesser interaction was detected with RAB8A-GTP (Q67L mutant) or WT RAB8A. No association was observed with GDP- or GTP-locked mutants of RAB11, another GTPase involved in intracellular trafficking (Fig. 1E). Using ciliated Madin Darby canine kidney (MDCK) cells, we found that endogenous RPGR and RAB8A co-localized at the primary cilia of epithelial cells (Fig. 1F).
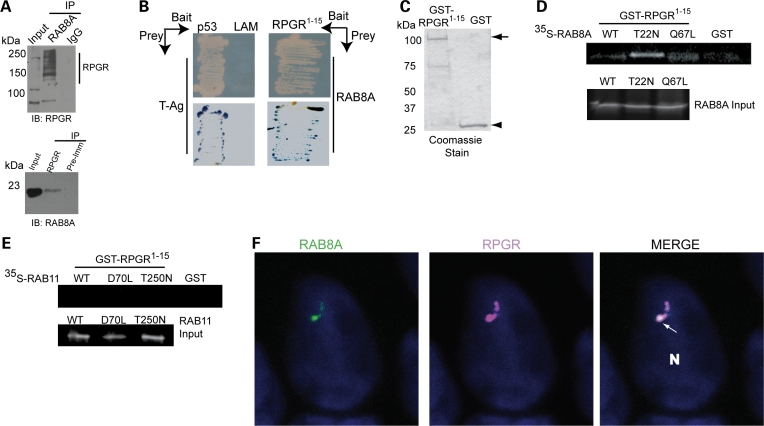
Interaction of RPGR with RAB8A. (A) Bovine retinal extract was prepared in the presence of the non-hydrolyzable GDP substrate and subjected to immunoprecipitation (IP) using anti-RAB8A, anti-RPGR antibodies or normal IgG and pre-immune (Pre-Imm) serum. Twenty percent of the protein used for IP was loaded in input lane. Vertical line represents different RPGR isoforms expressed in bovine retina, as described earlier (38,42). (B) A known-bait and known-prey yeast two-hybrid analysis. Upper panel represents the growth of yeast transformed with bait (RPGR1–15) and prey (RAB8A) constructs. Interaction between p53 (bait) and T antigen (T-Ag; prey) was used as a positive control, whereas laminin (LAM) and T-Ag association served as a negative control in this experiment. The lower panel represents β-galactosidase (blue) test of the growth assay shown in the upper panel. (C) Coomassie blue staining of the gel showing the purified WT GST-RPGR1–15 or GST proteins utilized in GST pulldown assay. Molecular mass markers are in kilo Daltons (kDa). (D and E) GST pulldown assay: 10 µg of purified GST-RPGR1–15 fusion protein or GST alone was incubated with 35S-labeled in vitro-translated RAB8A (C) or RAB11 (D) (or with indicated mutants). The proteins were collected by binding to Glutathione-Sepharose™ beads and analyzed by SDS–PAGE followed by autoradiography. Lanes are indicated. (F) Ciliated MDCK cells were stained with antibodies to RPGR (purple) and RAB8A (green). Immunostained cells were analyzed with OLYMPUS FV 500. White arrow in ‘Merge’ image shows co-localization of RPGR and RAB8A at the cilium. Nuclei (N) are stained with Hoechst (blue).
RPGR mutations alter its interaction with RAB8A
We then investigated the effect of disease-causing mutations in RPGR-RLD on its interaction with RAB8A. In a GST pulldown assay, the RPGR-H98Q mutant protein exhibited almost 5-fold less association with 35S-RAB8A-T22N compared with WT-RPGR. The G60V, G173R, T99N, F130C and G215V mutations in RPGR did not seem to affect the interaction with RAB8A (Fig. 2A and B).
