Abstract
Free full text

Cyclin-dependent kinase 9–cyclin K functions in the replication stress response
Abstract
Cyclin-dependent kinase 9 (CDK9) is a well-characterized subunit of the positive transcription elongation factor b complex in which it regulates transcription elongation in cooperation with cyclin T. However, CDK9 also forms a complex with cyclin K, the function of which is less clear. Using a synthetic lethal RNA interference screen in human cells, we identified CDK9 as a component of the replication stress response. Loss of CDK9 activity causes an increase in spontaneous levels of DNA damage signalling in replicating cells and a decreased ability to recover from a transient replication arrest. This activity is restricted to CDK9–cyclin K complexes and is independent of CDK9–cyclin T complex. CDK9 accumulates on chromatin in response to replication stress and limits the amount of single-stranded DNA in cells under stress. Furthermore, we show that CDK9 and cyclin K interact with ataxia telangiectasia and Rad3-related protein and other checkpoint signalling proteins. These results reveal an unexpectedly direct role for CDK9–cyclin K in checkpoint pathways that maintain genome integrity in response to replication stress.
Introduction
Precise replication of the genome and continuous surveillance of its integrity are essential for cell survival and the avoidance of various diseases, including cancer. The replication stress response (RSR) helps cells to cope with environmental and endogenous genotoxic insults that challenge DNA replication. The RSR is crucial for the prevention of cancer, acting as a barrier against genomic instability and tumorigenesis (Bartkova et al, 2005; Gorgoulis et al, 2005; Bartek et al, 2007).
In eukaryotic cells, replication forks stall due to DNA lesions, depletion of nucleotide pools, complex chromatin organization and protein–DNA interactions. One component of RSR is ataxia telangiectasia and Rad3-related protein (ATR) checkpoint kinase (Cimprich & Cortez, 2008). ATR stabilizes stalled replication forks and promotes recovery. Furthermore, it senses stalled replication forks that result from fork uncoupling that exposes replication protein A (RPA)-bound single-stranded DNA (Byun et al, 2005). RPA contains a protein interaction domain that binds to several checkpoint proteins including ATR-interacting protein (ATRIP) and RAD9 homologue A to promote the assembly of checkpoint signalling complexes (Cortez et al, 2001; Zou & Elledge, 2003; Ball et al, 2007; Xu et al, 2008). This assembly promotes the interaction of TopBP1 with ATR–ATRIP complex, which activates ATR kinase activity (Kumagai et al, 2006). Once activated, ATR phosphorylates numerous substrates, including checkpoint kinase 1 (CHK1), which helps to disperse signals that are necessary to maintain genome integrity (Cimprich & Cortez, 2008).
CDK9 is a serine/threonine kinase. Unlike most CDKs—which function in regulating cell cycle transitions—CDK9 has been predominantly linked with transcription and also functions in co-transcriptional histone modification, messenger RNA (mRNA) processing, mRNA export and DNA repair (Bres et al, 2008; Romano & Giordano, 2008; Pirngruber et al, 2009; Liu et al, 2010). CDK9 forms a heterodimer with a regulatory cyclin: cyclins T1, T2a, T2b or K. The T-type cyclins interact with CDK9 to form the main component of the positive transcription elongation factor b (P-TEFb) complex that stimulates transcription elongation by phosphorylating the carboxy-terminal domain of the largest subunit of RNA polymerase II. Cyclin K can also interact with CDK9 in vitro and in vivo (Fu et al, 1999; Lin et al, 2002), and the CDK9–cyclin K complex can activate transcription when tethered to RNA, but not to DNA, in vitro (Lin et al, 2002); however, the function of cyclin K in vivo is not clear. The expression of cyclin K is activated by p53 in response to DNA damage (Mori et al, 2002), suggesting that it might function in the DNA damage response.
