Abstract
Free full text

Inducible nitric oxide synthase is present in motor neuron mitochondria and Schwann cells and contributes to disease mechanisms in ALS mice
Abstract
Amyotrophic lateral sclerosis (ALS) is a fatal neurodegenerative disease of motor neurons (MNs). The molecular pathogenesis of ALS is not understood, thus effective therapies for this disease are lacking. Some forms of ALS are inherited by mutations in the superoxide dismutase-1 (SOD1) gene. Transgenic mice expressing human Gly93 → Ala (G93A) mutant SOD1 (mSOD1) develop severe MN disease, oxidative and nitrative damage, and mitochondrial pathology that appears to involve nitric oxide-mediated mechanisms. We used G93A-mSOD1 mice to test the hypothesis that the degeneration of MNs is associated with an aberrant up-regulation of the inducible form of nitric oxide synthase (iNOS or NOS2) activity within MNs. Western blotting and immunoprecipitation showed that iNOS protein levels in mitochondrial-enriched membrane fractions of spinal cord are increased significantly in mSOD1 mice at pre-symptomatic stages of disease. The catalytic activity of iNOS was also increased significantly in mitochondrial-enriched membrane fractions of mSOD1 mouse spinal cord at pre-symptomatic stages of disease. Reverse transcription-PCR showed that iNOS mRNA was present in the spinal cord and brainstem MN regions in mice and was increased in pre-symptomatic and early symptomatic mice. Immunohistochemistry showed that iNOS immunoreactivty was up-regulated first in spinal cord and brainstem MNs in pre-symptomatic and early symptomatic mice and then later in the course of disease in numerous microglia and few astrocytes. iNOS accumulated in the mitochondria in mSOD1 mouse MNs. iNOS immunoreactivity was also up-regulated in Schwann cells of peripheral nerves and was enriched particularly at the paranodal regions of the nodes of Ranvier. Drug inhibitors of iNOS delayed disease onset and significantly extended the lifespan of G93A-mSOD1 mice. This work identifies two new potential early mechanisms for MN degeneration in mouse ALS involving iNOS at MN mitochondria and Schwann cells and suggests that therapies targeting iNOS might be beneficial in treating human ALS.
Introduction
Amyotrophic lateral sclerosis (ALS) (also known in the US as Lou Gehrig’s disease) is a progressive, severely disabling fatal neurological disease in humans characterized by weakness, spasticity, skeletal muscle wasting, and eventual paralysis of movement, speech, swallowing, and breathing; patients die generally within 3–5 years after symptoms begin (Rowland and Shneider 2001). The cause of the spasticity, paralysis, and morbidity is progressive degeneration and loss of upper motor neurons (MNs) in cerebral cortex and lower MNs in brainstem and spinal cord (Rowland and Shneider 2001). ALS is the third most common neurodegenerative disorder with an adult onset, affecting about 2–5 in every 100,000 individuals (Rowland and Shneider 2001; Cozzolino et al. 2008). More than 5,600 people in the US are diagnosed with ALS each year (ALS Association, www.alsa.org). The disease-causing events that trigger MN degeneration are not understood and why MNs are selectively vulnerable in ALS is unclear. Two forms of ALS exist: sporadic and familial (Rowland and Shneider 2001; Bendotti and Carrì 2004; Cozzolino et al. 2008). The majority of ALS cases are sporadic with no known genetic component, except for missense mutations in TAR-DNA binding protein (Kabashi et al. 2008). Aging is a strong risk factor for ALS because the average age of onset is 55 years (ALS Association, www.alsa.org). Familial forms of ALS (fALS) have autosomal dominant or autosomal recessive inheritance patterns and make up ~10% or less of all ALS cases (Schymick et al. 2007; Turner and Talbot 2008). ALS-linked mutations occur in the genes encoding SOD1 (ALS1), Alsin (ALS2), senataxin (ALS4), vesicle associated membrane protein (VAMP/synaptobrevin)-associated protein B (ALS8), dynactin, TAR-DNA binding protein, and fused in sarcoma (FUS, ALS6) (Martin 2006; Schymick et al. 2007; Turner and Talbot 2008).
Mutations in the SOD1 gene account for ~20% of all fALS cases (~2% of all ALS cases) (Deng et al. 1993; Rosen et al. 1993). SOD1 (also known as copper/zinc SOD) is a metalloenzyme of 153 amino acids (~16 kDa) that binds one copper ion and one zinc ion per subunit. SOD1, functioning as a ~32 kDa non-covalently linked homodimer, is responsible for the detoxification and maintenance of intracellular superoxide anion (O2−) concentration in the low femtomolar range by catalyzing the dismutation of O2− to molecular oxygen and hydrogen peroxide (O2− + O2− + 2H+ → H2O2 + O2) (McCord and Fridovich 1969). SOD1 is ubiquitous (intracellular SOD concentrations are typically ~10–40 μM) in most tissues and possibly greater in neurons (Rakhit et al. 2004). SOD1 mutants appear to gain a toxic property or function, rather than having diminished O2− scavenging activity (Deng et al. 1993; Borchelt et al. 1994; Yim et al. 1996), and this toxicity might involve nitric oxide (NO•) (Beckman et al. 1993, 2001). Cellular stresses resulting from reactive oxygen species (ROS) and reactive nitrogen species (RNS) have been implicated in human ALS pathogenesis, and in animal and cell models of ALS (Martin 2006). One particular pathway for MN toxicity involves NO•, which can be synthesized by three isoforms of nitric oxide synthase (NOS) enzymes: neuronal or NOS1, inducible or NOS2, and endothelial or NOS3 (Mungrue et al. 2003). Although NO• has many beneficial cellular functions, it can react with superoxide radical (O2 •) to form the potent oxidant peroxynitrite (ONOO−) that can damage protein, lipids, and nucleic acids (Pacher et al. 2007). Inducible NOS (iNOS) differs from NOS1 and NOS3 because it is active constitutively in a calcium-independent manner and is active for extended periods yielding high-output NO• (MacMicking et al. 1997; Lowenstein and Padalko 2004). Although iNOS is studied most commonly in the context of the immune system, tissue inflammation, and macrophage function (MacMicking et al. 1997; Lowenstein and Padalko 2004), iNOS is also present in the nervous system and is expressed by subsets of glial cells and neurons (Heneka and Feinstein 2001). Interestingly, normal MNs neurons express constitutively iNOS at low levels (Martin et al. 2005), and after axotomy iNOS is up-regulated in MNs and is involved directly in their apoptotic death (Martin et al. 2005; Martin and Liu 2002). Thus, a gain in the activity of iNOS in response to certain signals can cause some forms of MN degeneration.