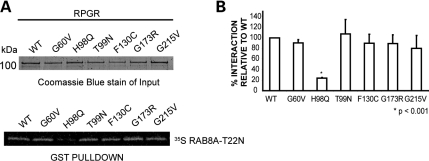
Interaction of RPGR mutants with RAB8A. (A) To evaluate the interaction of RPGR mutants with RAB8A-T22N, we expressed and purified GST-RPGR1–15 WT and mutant proteins. Upper panel represents the Coomassie blue-stained gel showing that equivalent amount of individual protein used in the GST pulldown assay. The proteins were incubated with in vitro-translated 35S RAB8A-T22N, followed by pulldown using Glutathione-Sepharose beads. 35S RAB8A-T22N-positive signal was detected by autoradiography with phosphorimager (Storm 840 GE Healthcare) (lower panel). (B) Quantification of GST pulldown was analyzed from Coomassie blue-stained gels and the autoradiograms, using ImageJ software. Area and intensity of RAB8A signal was measured and plotted against the amount of the respective mutant protein used in the pulldown assay. Graph represents each ratio normalized against the ratio of the binding of WT-RPGR with 35S RAB8A-T22N. Error bars represent mean ± SD from five independent experiments. The level of significance was calculated with two-tailed t-test. *P < 0.001.
RPGR can function as GEF for RAB8A
We then tested whether RPGR can potentiate the GTPase activity of RAB8A. Using a previously described fluorescence-based exchange assay (58,59), we measured the effect of RPGR on the degree of incorporation of fluorescent GTP into RAB8A. As a control, we utilized Rabin 8, which is an established GEF for RAB8A (60). Consistent with previous observations, the intrinsic activity of RAB8A (as observed by the amount of bound fluorescent analog of GTP [N-methylanthraniloyl-GTP (MANT-GTP)] was low (Fig. 3A); however, a 5-fold increase was observed in bound GTP in the presence of purified RPGR (Fig. 3A; Table 1), as was the case when RPGR was replaced by Rabin 8. In another set of control experiments, RPGR did not show a significant GEF activity for CDC42 GTPase, whereas DBS (an established GEF for CDC42) exhibited ~22-fold exchange activity (Table 2).
Table 1.
Activity of RPGR and Rabin8 over RAB8A
RAB8A intrinsic | RAB8A + Rabin8 | RAB8A + RPGR | |
---|---|---|---|
Exchange activity | 0.66 | 4.41 | 3.51 |
Fold change | 6.64 | 5.31 |
Relative fluorescence recording of incorporated MANT-GTP (λex = 360 nm and λem = 440 nm) onto His-RAB8A, when incubated with RPGR or RABIN8. Intrinsic rate was calculated as the MANT-GTP incorporation into RAB8A without the incubation of the GEF. Fold change is calculated as the ratio of exchange rate for each GEF over the intrinsic activity of RAB8A as a function of time.
Table 2.
Activity of RPGR and DBS over CDC42
CDC42 intrinsic | CDC42 + RPGR | CDC42 + DBS | |
---|---|---|---|
Exchange activity | 149.62 | 192.757 | 2580 |
Fold change | 0.8 | 22.5 |
Relative fluorescence recording of incorporated MANT-GTP (λex = 360 nm and λem = 440 nm) onto CDC42 incubated with RPGR or hDBS, a Dbl-family GEF for CDC42. Fold change is calculated as the ratio of exchange rate for each GEF over the intrinsic activity of RAB8A as a function of time.
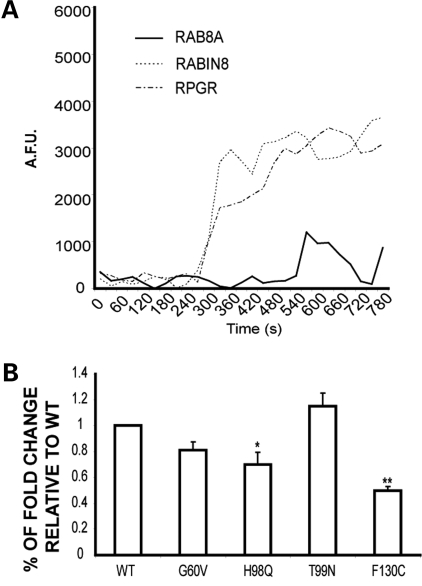
Modulation of RAB8A activity by RPGR. (A) Comparison of MANT-GTP incorporation into His-RAB8A as a function of time when incubated with RPGR or Rabin8. The activity of Rabin8 over RAB8A was used as positive control. AFU, arbitrary fluorescent units. (B) Fold change of RAB8A GDP/GTP exchange for each of the RPGR mutants relative to WT. Error bars represent mean ± SD from three independent experiments. Fold change was calculated as the ratio of RAB8A MANT-GTP incorporation after incubation with WT or mutant RPGR proteins with RAB8A MANT-GTP incorporation without incubation with RPGR (RAB8A intrinsic activity). Fold change by each mutant was compared with that depicted by WT RPGR from three independent experiments; level of significance was calculated with two-tailed t-test. *P < 0.05 and **P < 0.005.