Results And Discussion
Hydroxyurea sensitivity screen identifies CDK9
To identify genes involved in mediating recovery after replication fork arrest, we completed an unbiased loss-of-function genetic screen in human cultured cells. We reasoned that genes involved in the RSR would probably be required to maintain cell viability when challenged with an agent that stalls replication forks. We therefore optimized a high-throughput assay using two RSR genes—ATR and ATRIP—as positive controls and a nontargeting oligonucleotide as a negative control. The assay measures the ability of cells to recover from a transient, high dose of hydroxyurea (HU), a drug that stalls replication forks by inhibiting ribonucleotide reductase. The primary screen was completed in U2OS human osteosarcoma cells using the Dharmacon druggable genome library of 27,252 small interfering RNAs (siRNAs) targeting 6,813 human genes. Cells were transfected in duplicate with pools of four siRNAs targeting each gene arrayed in a one-gene-per-well format in 96-well plates, split 1:4, treated with or without 3 mM HU for 24 h and then assayed for cell number using WST-1 reagent at 24 h after recovery from treatment (Fig 1A). Plate-to-plate variability was controlled by normalizing to the average of the nontargeting siRNA values. Candidate RSR genes were identified by examining the ratio of HU-treated to non-HU-treated cell numbers (Fig 1B) and by analysis of significance by the weighted flexible compound covariate method (supplementary Fig S1 online; Shyr & KyungMann, 2003). Validation of the primary screen was accomplished by testing four individual siRNAs to eliminate off-target effects (validated HU-sensitive genes are presented in supplementary Table S1 online). Two of the genes that met these criteria were HUS1 and RAD17 (Fig 1C), known ATR signalling pathway genes, which provided internal validation of the screen. In this study, we focus on CDK9. All four siRNAs targeting CDK9 cause HU hypersensitivity (Fig 1C,D).
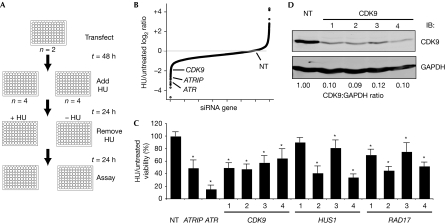
Silencing cyclin-dependent kinase 9 causes hydroxyurea hypersensitivity. (A) Diagram of primary screen assay as described in the text. (B) Summary of results of primary screen. The log2 ratio of treated compared with untreated cell viability relative to the nontargeting (NT) siRNA for each gene is shown. (C) Validation of CDK9, HUS1 and RAD17 after deconvolution of siRNA pools. Four siRNAs targeting each gene were tested as indicated. Treated compared with untreated percentage viability was calculated and the mean and s.d. values from three replica experiments are shown. Asterisk indicates P<0.05. (D) Western blot analysis demonstrating efficiency of knockdown with the indicated siRNAs. ATR, ataxia telangiectasia and Rad3-related protein; ATRIP, ATR-interacting protein; CDK9, cyclin-dependent kinase 9; HU, hydroxyurea; NT, nontargeting; siRNA, small interfering RNA.
CDK9 is a replication stress response protein
To validate that CDK9 is an RSR protein, we examined the ability of CDK9-silenced cells to recover from a transient replication fork arrest using cell cycle analysis by flow cytometry. U2OS cells treated with HU for 20 h were observed to be arrested in early S-phase (Fig 2A). At 10 h after removing HU, cells treated with nontargeting siRNA oligonucleotides progressed through S-phase and accumulated 4N DNA content, whereas U2OS cells treated with ATRIP, ATR or CDK9 siRNA oligonucleotides have a delayed progression through S-phase (Fig 2A,B). A similar impairment in recovery after CDK9 silencing was observed in human telomerase-immortalized epithelial cells, suggesting that the phenotype is not cell-type-specific (data not shown). Depletion of CDK9 caused a similar defect in recovery after a replication challenge of aphidicolin, a DNA polymerase inhibitor (Fig 2A,B). In the absence of exogenous damage, no changes in cell proliferation or apoptosis are seen after CDK9-silencing (supplementary Fig S2 online).
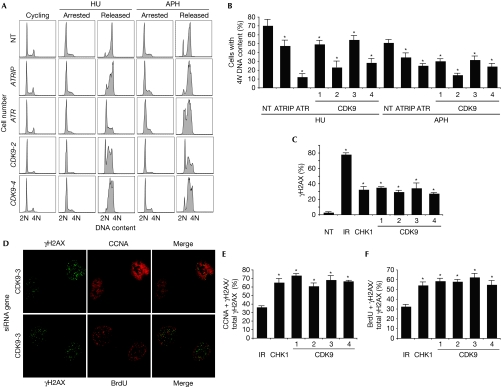
Cyclin-dependent kinase 9 is required for cells to complete DNA synthesis after replication stress. (A,B) U2OS cells were transfected with NT, ATR, ATRIP or CDK9 siRNA, treated with 3 mM HU or 15 μM APH for 20 h (arrested), and released into 1 μg/ml nocodazole for 10 h (released). DNA content was analysed by using flow cytometry. (B) The percentage (mean and s.d.) of cells that completed DNA synthesis in three replicate experiments is shown. (C) Depletion of CDK9 induces DNA damage signalling in replicating cells. U2OS cells were transfected with NT, CHK1 or CDK9 siRNA or treated with 5 Gy IR and processed 72 or 4 h later, respectively, for γH2AX staining by indirect immunofluorescence microscopy. The percentage of cells staining for γH2AX was scored. (D) U2OS cells were co-stained with γH2AX and CCNA or γH2AX and BrdU 72 h after transfection with siRNA. Quantitation of the percentage of cells co-staining with (E) γH2AX and CCNA or (F) γH2AX and BrdU 4 h after treatment with 5 Gy IR or 72 h after transfection with CHK1 or CDK9 siRNA. Mean and s.d. values from three replica experiments are shown. Asterisks in all panels indicate P<0.05. APH, aphidicolin; ATR, ataxia telangiectasia and Rad3-related protein; ATRIP, ATR-interacting protein; BrdU, 5-bromo-2-deoxyuridine; CCNA, cyclin A; CDK9, cyclin-dependent kinase 9; HU, hydroxyurea; IR, ionizing radiation; NT, nontargeting; siRNA, small interfering RNA.