In the present experiments, we examined further the contribution of iNOS to the pathogenesis of ALS in a mutant SOD1 (mSOD1) mouse model. Our goals were to measure the levels and activity of iNOS in the mSOD1 mouse nervous system, to determine the cellular and subcellular localizations of iNOS, and to determine if pharmacological interventions using iNOS inhibitors could ameliorate disease. Our findings strongly implicate iNOS in the disease mechanisms of ALS in mice.
Materials and methods
Animal model
A common mutation in human SOD1 is the substitution of glycine by alanine at position 93 (G93A). Transgenic (tg) mice that express this mutant form of human SOD1 linked to fALS (Gurney et al. 1994; Dal Canto and Gurney 1994) are used widely as an animal model of ALS (Bendotti and Carrì 2004; Martin and Liu 2004; Cozzolino et al. 2008; Turner and Talbot 2008). The effects of this human mutant gene on mice are profound. Hemizygous tg mice expressing a high copy number of the G93A variant of mutant SOD1 (mSOD1) become completely paralyzed and die at ~16–18 weeks of age (Gurney et al. 1994). MNs in mice expressing G93Ahigh-mSOD1 undergo prominent degeneration; about 70% of lumbar MNs are eliminated by end-stage disease (Martin et al. 2007). Tg mice expressing either the human mutant SOD1 gene or the human wild-type (wt) human SOD1 gene (Gurney et al. 1994) were used in this study. The mouse line (B6SJL-TgN[SOD1-G93A]1Gur, G1H, Jackson Laboratory, Bar Harbor, ME) with a high copy number of mutant allele (~20 copies) and a rapid disease onset was used. Mice were studied throughout the course of disease generally from pre-symptomatic stages (<10 weeks) to early symptomatic stages (10–14 weeks) to late stages of disease (15–18 weeks). Mice were classified specifically by visible neurologic signs as pre-symptomatic, early-stage disease, or late-stage disease by their hindlimb reflex appearance when held by the tail, gait and posture, and paralysis, respectively. Controls were tg mice expressing the normal allele of the human SOD1 gene (B6SJL-TgN[SOD1]2Gur, Jackson Laboratory) studied at the same ages as the mutants. Animal protocols were approved by the Johns Hopkins University Institutional Animal Care and Use Committee.
Western blots and immunoprecipitation
To determine the levels of iNOS protein in wtSOD1 and mSOD1 tg mice spinal cord, Western blotting (WB) and immunoprecipitation (IP) were used. mSOD1 mice at pre-symptomatic (n = 4–6) and early symptomatic (n = 4–6) stages of disease, along with age-matched and wtSOD1 tg mice (n = 4), were killed by isoflurane overdose and decapitated. Spinal cords were isolated and homogenized, followed by differential centrifugation into nuclear, soluble, and mitochondrial-enriched membrane fractions as described (Martin et al. 2003). Spinal cord protein samples (10–20 μg) and purified recombinant mouse iNOS (Calbiochem) were subjected to SDS-PAGE, followed by electrophoretic transfer to nitrocellulose membranes. The quality of the SDS-PAGE and transfer was assessed by Ponceau S staining. These membranes were blocked with 2.5% dry milk/0.1% Tween 20/Tris–buffered saline (TBS) and then incubated with antibody to iNOS. Four different antibodies to iNOS were used: mouse monoclonal C-11 antibody to mouse iNOS C-terminus (Santa Cruz Biotechnology), mouse monoclonal clone 6 antibody to mouse iNOS C-terminus (BD Biosciences), rabbit polyclonal antibody to mouse iNOS N-terminus (Upstate Biotechnology), and rabbit polyclonal antibody to mouse iNOS C-terminus (Sigma). Primary antibody binding was detected with HRP-conjugated secondary antibodies (BioRad), followed by a chemiluminescent HRP substrate (Pierce). Immunoblots were exposed to X-ray film to visualize immunoreactivity. Blots were re-probed with monoclonal antibody 6C5 to glyceraldehyde phosphate dehydrogenase (GAPDH, Research Diagnostics International) for a protein loading control. iNOS immunoreactivity levels relative to GAPDH levels on films were quantified using densitometry (Quantity One).
For IP, as described previously in more detail (Golden et al. 2003), samples of spinal cord protein fractions (50 μg) were pre-cleared with Protein A/G Sepharose (Pierce), followed by incubation with iNOS primary antibody (Santa Cruz, sc-7271 or Upstate, 06-573). Samples were then incubated with Protein A/G Sepharose to form sepharose-antibody–iNOS complexes. These complexes were captured by centrifugation. Precipitated proteins were isolated by boiling samples in 4× treatment buffer and subjected to SDS-PAGE and WB. Films were quantified using computer densitometry program (Quantity One). IgG bands were quantified as loading controls.