Disease-causing mutations in RPGR affect its GEF function
Consistent with its diminished association with RAB8A, we found that RPGR-H98Q mutation exhibits ~30% decrease in the GEF activity for RAB8A compared with WT-RPGR (Fig. 3B). The RPGR-F130C mutant exhibited ~50% decrease in GEF activity though it did not display a significant decrease in its interaction with RAB8A (Fig. 2B). As RPGR-F130C mutation is reported to abolish its interaction with RPGRIP1 (61), our results indicate that, in photoreceptors, the conformation of RPGR or its interaction with RPGRIP1 may also modulate RPGR's RAB8A-GEF activity.
Knockdown of RPGR in hTERT-RPE1 cells results in shorter cilium and mislocalization of RAB8A
To further evaluate the role of RPGR in regulating RAB8A, we performed shRNA-mediated suppression of RPGR in hTERT-RPE1 cells. Transfection of RPGR-shRNA resulted in ~80% reduction of the endogenous RPGR protein compared with cells transfected with a scrambled shRNA construct (Fig. 4A). The RPGR isoforms at ~200 kDa were partially depleted, whereas 110 kDa isoform was undetectable in RPGR-shRNA-expressing cells. Interestingly, RPGR-shRNA-treated cells did have primary cilia, though in 65–70% of the cells the cilium was shorter (1.5–3.9 µm) (Fig. 4B) than in the control cells (expressing scrambled shRNA) (Fig. 4C and D). These observations are consistent with our recent study showing shorter cilia in Kupffer's vesicles upon morpholino-mediated knockdown of rpgr in zebrafish embryos (49).
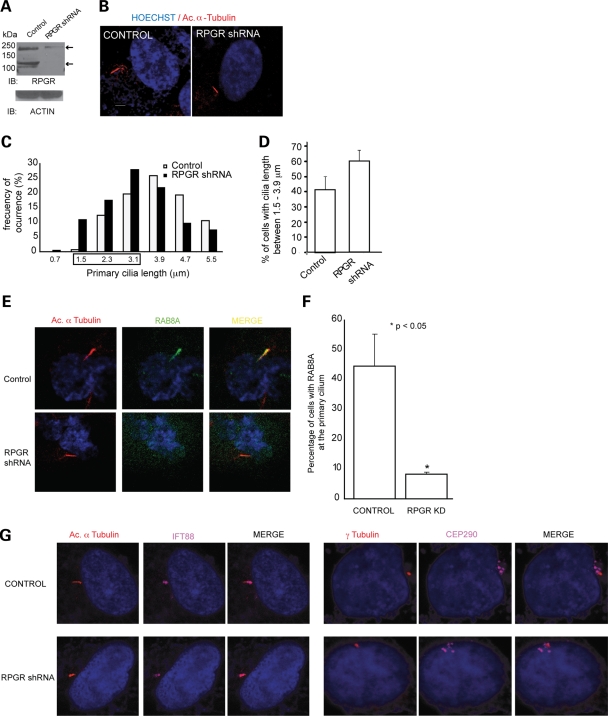
RPGR regulates ciliary localization of RAB8A. (A) Generation of RPGR-knockdown cells: lysates from stable transfected hTERT-RPE1 cells with control (scrambled) shRNA or RPGR shRNA were analyzed using anti-RPGR antibody or actin. Arrows represent distinct RPGR isoforms. (B) This panel represents cilia (red; acetylated α-tubulin) formation in control and RPGR-shRNA cells. Nuclei (blue) are stained with Hoechst. (C) Histograms show the distribution of cilia length in RPGR-shRNA-expressing cells. (D) There is increase in the number of cells with shorter cilia (1.5–3.9 µm) when treated with RPGR-shRNA compared with controls. Distribution of cilia length was calculated from three independent experiments with at least 150 cells analyzed in each experiment. Error bars represent standard deviation. (E) Knockdown of RPGR interferes with ciliary localization of RAB8A (green) to primary cilia (acetylated α-tubulin; red). Yellow in the Merge image indicates RAB8A localization at primary cilia. (F) Histogram showing decrease in number of cells with RAB8A at the cilium. We analyzed a total of 180 cells in each group and the experiment was repeated three times. (G) Knockdown of RPGR does not affect the localization of IFT88/Polaris (purple; left panel) to the cilium (red; Ac. α-tubulin; left panel) or of CEP290 (purple; right panel) to basal bodies (red; γ-tubulin; right panel).