Next, we examined the induction of DNA damage after CDK9 knockdown by immunofluorescence microscopy for phosphorylated histone γH2AX. Silencing many genes that function in RSR pathways causes an increase in spontaneous γH2AX staining during S-phase due to a failure to maintain replication fork stability, even in the absence of added genotoxic agents (Lovejoy et al, 2009; Paulsen et al, 2009). At 72 h after CDK9 silencing, phosphorylation of γH2AX was significantly increased compared with nontargeting silencing. To determine whether the induction of γH2AX occurs in replicating cells, we co-stained the cells for cyclin A—a cell marker in S- and G2-phase—and 5-bromo-2-deoxyuridine (BrdU)—a cell marker in S-phase. In contrast to cells treated with ionizing radiation—which causes damage in all phases of the cell cycle—cells in which CHK1 or CDK9 is silenced exhibit significantly increased co-staining for γH2AX and cyclin A (Fig 2D,E) and for γH2AX and BrdU (Fig 2D,F), suggesting that silencing of CDK9 induces DNA damage in replicating cells. Collectively, these findings demonstrate that CDK9 functions in an RSR pathway to maintain genome integrity during DNA replication.
CDK9 kinase activity is essential for its functions in the RSR
To assess whether the kinase activity of CDK9 is essential for mediating cell cycle recovery after replication stress, we generated U2OS cell lines stably expressing exogenous wild-type FLAG–HA (haemagglutinin)–CDK9 or FLAG–HA–CDK9 D167N—a kinase-dead mutant (Garriga et al, 1996)—and silenced endogenous CDK9 using siRNA targeting the 3′-untranslated region. Wild-type FLAG–HA–CDK9 but not FLAG–HA–CDK9 D167N complemented the HU and aphidicolin recovery deficits of CDK9-silenced cells (Fig 3A,B), suggesting that the kinase activity of CDK9 is essential for its functions in the RSR and confirming the siRNA phenotypes are not due to off-target effects. Western blot analysis demonstrated the expression of exogenous fusion proteins and knockdown of endogenous CDK9 in these experiments (Fig 3C).
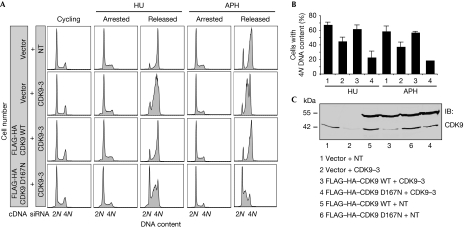
Cyclin-dependent kinase 9 activity is essential for its activities in the replication stress response. (A,B) U2OS cells stably expressing an empty vector, wild-type FLAG–HA–CDK9 or FLAG–HA–CDK9 D167N were transfected with NT or CDK9 siRNA directed against the 3′-UTR of CDK9, treated with 3 mM HU or 15 μM APH for 20 h and released into nocodazole for 10 h. DNA content was analysed by flow cytometry. (B) The percentage (mean and s.d.) of cells that completed DNA synthesis in three replicate experiments is shown. (C) Western blot analysis demonstrating expression of fusion proteins and knockdown of endogenous CDK9. APH, aphidicolin; CDK9, cyclin-dependent kinase 9; HA, haemagglutinin; HU, hydroxyurea; NT, nontargeting; siRNA, small interfering RNA; UTR, untranslated region.