NOS enzyme activity assay
Mice classified as pre-symptomatic, early-stage disease, late-stage of disease, and controls (wtSOD1 tg) were used (n = 6 mice/group). Spinal cords were collected freshly and tissue homogenates obtained as described above. A NOS biochemical assay kit (Cayman Chemical, 781001) was used to measure enzymatic activity of iNOS in spinal cord preparations of mSOD1 mice. L-arginine is consumed by NOS enzymes using a variety of cofactors and electron carriers, producing NO· and L-citrulline (Mungrue et al. 2003); the NOS assay kit measures production of NO· in vitro by measuring conversion of [14C] radiolabeled L-arginine to L-citrulline using a modification of the method described previously (Bhardwaj et al. 1997). To detect the calcium-independent enzymatic activity of iNOS activity, the reaction mixtures omitted calcium ion and calmodulin, which were required for other NOS isoforms (e.g., nNOS). Alternatively, a highly specific inhibitor of iNOS (S-methylisothiourea sulfate, SMT; Santa Cruz Biotechnology, sc-3566; Southan et al. 1995) was included in the reaction mix to assay selectively for nNOS activity. Samples were incubated with the kit’s reaction mix, which includes cofactors (e.g., FAD, NADPH) and [14C] radiolabeled L-arginine. After 1 h of reaction time, reactions were terminated using a low pH stop buffer included in the kit. A negatively charged resin was then suspended with the reaction mixture, binding the positively charged, unreacted L-arginine. The solution was placed in spin cups containing filters, and the solutions centrifuged briefly. The filter retained the resin bound to leftover L-arginine, and a liquid solution was eluted that contained neutrally charged, reacted L-citrulline unbound by resin. The L-citrulline that was eluted corresponded to NO· produced, since the stoichiometry of NOS produced one L-citrulline molecule and one NO· molecule for every L-arginine molecule consumed. The eluent was combined with 5 ml scintillation fluid and run through a scintillation counter. The efficacy of iNOS and nNOS selective conditions were confirmed by performing NOS activity assay in the absence of inhibiting conditions (i.e., including Ca2+/calmodulin or omitting SMT). Activity was controlled against background reactivity (incubating [14C] L-arginine with purified iNOS) and converted from decompositions per minute to nmol/min of NO·.
Immunohistochemistry
mSOD1 and wtSOD1 tg mice at 7, 8, 9, 10, 11, 14, and 15 weeks of age (n = 6/geneotype/age) were anesthetized with an overdose of chloral hydrate and perfused intracardially with ice-cold 100 mM phosphate-buffered saline (PBS; 0.9% NaCl, pH 7.4) followed by ice-cold 4% paraformaldehyde. After perfusion fixation, spinal cords remained in situ for 2 h before they were removed from the vertebral column and then placed in 20% glycerol for cryoprotection. Transverse serial symmetrical sections of lumbar spinal cord (40 μm) were obtained by frozen sectioning on a sliding microtome and stored in 96-well plates with one section/per well.
Selected sections of lumbar spinal cord (every two in ten series) were immunostained for iNOS using the immunoperoxidase method as done before (Martin et al. 2007, 2009). Sections were first permeabilized by 0.4% triton-x/TBS and then blocked with a solution of 4% normal goat serum/0.1% triton-x/TBS. Sections were then incubated in primary antibody to iNOS. Two different antibodies to iNOS were used for immunohistochemistry: mouse monoclonal C-11 antibody to mouse iNOS C-terminus (Santa Cruz Biotechnology) and mouse monoclonal clone 6 antibody to mouse iNOS C-terminus (BD Biosciences). After incubation in primary antibody, affinity-purified goat anti-mouse secondary antibody was applied, followed by peroxidase anti-peroxidase. Antibody binding to iNOS was visualized using diaminobenzidine (DAB) as chromogen. Labeling intensity of individual MNs was quantified by computer densitometry using IPLab Gel.
Immunofluorescence
Immunohistochemistry using dual-label immunofluorescence was done as before (Martin et al. 2007, 2009) to identify iNOS at various organelles and in different types of cells. Lumbar spinal cord sections were permeabilized and blocked by incubation in 5% normal goat serum and 0.4% triton-x/TBS. iNOS was detected with mouse monoclonal primary antibody (Santa Cruz) in combination with different rabbit or sheep second primary bodies for co-localization analyses. Mitochondria were identified by MnSOD (SOD2) by rabbit polyclonal antibody (Stressgen). Peroxisomes were identified using sheep antibody to catalase (BioDesign International). Microsomes were visualized by rabbit antibody to cytochrome P450 reductase (Upstate Biotechnology). Microglial cells were identified with rabbit polyclonal antibodies (Affinity Bioreagents) to the integrin protein CD11b (Akiyama and McGeer 1990). Astrocytes were detected with rabbit polyclonal antibodies (DAKO) to glial fibrillary acidic protein (GFAP). Schwann cells in the ventral roots/peripheral nerve were identified with rabbit antibodies to p75-low affinity neurotrophin receptor (Promega) and vimentin (Chemicon), as shown before to be markers for Schwann cells (Autilio-Gambetti et al. 1982; Johnson et al. 1988). Secondary antibodies conjugated with Alexa-488 or Alexa-594 were applied and sections were viewed using epifluorescence microscopy.
Reverse transcription-polymerase chain reaction (RT-PCR)
To corroborate findings based on iNOS antibody approaches, RT-PCR was used to analyze mRNA expression for iNOS in mSOD1 mice. Total RNA was extracted using TRIzol (Invitrogen) from mouse whole cerebrum and from brainstem (medulla containing cranial nerve nuclei V and VII) and spinal cord ventral isolated freshly by micro-dissection and micro-punching. cDNA synthesis was accomplished using SuperScript One-Step RT-PCR with Platinum Taq (Invitrogen) followed by PCR. Two different sets of oligonucleotide primer pairs were used to amplify mouse iNOS cDNA. One set of primers, commercially available (R&D Systems), amplifies a 513-bp product. Another set of primer pairs used were chosen from the published cDNA sequence of mouse iNOS (Lyons et al. 1992) and were 5′-AGCATCACCCCTGTGTTCCACC-3′(sense) and 5′-TGGGACAGTCTCCATTCCCA-3′(anti-sense). These primers amplify a 388-bp product (Wu et al. 2003). Oligonucleotide primers for mouse voltage-dependent anion channel-1 (VDAC1), used as an RNA control for each sample, were 5′-GCTAAGGATGACTCGGCTT TAAGG-3′ and 5′-AGGTTAAGTGATGGGCTAGGATG G-3′, which give a 335-bp amplification product (Massa et al. 2000). The PCR products were separated on a 1.0% agarose gel, stained with ethidium bromide and imaged using a BioRad molecular imaging VersaDoc system.