As appropriate localization of RAB8A is required for cilia biogenesis and elongation, we examined whether depletion of RPGR might affect RAB8A localization. We observed that RAB8A is not localized at the cilium in 90% of RPGR-shRNA-treated cells compared with scrambled-shRNA-treated cells (Fig. 4 E and F) even though RAB8A protein levels were unaltered. RPGR-shRNA treatment did not significantly affect the localization of other RPGR-interacting ciliary and centrosomal proteins tested (CEP290, IFT88/Polaris, PCM1 and Pericentrin) (Fig. 4G; data not shown).
DISCUSSION
Ciliary dysfunction and disruption in trafficking of proteins are associated with early-onset severe retinal degeneration and blindness (62). RPGR is frequently involved in the manifestation of inherited severe photoreceptor degeneration. Although RPGR is primarily a ciliary protein and interacts with several ciliary and microtubule-based assemblies (41,42,45), its function and the mechanism of associated pathogenesis are still unclear. Here, we show that RPGR interacts with and regulates the activity of RAB8A, which is a critical GTPase involved in photoreceptor protein trafficking (57). Our results suggest that RPGR facilitates transport of proteins likely by promoting appropriate localization and activity of RAB8A.
RAB8A is involved in polarized trafficking of vesicles from the trans-Golgi network (63–67), and is the only RAB-family protein identified in mammalian cilia (68,69). Recent studies show that macromolecular complexes formed by selected BBS proteins (BBSome) regulate ciliogenesis by mediating the localization of Rabin 8 (56). Disruption of the BBSome compromises RAB8A function and cilia formation. As retinal degeneration is a principal feature of BBS, defects in RAB8A localization or activity may also affect photoreceptor protein trafficking. Given high trafficking demands of photoreceptors due to periodic OS disc shedding and renewal, activation of RAB8A may require RPGR GEF activity in addition to Rabin8. RPGR may therefore play a critical role in promoting RAB8A function in photoreceptors. Consistent with this hypothesis, mutations in RPGR that alter its association with RAB8A are predominantly associated with photoreceptor dysfunction and/or degeneration.
Several reports have demonstrated the involvement of extrinsic signals in dynamic regulation of cilia length in mammalian cells (70). Cilia extension has also been characterized in Chlamydomonas (71,72) and is believed to be associated with human disease (4,73). It is proposed that the IFT complexes, composed of microtubule-based motor assemblies and cargo proteins, are involved in regulating cilia extension. As RPGR associates with IFT88 and the Kinesin-II subunit KIF3A (42) and IFT88 interacts with opsin and other cargo in photoreceptors (74), an interesting hypothesis that remains to be tested is whether RPGR can facilitate protein interactions between cargo and IFT proteins during photoreceptor OS disc renewal. What might be the cargo for RPGR–RAB8A complex(es) in photoreceptors? As RAB8A regulates rhodopsin trafficking in photoreceptors, we tested but did not detect an association of RPGR with rhodopsin in bovine retinal protein complexes (data not shown). We cannot exclude the possibility of dynamic or transient nature of such interactions. It is also possible that opsin is not a cargo protein for RPGR-mediated ciliary transport.