Cyclin K is a replication stress response protein
To determine which regulatory subunit works with CDK9 in the RSR, we examined cell cycle recovery after a replication challenge of HU or aphidicolin in cells silenced for cyclins T1, T2 and K. Four siRNAs targeting cyclin K strongly impaired cell cycle recovery (Fig 4A,B). By contrast, silencing cyclin T1 and cyclin T2 did not cause a deficit in cell cycle recovery (Fig 4A,B; supplementary Fig S3 online), suggesting that cyclin K, but not cyclin T1 or cyclin T2, is the regulatory subunit of CDK9, which mediates its activities in the RSR. Western blot analysis of cells treated with siRNA against cyclin K, T1 and T2 confirmed the knockdown of protein expression (Fig 4C–E). Silencing of cyclin K in the absence of exogenous damage also induced a significant increase in phosphorylation of γH2AX compared with nontargeting silencing (Fig 4F), consistent with a role for cyclin K in maintaining genome integrity.
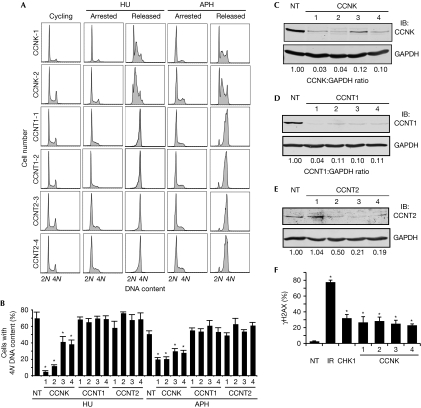
The cyclin-dependent kinase 9 regulatory subunit cyclin K, but not cyclin T1 or cyclin T2, is required for cell cycle recovery after replication arrest. (A,B) U2OS cells were transfected with NT, cyclin K, cyclin T1 or cyclin T2 siRNA, treated with 3 mM HU or 15 μM APH for 20 h and released into nocodazole for 10 h. DNA content was analysed by using flow cytometry. (B) The percentage (mean and s.d.) of cells that completed DNA synthesis in three replicate experiments is shown. (C–E) Western blot analysis demonstrating efficiency of knockdown with four unique siRNAs. (F) Depletion of cyclin K induces DNA damage signalling. U2OS cells were transfected with NT, CHK1 or cyclin K siRNA or treated with 5 Gy IR and processed 72 or 4 h later, respectively, for γH2AX by indirect immunofluorescence microscopy. Mean and s.d. values from three replicate experiments is shown. Asterisks indicate P<0.05. APH, aphidicolin; CDK9, cyclin-dependent kinase 9; HU, hydroxyurea; IB, immunoblotted; IR, ionizing radiation; NT, nontargeting; siRNA, small interfering RNA.
The absence of a deficit in cell cycle recovery after silencing of cyclin T1 and cyclin T2—well-characterized regulatory subunits of the P-TEFb complex—suggests that the RSR phenotypes observed after CDK9 knockdown might be independent of P-TEFb-mediated transcription regulation. To further examine this issue, we performed a microarray genome-wide expression analysis in U2OS cells treated with nontargeting or CDK9 siRNA under the same conditions in which we obtained our loss-of-function phenotypes. The microarray results are summarized in supplementary Table S2 online. The expression levels of 60 genes without treatment with HU and 127 genes treated with HU were significantly downregulated by at least 1.5-fold, and the expression levels of 75 genes without treatment with HU and 138 genes treated with HU were significantly upregulated after CDK9 silencing, compared with nontargeting silencing. However, few of the regulated genes have any functional link to the cell cycle or DNA damage, suggesting that transcriptional changes might not account for RSR defects in CDK9-silenced cells.
CDK9–cyclin K complex interacts with other RSR proteins
To test whether CDK9 functions directly in the ATR-dependent RSR pathway, we tested whether CDK9 could form complexes with ATR or other RSR proteins. Immunoprecipitation of CDK9–HA expressed in HEK293T cells pulled down endogenous ATR, Claspin and ATRIP, but not PCNA or RPA32 (Fig 5A). Endogenous CDK9 also co-immunoprecipitated with endogenous ATR, Claspin and ATRIP (Fig 5B,C), suggesting that the proteins interact in a complex or complexes. These interactions are not DNA-dependent or regulated by replication stress as they are preserved after treatment of the immunoprecipitation reactions with benzonase nuclease and are unchanged after treatment of the cells with HU (data not shown). We further investigated whether any of the regulatory cyclins interacted with components of the RSR. Immunoprecipitation of ATR pulled down both CDK9 and cyclin K but not cyclin T1 or cyclin T2 (Fig 5C,D). These data further confirm that cyclin K, not cyclin T, mediates the RSR functions of CDK9.