Pharmacological intervention
To determine if the activity of iNOS participates directly the mechanisms of disease in ALS mice, we conducted pharmacological studies using iNOS inhibitors. Two different highly specific inhibitors of iNOS were used. A small-scale study was done on a cohort of mSOD1 mice (n = 5) injected intraperitoneally with SMT (Santa Cruz Biotechnology), a potent competitive inhibitor of iNOS (Southan et al. 1995), at a dosage of 5 mg/body weight daily starting at 9 weeks of age. mSOD1 mice receiving normal saline vehicle were controls. A larger-scale study was done on a cohort of mSOD1 mice (n = 12) injected subcutaneously with 1400 W (Sigma), a slow tight-binding potent iNOS inhibitor (Garvey et al. 1997) at a dosage of 3 mg/body weight on alternate days, starting at 6 weeks of age. mSOD1 mice receiving normal saline/cyclodextran vehicle were controls. Lifespan was the outcome measurement.
Data analysis
Group means and variances were evaluated statistically by one-way ANOVA and a Newman–Keuls post hoc test.
Photography and figure construction
The original images of immunohistochemical preparations used for figure construction were generated using digital photography. Digital images were captured as TiFF files using a SPOT digital camera and SPOT Advanced software (Diagnostic Instruments) or a Nikon digital camera (DXM1200) and ACT-1 software. Images were altered slightly for brightness and contrast using ArcSoft Photo-Studio 2000 or Adobe Photoshop software without changing the content and actual result. Figure composition was done using CorelDraw 9 software with final composite figures being converted to TiFF files. Files of composite figures were adjusted for brightness and contrast in Adobe Photoshop.
Results
iNOS protein levels are up-regulated in pre-symptomatic ALS mice
Western blots of wtSOD1 and mSOD1 tg mouse spinal cord extracts probed with iNOS antibodies showed a band of immunoreactivity at ~130 kDa (Fig. 1a), consistent with the molecular weight of full-length iNOS protein (Heneka and Feinstein 2001; Mungrue et al. 2003). Corroboration that this immunoreactive band was indeed iNOS was based on the findings that this band was strongly positive in lanes loaded with cell extracts of lipopolysaccharide-stimulated mouse macrophages (RAW 264.7 cells) or purified iNOS recombinant protein and absent in lanes loaded with tissue extracts from iNOS−/− mice (data not shown). iNOS was detected at near equivalent levels in soluble and mitochondria-enriched membrane fractions of wtSOD1 mouse spinal cord (Fig. 1a). In contrast, mSOD1 mice had greater levels of iNOS in the mitochondria-enriched membrane fractions compared to the soluble fraction, and the level of iNOS in the membranous fraction was significantly greater than that found in wtSOD1 mice (Fig. 1a, b).
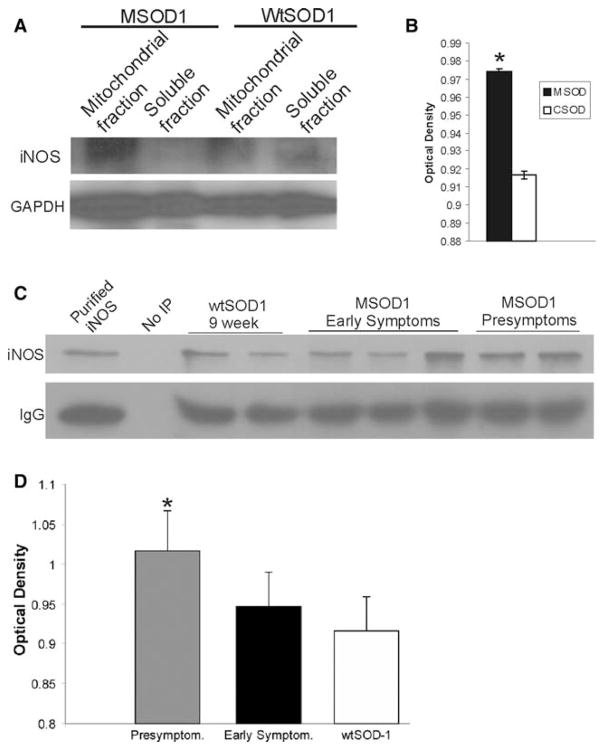
iNOS protein levels in mSOD1 and wtSOD1 tg mouse spinal cord subcellular fractions as determined by Western blotting and immunoprecipitation-Western blotting. a Standard Western blotting reveals full-length iNOS protein (~130 kDa) more enriched in the mitochondrial membrane fraction (see Martin et al. 2003 for confirmation of subcellular fractionation method) compared to the soluble fraction of pre-symptomatic mSOD1 mice, while in age-matched control tg mice expressing wtSOD1 iNOS protein is more equivalently found in mitochondrial and soluble fractions. GAPDH is shown as a loading control. b Densitometry (mean ± SD) of iNOS immunoreactive bands (normalized to GAPDH) in spinal cord mitochondrial-enriched membrane fractions of mSOD1 and wtSOD1 (control SOD, CSOD) tg mice reveals significantly (p < 0.001) greater levels in mSOD1 mice (n = 4–6 mice/group). c Immunoprecipitation of iNOS in spinal cord soluble fractions of mSOD1 and wtSOD1 mice. Purified mouse macrophage iNOS was immunoprecipitated as a positive control. Non-immunoprecipitated mSOD1 mouse spinal cord soluble fraction was a negative control. Immunoprecipitation was necessary to detect full-length 130 kDa iNOS in mSOD1 mouse spinal cord soluble fraction. IgG is shown as a control. d Optical density determinations (mean ± SD) of immunoreactive band intensity (normalized to IgG) show significantly (p < 0.05) greater levels of iNOS in pre-symptomatic mSOD1 mice (n = 4–6 mice/group)
To detect and measure the full-length iNOS protein in its native state, IP followed by WB was used (Fig. 1c). iNOS immunoprecipitated from wtSOD1 and mSOD1 tg mouse spinal cord migrated as a 130-kDa band corresponding to an immunoreactive band of immunoroprecipitated purified iNOS from mouse macrophage cells (Fig. 1c). Computer densitometry of this 130-kDa band, controlled against the IgG heavy chain labeling, demonstrated a significant increase in the level of iNOS in pre-symptomatic mSOD1 mouse spinal cord compared to wtSOD1 mouse spinal cord (Fig. 1d).