The RPGR H98Q and F130C mutants exhibit compromised interaction with RPGRIP1 (61), suggesting that RPGR–RPGRIP1 interaction may be an important determinant of RPGR activity. As RPGRIP1 appears to link RPGR to the cilium (75), it is possible that RPGR's effect on RAB8A function may occur in distinct subcellular compartments of photoreceptors and that RPGRIP1 and other interacting proteins may modulate targeting of RPGR to such compartments. An indirect support for this hypothesis comes from CEP290, another RPGR-interacting protein (22) that also regulates cilia biogenesis and RAB8A localization (76). However, depletion of RPGR does not alter the localization of CEP290. Hence, there seems to be interplay between discrete protein complexes that function at distinct pathways in ciliary trafficking pathways, which culminate in docking and transport of proteins along the photoreceptor cilium. Identification of such dynamic protein complexes and their coordinated mechanism of action should contribute to better understanding of the dynamic nature of ciliary function in sensory neurons as well as pathomechanism of associated disorders.
MATERIALS AND METHODS
Antibodies and constructs
Generation and characterization of anti-RPGRORF15, CEP290 and anti-RAB8A antibodies have been described (22,42,60). The γ-tubulin and acetylated α-tubulin antibodies were purchased from Sigma, and pericentrin antibody from Abcam. PCR- based site-directed mutagenesis was performed using QuikChange kit from Stratagene.
Cell culture, transfection and microscopy
hTERT-RPE1 and MDCK cells were cultured according to American Type Culture Collection. Cilia growth and staining were performed as described (42). Cells were imaged on Olympus FV-500 or LEICA SP5 confocal microscope. To evaluate cilia length, hTERT-RPE1 cells stably expressing specific RPGR shRNA or scrambled shRNA (as control) were grown to confluence for 24 h followed by serum deprivation. Cells were then fixed with 4% PFA and cilia growth was analyzed by immunostaining with acetylated α-tubulin from (0.25 µm step size) z-stacks taken with LEICA SP6 confocal microscope. Cilia length was measured with LAS AF Lite software.
Protein–protein interaction
The protocols for yeast two-hybrid, GST pulldown and co-IP analyses have been described (42,45).
RAB8A activation assay
RhoGEF Exchange Assay Biochem kit (Cytoskeleton Inc., Denver, CO, USA) (58,59) was used according to manufacturer's instructions. The rate of guanine nucleotide exchange was recorded as the increase in N-methylanthraniloyl-GTP (MANT-GTP) fluorescence intensity incorporated into the GTPase. Then 0.5 µm RAB8A, 0.5 µm RPGR and 0.32 µm RABIN8 were incubated with the exchange buffer. Fluorescence intensity was recorded in Flex Station II Fluorometer (Molecular Devices, Sunnydale, CA, USA) at λex = 360 nm and λem = 440 nm, for 30 min at 27°C. RAB8A and Rabin 8 were purified from Escherichia coli, as described (60).
Knockdown of RPGR in cells
Plasmids encoding shRNA against human RPGR (exon 2) were purchased from OpenBiosystems (TGCTGTTGACAGTGAGCGACCGATTCGGGTGCTGTGTTTATAGTGAAG CCACAGATGTATAAACACAGCACCCGAATCGGGTGCCTACTGCCTCGGA). The shRNA plasmids were packaged into lentiviral particles and transfected into hTERT-RPE1 cells using standard protocols. Knockdown of RPGR expression was tested by immunoblotting.
FUNDING
This work is supported by NEI intramural funds and grants from the National Institutes of Health (RO1-EY007961), Midwest Eye Banks and Transplantation Center, Rare Disease Initiative at the University of Michigan and The Foundation Fighting Blindness. This work also utilized the services of Michigan Diabetes Research and Training Center (NIH5P60 DK20572) and Vision Core Facilities (EY07003). Funding to pay the Open Access Charge was provided by Midwest Eye Banks and Transplantation Center.