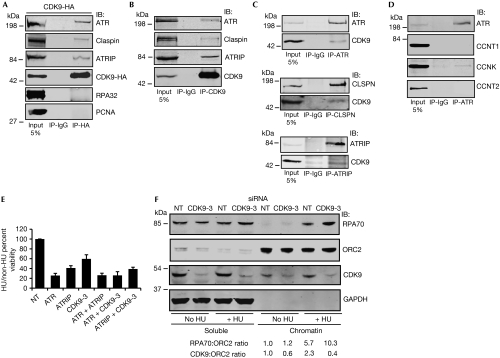
Cyclin-dependent kinase 9 interacts with components of the replication stress response, localizes to chromatin and reduces chromatin-bound RPA after replication stress. (A) Cells were transfected with CDK9–HA, harvested to prepare nuclear extracts and immunoprecipitated with HA or control IgG antibodies. Bound proteins were washed, separated by SDS–PAGE and immunoblotted with antibodies against ATR, Claspin, ATRIP, HA, RPA32, and PCNA. (B–D) Endogenous CDK9, ATR, Claspin or ATRIP were immunoprecipitated from cell lysates as indicated. Immunocomplexes were washed, separated by SDS–PAGE and immunoblotted with antibodies against the indicated proteins. (E) U2OS cells were transfected with the indicated siRNAs and tested for sensitivity to HU. Mean and s.d. values from three replicate experiments is shown. (F) Soluble and chromatin fraction of U2OS cells transfected with NT or CDK9-3 siRNA for 72 h and treated with or without 3 mM HU for 20 h. Chromatin-bound RPA70/ORC2 and CDK9/ORC2 ratio of representative blot from three independent experiments is shown. APH, aphidicolin; ATR, ataxia telangiectasia and Rad3-related protein; ATRIP, ATR-interacting protein; CDK9, cyclin-dependent kinase 9; HA, haemagglutinin; HU, hydroxyurea; IB, immunoblotted; IR, ionizing radiation; ORC2, origin recognition complex 2; PCNA, proliferating cell nuclear antigen; RPA, replication protein A; SDS–PAGE, sodium dodecyl sulphate–polyacrylamide gel electrophoresis; siRNA, small interfering RNA.
To determine whether CDK9 and ATR function in a common pathway, we performed epistasis experiments for HU sensitivity after silencing of ATR, ATRIP and CDK9. The silencing of ATR and ATRIP showed a similar HU sensitivity compared with ATR silencing alone, consistent with ATR and ATRIP functioning in the same pathway (Fig 5E). Similarly, ATR silencing and CDK9 silencing also caused a similar HU sensitivity compared with ATR silencing alone, suggesting that ATR and CDK9 act in a common pathway that sensitizes to HU (Fig 5E).
To investigate the function of CDK9 in the ATR signalling pathway, we examined the requirement of CDK9 for ATR-dependent signalling in response to replication stress. Silencing of CDK9 did not impair CHK1 or minichromosome maintenance 2 phosphorylation in response to HU treatment, suggesting that CDK9 does not regulate ATR activation (supplementary Fig S4A online). Furthermore, CHK1 autophosphorylation is not impaired in CDK9-silenced cells (supplementary Fig S4B online). We did observe an increase in CDC25A protein levels (with no change in mRNA levels, P=0.515) in CDK9-silenced cells both before and after treatment with HU, which was rescued by the siRNA-resistant CDK9 complementary DNA (supplementary Fig S4B online). These data suggest that CDK9 might function either downstream from CHK1 or perhaps in a parallel pathway to regulate CDC25A stability.
CDK9 reduces chromatin-bound RPA
To determine whether CDK9 is regulated in response to replication stress, we examined whether its activity or localization is changed in HU-treated cells. Although we observed no evidence that its kinase activity was altered, we did observe a 2.3-fold increase in the amount of CDK9 bound to chromatin after treatment with HU (Fig 5F). Furthermore, the amount of chromatin-bound RPA increased approximately twofold in CDK9-silenced cells after HU treatment (Fig 5F), suggesting that CDK9 acts to limit the amount of single-stranded DNA available for RPA binding. Together with the induction of γH2AX phosphorylation in CDK9-silenced, replicating cells, these findings are consistent with a model in which CDK9 responds to replication stress by localizing to chromatin to reduce the breakdown of stalled replication forks.