NOS activity is increased in pre-symptomatic and early symptomatic ALS mice
To determine the functional activity of iNOS in mSOD1 mice, a NOS biochemical assay was employed to measure enzymatic conversion of radiolabeled L-arginine to L-citrulline. As negative controls, reactions were incubated with known inhibitors of iNOS and nNOS that confirmed the assay to be effective and specific (Fig. 2a). Specific iNOS activity was found in nuclear-enriched, soluble, and mitochondrial membrane-enriched fractions of mouse spinal cord (Fig. 2b). In the mitochondrial membrane-enriched fraction, iNOS activity was increased significantly in mSOD1 mice compared to wtSOD1 mice at early symptomatic stages of disease (Fig. 2b). iNOS activity was not significantly different in nuclear-enriched and soluble fractions of mSOD1 mice (Fig. 2b). nNOS activity was measured to determine if the changes in iNOS activity were isoform specific. nNOS activity was detected in soluble and mitochondrial subcellular compartments of spinal cord. nNOS activity was increased significantly in the mitochondrial-enriched membrane compartment of mSOD1 mice compared to wtSOD1 mice at the pre-symptomatic stages of the disease (Fig. 2c).
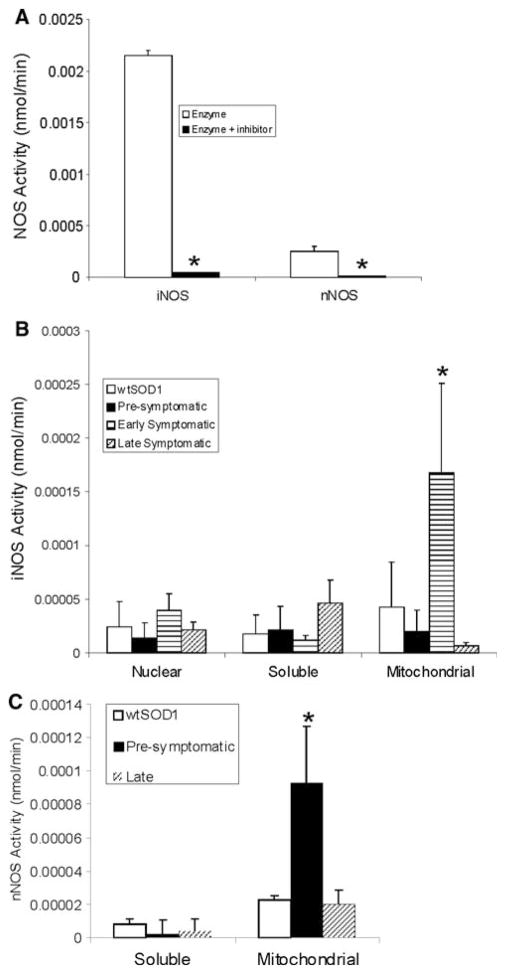
NOS catalytic activity in mSOD1 and wtSOD1 tg mouse spinal cord subcellular fractions as determined by biochemical isotopic assay. a Use of specific inhibitors of recombinant iNOS (S-methylisothiourea sulfate) and nNOS (provided in kit) demonstrates the effectiveness and specificity of the assay. b iNOS activity (mean ± SD) was detected in nuclear, soluble, and mitochondrial-enriched membrane fractions of in mSOD1 and wtSOD1 tg mouse spinal cord. iNOS activity was increased significantly (p < 0.05) in mitochondrial fractions of mSOD1 mice at early stages of disease. c nNOS activity (mean ± SD) was detected in soluble and mitochondrial-enriched membrane fractions of in mSOD1 and wtSOD1 tg mouse spinal cord. nNOS activity was increased significantly (p < 0.01) in mitochondrial fractions of mSOD1 mice at pre-symptomatic stages of disease
iNOS immunoreactivity is increased in mSOD1 MNs and microglia
Immunohistochemical staining of iNOS using specific antibodies confirmed by Western blotting showed increases in iNOS immunoreactivity in motor neurons during the progression of disease (Fig. 3a–d). iNOS immunoreactivity was seen as dot-like particles and aggregates in the cytoplasm of the somatodendritic compartment and nuclear compartment of MNs (Fig. 3a–c, h, i). MNs in wtSOD1 mice maintained a steadily low level of iNOS immunoreactivity at 7 through 15 weeks of age (Fig. 3a), similar to that seen before in non-transgenic mouse MNs (Martin et al. 2005); in contrast, mSOD1 mice showed progressively increased immunoreactivity throughout this time course (Fig. 3b–d). The levels of iNOS immunoreactivity in symptomatic mSOD1 mice reached an optical density of more than 3.3 times greater than the average optical density in wtSOD1 mice (Fig. 3d). The iNOS localization pattern in MNs of pre-symptomatic and symptomatic mSOD1 mice differed markedly from that seen in wtSOD1 tg mice. The increased iNOS immunoreactivity occurred specifically in MNs in mice at the pre-symptomatic and early symptomatic stages of disease and then later also in cells appearing as microglia (Fig. 3c). The rich iNOS immunostaining in MNs of pre-symptomatic mSOD1 mice was confirmed by immunofluoresence (Fig. 4g). iNOS immunoreactivity specifically in microglia was demonstrated by dual labeling for iNOS and CD11b (Fig. 4a–c). The co-localization of iNOS and CD11b was common and robust (Fig. 4a–c). In advanced disease, iNOS-positive microglia and their processes were found closely associated with, and perhaps penetrating, iNOS-positive degenerating and remnant MNs (Fig. 4d–f). iNOS immunoreactivity in pre-symptomatic mSOD1 mouse spinal cord was not associated with astrocytes identified by GFAP immunostaining (Fig. 4g–i), but at end-stage disease some infrequent co-localization of iNOS and GFAP was observed (Fig. 4j–k).