ACKNOWLEDGEMENTS
We thank Toby Hurd for valuable discussions.
Conflict of Interest statement. None declared.
REFERENCES
Articles from Human Molecular Genetics are provided here courtesy of Oxford University Press
Full text links
Read article at publisher's site: https://doi.org/10.1093/hmg/ddq275
Read article for free, from open access legal sources, via Unpaywall:
https://academic.oup.com/hmg/article-pdf/19/18/3591/8226408/ddq275.pdf
Free after 12 months at hmg.oxfordjournals.org
http://hmg.oxfordjournals.org/cgi/content/full/19/18/3591
Free to read at hmg.oxfordjournals.org
http://hmg.oxfordjournals.org/cgi/content/abstract/19/18/3591
Free after 12 months at hmg.oxfordjournals.org
http://hmg.oxfordjournals.org/cgi/reprint/19/18/3591.pdf
Citations & impact
Impact metrics
Citations of article over time
Alternative metrics
Article citations
Retinal Ciliopathies and Potential Gene Therapies: A Focus on Human iPSC-Derived Organoid Models.
Int J Mol Sci, 25(5):2887, 01 Mar 2024
Cited by: 1 article | PMID: 38474133 | PMCID: PMC10932180
Review Free full text in Europe PMC
Exome sequencing and genome-wide association analyses unveils the genetic predisposition in hydroxychloroquine retinopathy.
Eye (Lond), 38(10):1926-1932, 28 Mar 2024
Cited by: 0 articles | PMID: 38548946 | PMCID: PMC11226719
Retinitis pigmentosa GTPase regulator-related retinopathy and gene therapy.
Saudi J Ophthalmol, 37(4):276-286, 24 Oct 2023
Cited by: 2 articles | PMID: 38155670 | PMCID: PMC10752277
The New Era of Therapeutic Strategies for the Treatment of Retinitis Pigmentosa: A Narrative Review of Pathomolecular Mechanisms for the Development of Cell-Based Therapies.
Biomedicines, 11(10):2656, 28 Sep 2023
Cited by: 5 articles | PMID: 37893030 | PMCID: PMC10604477
Review Free full text in Europe PMC
Retinal degeneration in rpgra mutant zebrafish.
Front Cell Dev Biol, 11:1169941, 07 Jun 2023
Cited by: 0 articles | PMID: 37351277 | PMCID: PMC10282147
Go to all (71) article citations
Other citations
Data
Similar Articles
To arrive at the top five similar articles we use a word-weighted algorithm to compare words from the Title and Abstract of each citation.
Multiprotein complexes of Retinitis Pigmentosa GTPase regulator (RPGR), a ciliary protein mutated in X-linked Retinitis Pigmentosa (XLRP).
Adv Exp Med Biol, 664:105-114, 01 Jan 2010
Cited by: 20 articles | PMID: 20238008 | PMCID: PMC3464500
Review Free full text in Europe PMC
Prenylated retinal ciliopathy protein RPGR interacts with PDE6δ and regulates ciliary localization of Joubert syndrome-associated protein INPP5E.
Hum Mol Genet, 25(20):4533-4545, 01 Oct 2016
Cited by: 22 articles | PMID: 28172980 | PMCID: PMC6078598
Gelsolin dysfunction causes photoreceptor loss in induced pluripotent cell and animal retinitis pigmentosa models.
Nat Commun, 8(1):271, 16 Aug 2017
Cited by: 36 articles | PMID: 28814713 | PMCID: PMC5559447
Retinitis Pigmentosa GTPase Regulator (RPGR) protein isoforms in mammalian retina: insights into X-linked Retinitis Pigmentosa and associated ciliopathies.
Vision Res, 48(3):366-376, 27 Sep 2007
Cited by: 41 articles | PMID: 17904189 | PMCID: PMC2267686
Funding
Funders who supported this work.
Intramural NIH HHS
NEI NIH HHS (2)
Grant ID: R01-EY007961
Grant ID: EY07003
NIDDK NIH HHS (1)
Grant ID: 5P60 DK20572