CDK9 is overexpressed in several cancers—including leukaemias and lymphomas—and has been suggested as a useful drug target (Bellan et al, 2004; Romano & Giordano, 2008). Our data indicate that targeting CDK9 would not only alter transcriptional regulation but also have an impact on replication stress responses. Indeed, U2OS cells treated with flavopiridol or 5,6-dichloro-1-β-D-ribofuranosylbenzimidazole—which inhibit CDK9 activity—impair cell cycle recovery in response to treatment with HU (supplementary Fig S5 online). Thus, CDK9 inhibitors might be useful in cancers involving high levels of replication stress, or in combination with replication stress-inducing chemotherapies.
Our data suggest a direct function for CDK9–cyclin K complexes in the RSR. The RSR function of CDK9 might be evolutionarily conserved; its Saccharomyces cerevisiae homologue—Sgv1—is synthetically lethal with the checkpoint proteins Mrc1, Csm3, Tof1 and Sgs1 (Collins et al, 2007), which help to stabilize DNA polymerase at stalled replication forks. Furthermore, a mutation in the T-loop of Schizosaccharomyces pombe CDK9 causes UV hypersensitivity (Gerber et al, 2008). These data provide evidence that the CDK9–cyclin K complex has different biological activities to the CDK9–cyclin T complex.
Methods
Cell lines, plasmids and siRNA. U2OS and HEK293T were maintained in Dulbecco's modified Eagle medium supplemented with 7.5% fetal bovine serum. Kinase-dead CDK9 D167N was generated by site-directed PCR mutagenesis. Stable cell lines expressing wild-type or mutant CDK9 were created by retroviral integration. The human druggable genome RNA-mediated interference library was obtained from Dharmacon. Individual siRNA sequences used for experiments are described in the supplementary information online. RSR assays were performed as previously described (Mordes et al, 2008) using 20-h treatments with either 3 mM HU or 15 μM aphidicolin. The primary screen was completed in duplicate with siRNA at a concentration of 25 nM using HiPerfect (Qiagen). Individual siRNAs in validation experiments were each transfected six times at a concentration of 10 nM. Drug was added 72 h after transfection for both primary and validation screens.
Immunofluorescence and immunoprecipitation. Indirect immunofluorescence for γH2AX, cyclin A and BrdU was performed as previously described (Bansbach et al, 2009). Cell lysates for overexpression, co-immunoprecipitation and western blot analyses were prepared with 0.5% NP-40 lysis buffer (50 mM Tris (pH 8.0) and 150 mM NaCl) supplemented with protease and phosphatase inhibitors. Nuclear extracts were used for the endogenous co-immunoprecipitation experiments as described previously (Mordes et al, 2008). Chromatin fractions were prepared as previously described (Bansbach et al, 2009). Antibodies are described in the supplementary information online.
Supplementary information is available at EMBO reports online (http://www.emboreports.org).
Acknowledgments
We thank D. Hallahan for useful discussions. This study was supported by National Cancer Institute grant R01CA136933 to D.C.; the Vanderbilt Center in Molecular Toxicology (P30ES000267), Vanderbilt-Ingram Cancer Center and Vanderbilt Institute for Clinical and Translational Research (UL1 024975); and a Department of Defense Breast Cancer Research Programme Era of Hope Postdoctoral Award BC085283 to D.S.Y., who is also a recipient of the American Board of Radiology Holman Research Pathway fellowship.