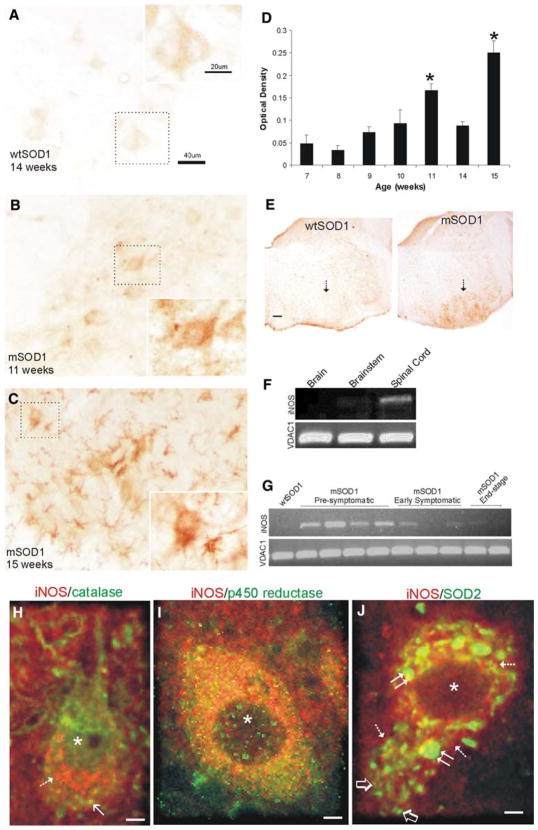
Immunohistochemical localization of iNOS protein and RT-PCR analysis of iNOS mRNA in mSOD1 and wtSOD1 tg mice. Immunoperoxidase staining for iNOS in lumbar spinal cord sections of 14-week-old wtSOD1 tg mouse (a) and mSOD1 tg mice (b, c) at 11 weeks of age (early symptomatic) and 15 weeks old (end-stage). iNOS immunoreactivity is seen as brown labeling. Insets show delineated MNs at higher magnification. Scale bars (in a) apply to b and c. d Single-cell computer densitometry (mean ± SD) of immunohistochemically stained motor neurons (n = ~25–30 MNs/group) shows significantly (p <0.01) increased iNOS immunoreactivity in MNs of mSOD1 mice at 11 and 15 weeks of age (compared to baseline at 7 weeks of age). e Immunoperoxidase staining for iNOS in brainstem sections from a 14-week-old wtSOD1 tg mouse (left) and a 9-week-old (pre-symptomatic) mSOD1 tg mouse (right). iNOS immunoreactivity is seen as brown labeling. The facial motor nucleus (arrow) shows high levels of iNOS immunoreactivity in mSOD1 mice. Scale bar (in e) = 130 μm. f RT-PCR analysis (see “Methods” for primers) of iNOS mRNA expression in brain (cerebrum), brainstem, and spinal cord of control mouse. In normal CNS, iNOS mRNA levels are moderate in spinal cord, low in brainstem, and not detectable in cerebrum. RT-PCR for VDAC1 mRNA expression in the same samples is shown as a control. g RT-PCR analysis of iNOS mRNA expression in microdissected brainstem (medulla containing facial and trigeminal motor nuclei) of wtSOD1 and mSOD1 tg mice. iNOS expression is highest at pre-symptomatic stages of disease. RT-PCR for VDAC1 mRNA expression in the same samples is shown as a control. Dual-label immunofluorescence for iNOS (red) and the peroxisomal marker catalase (h), the microsomal marker cytochrome p450 reductase (i), and the mitochondrial marker SOD2 (j). Asterisks identify nuclei. iNOS immunoreactivity (h, hatched arrow) is mostly distinct from catalase immunoreactivity (h, solid arrow) and thus there is sparse yellow. iNOS and cytochrome p450 reductase robustly, but incompletely, co-localize (yellow) in the cytoplasm (i). iNOS robustly localizes to SOD2-positive mitochondria as revealed by the discrete merging of red and green (j, yellow). Small (~0.5 μm in diameter), morphologically normal-appearing mitochondrial are single-labeled for green-SOD2 (j, lower left of cell, open arrows), while swollen mitochondria (double arrows) are iNOS-positive (yellow). Other structures, probably microsomes, are red-iNOS single labeled (j, hatched arrows) Scale bars h 6.7 μm; i 4.5 μm; j 7 μm
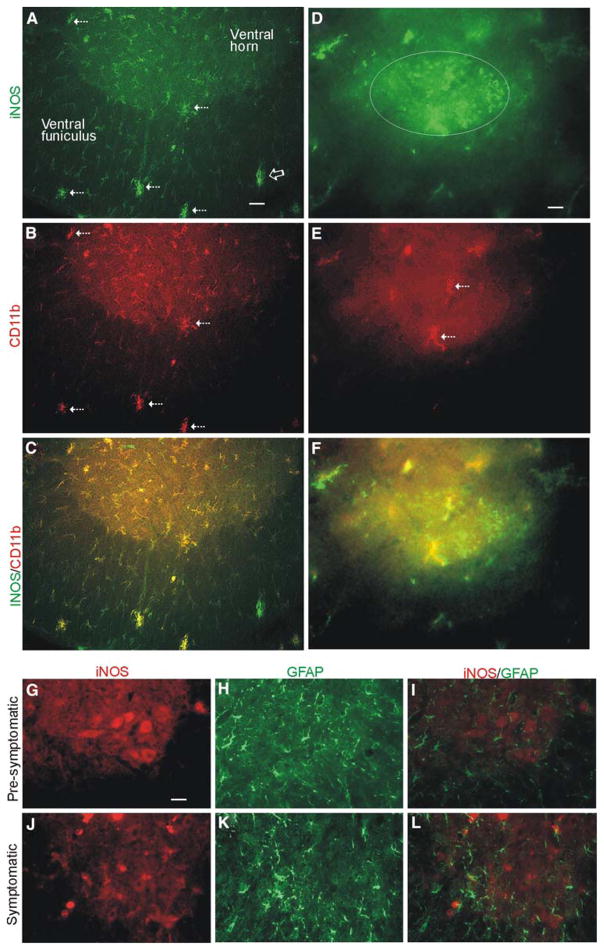
iNOS immunoreactivity is found in microglia, but rarely in astrocytes, in symptomatic mSOD1 mice. Immunolocalization of iNOS (a, green) and the microglial cell marker CD11b (b, red) demonstrates the near complete localization of iNOS to microglia (c, yellow) in spinal cord ventral horn and white matter ventral funiculus at end-stage disease. Hatched arrows identify selected microglial cells that are positive for iNOS; however, not all iNOS positive structures (a, open arrow) are CD11b-positive microglia. There is also iNOS immunoreactivity throughout the ventral horn parenchyma that appears to localize as fine microglial processes. Scale bar (in a) = 25 μm. d–f At end-stage disease remnant MNs (delineated by oval) show green-iNOS particulate immunoreactivity in the cytoplasm, while iNOS+/CD11b+ microglia (e, red at arrows) form satellites and infiltrate into the degenerating MN. Scale bar (in d) = 2.5 μm. Immunolocalization of iNOS (g, red) and the astroglial marker GFAP (h, red) demonstrate the absence of co-localization of iNOS to astrocytes (i, no yellow, compare to Fig. 4C) in pre-symptomatic mSOD1 mouse spinal cord ventral horn, but MNs are strongly positive for iNOS (g). Scale bar (in g, applies to h–l) = 35 μm. j–l. iNOS immunoreactivity is found very rarely in some astrocyte processes in symptomatic mSOD1 mice
iNOS expression is increased in mSOD1 mouse brainstem motor nuclei
The pattern of increased iNOS immunoreactivity seen in spinal MNs was also seen in cranial nerve MN nuclei in brainstem (Fig. 3e). This observation afforded an opportunity to use higher resolution micro-dissection of brainstem regions containing cranial nerve MN nuclei (CN5 and CN7) for RT-PCR. In normal mouse CNS, constitutive levels of iNOS mRNA were undetectable in cerebrum, low in brainstem, and highest in spinal cord (Fig. 3f) consistent with the constitutive expression of iNOS in MNs (Martin et al. 2005). A comparison of iNOS mRNA expression in wtSOD1 and mSOD1 mouse brainstem revealed consistently elevated levels of iNOS mRNA in pre-symptomatic mSOD1 mice (Fig. 3g). Surprisingly, mSOD1 mice generally expressed lower levels of iNOS mRNA at early symptomatic and end-stage disease compared to the pre-symptomatic stages (Fig. 3g).
iNOS immunoreactivity is localized to mSOD1 mouse MN mitochondria
iNOS immunoreactivity in MN cell bodies was seen in the cytoplasm as fine discrete dots, larger round or oval particles, and as diffuse labeling (Fig. 3a–c, h–j). Dual labeling for iNOS and organelle markers was done to identify the subcellular localization of iNOS in MNs. iNOS immunoreactivity was largely distinct from the peroxisomal compartment identified by catalase (Fig. 3h). In contrast, the fine diffusely particulate iNOS immunoreactivity in MNs of mSOD1 mice showed registration with the microsomal compartment identified by cytochrome p450 reductase (Fig. 3i) and the larger particulate iNOS immunoreactivity co-localized with mitochondrial marker SOD2 (Fig. 3j). In mSOD1 mouse motor neurons with mitochondrial swelling, iNOS was consistently localized to swollen mitochondria while normal-sized mitochondria were mostly iNOS-negative (Fig. 3j).
iNOS is expressed by Schwann cells in mSOD1 mice and is enriched at the paranodal regions of nodes of Ranvier
In the course of analyzing the cellular localization of iNOS in mSOD1 mouse spinal cord, we found serendipitously iNOS immunoreactivity enriched at the ventral root exit zones of the peripheral nerves (Fig. 5a). This iNOS immunoreactivity appeared to be associated with the myelin sheaths of MN axons (Fig. 5a) and was enriched particularly at the node of Ranvier paranodal sites of peripheral nerves (Fig. 5a, lower left inset), suggesting the expression of iNOS by Schwann cells. Many peripheral nerves of pre-symptomatic and symptomatic mSOD1 mice contained subsets of iNOS-immunoreactive axons that were ensheathed by iNOS immunoreactivity (Fig. 5b). Dual immunofluorescence for iNOS and the Schwann cell marker vimentin (Autilio-Gambetti et al. 1982) demonstrated that Schwann cells were positive for iNOS (Fig. 5c–e). p75NTR staining was also used to identify activated Schwann cells in response to injured axons (Johnson et al. 1988), confirming iNOS expression by Schwann cells (Fig. 5f–h). Dual immunofluorescence for iNOS and p75NTR also confirmed the accumulation of iNOS in swollen, degenerating axons within peripheral nerves (Fig. 5f–h).