References
- Ball HL, Ehrhardt MR, Mordes DA, Glick GG, Chazin WJ, Cortez D (2007) Function of a conserved checkpoint recruitment domain in ATRIP proteins. Mol Cell Biol 27: 3367–3377 [Europe PMC free article] [Abstract] [Google Scholar]
- Bansbach CE, Betous R, Lovejoy CA, Glick GG, Cortez D (2009) The annealing helicase SMARCAL1 maintains genome integrity at stalled replication forks. Genes Dev 23: 2405–2414 [Europe PMC free article] [Abstract] [Google Scholar]
- Bartek J, Lukas J, Bartkova J (2007) DNA damage response as an anti-cancer barrier: damage threshold and the concept of ‘conditional haploinsufficiency'. Cell Cycle 6: 2344–2347 [Abstract] [Google Scholar]
- Bartkova J et al. (2005) DNA damage response as a candidate anti-cancer barrier in early human tumorigenesis. Nature 434: 864–870 [Abstract] [Google Scholar]
- Bellan C et al. (2004) CDK9/CYCLIN T1 expression during normal lymphoid differentiation and malignant transformation. J Pathol 203: 946–952 [Abstract] [Google Scholar]
- Bres V, Yoh SM, Jones KA (2008) The multi-tasking P-TEFb complex. Curr Opin Cell Biol 20: 334–340 [Europe PMC free article] [Abstract] [Google Scholar]
- Byun TS, Pacek M, Yee MC, Walter JC, Cimprich KA (2005) Functional uncoupling of MCM helicase and DNA polymerase activities activates the ATR-dependent checkpoint. Genes Dev 19: 1040–1052 [Europe PMC free article] [Abstract] [Google Scholar]
- Cimprich KA, Cortez D (2008) ATR: an essential regulator of genome integrity. Nat Rev Mol Cell Biol 9: 616–627 [Europe PMC free article] [Abstract] [Google Scholar]
- Collins SR et al. (2007) Functional dissection of protein complexes involved in yeast chromosome biology using a genetic interaction map. Nature 446: 806–810 [Abstract] [Google Scholar]
- Cortez D, Guntuku S, Qin J, Elledge SJ (2001) ATR and ATRIP: partners in checkpoint signaling. Science 294: 1713–1716 [Abstract] [Google Scholar]
- Fu TJ, Peng J, Lee G, Price DH, Flores O (1999) Cyclin K functions as a CDK9 regulatory subunit and participates in RNA polymerase II transcription. J Biol Chem 274: 34527–34530 [Abstract] [Google Scholar]
- Garriga J, Mayol X, Grana X (1996) The CDC2-related kinase PITALRE is the catalytic subunit of active multimeric protein complexes. Biochem J 319: 293–298 [Europe PMC free article] [Abstract] [Google Scholar]
- Gerber HB, Pikman Y, Fisher RP (2008) The CDK-activating kinase (CAK) Csk1 is required for normal levels of homologous recombination and resistance to DNA damage in fission yeast. PLoS ONE 3: e1492. [Europe PMC free article] [Abstract] [Google Scholar]
- Gorgoulis VG et al. (2005) Activation of the DNA damage checkpoint and genomic instability in human precancerous lesions. Nature 434: 907–913 [Abstract] [Google Scholar]
- Kumagai A, Lee J, Yoo HY, Dunphy WG (2006) TopBP1 activates the ATR–ATRIP complex. Cell 124: 943–955 [Abstract] [Google Scholar]
- Lin X, Taube R, Fujinaga K, Peterlin BM (2002) P-TEFb containing cyclin K and Cdk9 can activate transcription via RNA. J Biol Chem 277: 16873–16878 [Abstract] [Google Scholar]
- Liu H, Herrmann CH, Chiang K, Sung TL, Moon SH, Donehower LA, Rice AP (2010) 55K isoform of CDK9 associates with Ku70 and is involved in DNA repair. Biochem Biophys Res Commun 397: 245–250 [Europe PMC free article] [Abstract] [Google Scholar]
- Lovejoy CA, Xu X, Bansbach CE, Glick GG, Zhao R, Ye F, Sirbu BM, Titus LC, Shyr Y, Cortez D (2009) Functional genomic screens identify CINP as a genome maintenance protein. Proc Natl Acad Sci USA 106: 19304–19309 [Europe PMC free article] [Abstract] [Google Scholar]
- Mordes DA, Glick GG, Zhao R, Cortez D (2008) TopBP1 activates ATR through ATRIP and a PIKK regulatory domain. Genes Dev 22: 1478–1489 [Europe PMC free article] [Abstract] [Google Scholar]
- Mori T, Anazawa Y, Matsui K, Fukuda S, Nakamura Y, Arakawa H (2002) Cyclin K as a direct transcriptional target of the p53 tumor suppressor. Neoplasia 4: 268–274 [Europe PMC free article] [Abstract] [Google Scholar]
- Paulsen RD et al. (2009) A genome-wide siRNA screen reveals diverse cellular processes and pathways that mediate genome stability. Mol Cell 35: 228–239 [Europe PMC free article] [Abstract] [Google Scholar]
- Pirngruber J, Shchebet A, Schreiber L, Shema E, Minsky N, Chapman RD, Eick D, Aylon Y, Oren M, Johnsen SA (2009) CDK9 directs H2B monoubiquitination and controls replication-dependent histone mRNA 3′-end processing. EMBO Rep 10: 894–900 [Europe PMC free article] [Abstract] [Google Scholar]
- Romano G, Giordano A (2008) Role of the cyclin-dependent kinase 9-related pathway in mammalian gene expression and human diseases. Cell Cycle 7: 3664–3668 [Abstract] [Google Scholar]
- Shyr Y, KyungMann K (2003) Weighted flexible compound covariate method for classifying microarray data. In A Practical Approach to Microarray Data Analysis, Berrar D (ed), pp 186–201. Kluwer Academic Publishers: Norwell, MA [Google Scholar]
- Xu X, Vaithiyalingam S, Glick GG, Mordes DA, Chazin WJ, Cortez D (2008) The basic cleft of RPA70N binds multiple checkpoint proteins, including RAD9, to regulate ATR signaling. Mol Cell Biol 28: 7345–7353 [Europe PMC free article] [Abstract] [Google Scholar]
- Zou L, Elledge SJ (2003) Sensing DNA damage through ATRIP recognition of RPA–ssDNA complexes. Science 300: 1542–1548 [Abstract] [Google Scholar]
Articles from EMBO Reports are provided here courtesy of Nature Publishing Group
Full text links
Read article at publisher's site: https://doi.org/10.1038/embor.2010.153
Read article for free, from open access legal sources, via Unpaywall:
https://onlinelibrary.wiley.com/doi/pdfdirect/10.1038/embor.2010.153
Citations & impact
Impact metrics
Citations of article over time
Article citations
SAMHD1 expression contributes to doxorubicin resistance and predicts survival outcomes in diffuse large B-cell lymphoma patients.
NAR Cancer, 6(1):zcae007, 24 Feb 2024
Cited by: 0 articles | PMID: 38406263 | PMCID: PMC10894040
CDK9-55 guides the anaphase-promoting complex/cyclosome (APC/C) in choosing the DNA repair pathway choice.
Oncogene, 43(17):1263-1273, 04 Mar 2024
Cited by: 0 articles | PMID: 38433256
The CDK Inhibitor Dinaciclib Improves Cisplatin Response in Nonseminomatous Testicular Cancer: A Preclinical Study.
Cells, 13(5):368, 20 Feb 2024
Cited by: 0 articles | PMID: 38474332 | PMCID: PMC10931172
Cyclin-dependent kinases: Masters of the eukaryotic universe.
Wiley Interdiscip Rev RNA, e1816, 17 Sep 2023
Cited by: 3 articles | PMID: 37718413 | PMCID: PMC10909489
Review Free full text in Europe PMC
RNAPII-dependent ATM signaling at collisions with replication forks.
Nat Commun, 14(1):5147, 24 Aug 2023
Cited by: 2 articles | PMID: 37620345 | PMCID: PMC10449895
Go to all (69) article citations
Data
Data behind the article
This data has been text mined from the article, or deposited into data resources.
BioStudies: supplemental material and supporting data
Similar Articles
To arrive at the top five similar articles we use a word-weighted algorithm to compare words from the Title and Abstract of each citation.
A role for CDK9-cyclin K in maintaining genome integrity.
Cell Cycle, 10(1):28-32, 01 Jan 2011
Cited by: 35 articles | PMID: 21200140 | PMCID: PMC3048070
Review Free full text in Europe PMC
SIRT2 directs the replication stress response through CDK9 deacetylation.
Proc Natl Acad Sci U S A, 110(33):13546-13551, 29 Jul 2013
Cited by: 62 articles | PMID: 23898190 | PMCID: PMC3746840
Cyclin K inhibits HIV-1 gene expression and replication by interfering with cyclin-dependent kinase 9 (CDK9)-cyclin T1 interaction in Nef-dependent manner.
J Biol Chem, 286(26):22943-22954, 09 May 2011
Cited by: 12 articles | PMID: 21555514 | PMCID: PMC3123062
Kaposi's sarcoma-associated herpesvirus K-cyclin interacts with Cdk9 and stimulates Cdk9-mediated phosphorylation of p53 tumor suppressor.
J Virol, 82(1):278-290, 17 Oct 2007
Cited by: 28 articles | PMID: 17942552 | PMCID: PMC2224387
Funding
Funders who supported this work.
NCI NIH HHS (7)
Grant ID: R01 CA102729-10
Grant ID: R01 CA136933
Grant ID: K08 CA143902
Grant ID: R01 CA102729
Grant ID: R01 CA136933-04
Grant ID: R01CA136933
Grant ID: K08 CA143902-01
NIEHS NIH HHS (2)
Grant ID: P30 ES000267
Grant ID: P30ES000267
PHS HHS (1)
Grant ID: UL1 024975