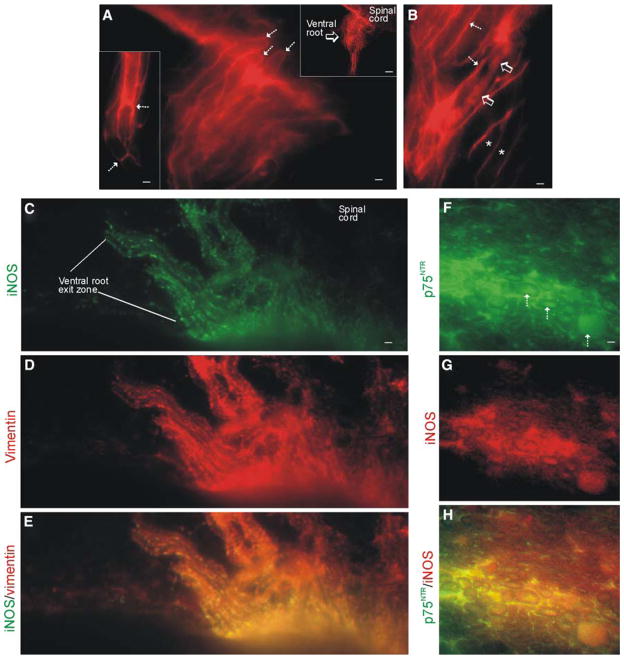
iNOS is present in Schwann cells and their paranodal zones of the nodes of Ranvier in mSOD1 mice. a iNOS immunoreactivity is present in early symptomatic (10-week-old) mSOD1 mice at the spinal nerve rootlets (upper right inset, open arrow), ventral root exit zones of the spinal nerves (hatched arrows), and nerve sheaths of peripheral nerves where iNOS is enriched highly at the paranodes of some axons (lower left inset, hatched arrows). Scale bars: a and lower left inset, 3 μm; upper right inset, 16 μm. b In pre-symptomatic mSOD1 mice, iNOS accumulates in subsets of degenerating axons (open arrows) in peripheral nerve. Axons that appear normal are iNOS-negative (asterisks) but the sheaths are iNOS-positive (hatched arrows). Scale bar 2.4 μm. c–e iNOS is expressed in the ventral root exit zone Schwann cells as demonstrated by the co-localization (yellow in e) of iNOS and the Schwann cell marker vimentin. Scale bar (in c) = 3.5 μm. f–h In symptomatic mSOD1 mice, the degenerating peripheral nerve axons and nerve interstitial components are marked by p75 neurotrophin receptor (f, hatched arrows) and these axons are iNOS-positive (yellow in h). Nerve interstitial components are also marked by p75 and iNOS. Scale bar (in f) = 6.0 μm
Inhibition of iNOS has beneficial effects on ALS mice
We studied in vivo the effects of drug inhibitors of iNOS activity on disease mSOD1 mice. In a small cohort study, ALS symptoms were delayed by administration of SMT starting at 9 weeks of age (Fig. 6a). Nevertheless, after this temporary period of ALS-like symptoms, SMT-receiving mice quickly reached end-stage disease, like vehicle-treated mice, with no extension of lifespan (Fig. 6a). In contrast, treatment of mSOD1 mice with 1400 W starting at 6 weeks of age delayed the onset of disease (Fig. 6b) and significantly extended survival, as evidenced by the 23% increase in lifespan (Fig. 6c).
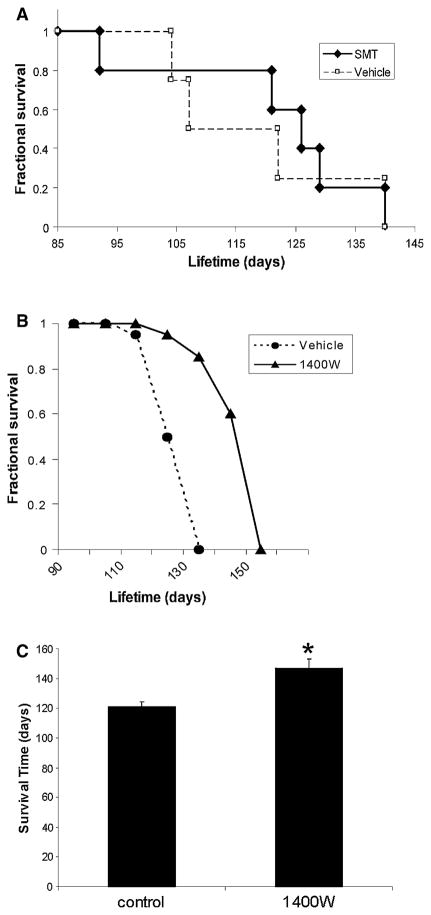
iNOS inhibitors have beneficial effects in G93Ahigh-mSOD1 tg mice. a Kaplan–Meier survival curve for small cohort study using iNOS-specific inhibitor (SMT). Mice receiving SMT delayed symptom onset (age ~90–120 days) compared to vehicle-treated mice, but had on effects on survival. b G93A mice treated with 1400W (3 mg/kg, sc every other day) had delayed disease onset and an extended lifespan compared to mice treated with vehicle (n = 10–14 mice/group). c Graph of the mean lifespan of vehicle- and 1400W-treated mSOD1 mice. Values are mean ± SD. Significant difference (*p <0.05) from vehicle control
Discussion
The disease mechanisms in mSOD1 mice are studied intensively, but clinically translatable effective mechanism-based therapies have not yet been developed from work on this animal model or any other animal model of MN degeneration (Martin and Liu 2004; Bendotti and Carrì 2004; Cozzolino et al. 2008; Turner and Talbot 2008). The focus of this study was on the role of iNOS in the pathobiology of ALS in mice. We found that iNOS mRNA, protein, and enzyme activity are up-regulated in mSOD1 mouse spinal cord and brainstem at pre-symptomatic and early symptomatic stages of disease. iNOS is expressed constitutively at low levels in mouse MNs and an early pre-symptomatic up-regulation of iNOS occurs in MNs in mSOD1 mice. iNOS in MNs of mSOD1 mice associates with mitochondria and microsomes. Finally, pharmacological inhibition of iNOS has significant effects in ALS mice by delaying disease onset and extending survival. These observations demonstrate that iNOS participates in the causal mechanisms of MN degeneration in mouse ALS.
This study is important because the role of NOS in the degeneration of MNs in mSOD1 mice has been very controversial and most studies have focused only on the nNOS (NOS1) isoform (Martin 2006). nNOS isoform and super-oxide have been implicated in the death of MNs induced by mSOD1 and Zn2+-deficient wild-type SOD1 in cell culture (Estévez et al. 1999; Raoul et al. 2002). Cultured MNs from tg mouse embryos expressing G93A, G85R, and G37R variants of mSOD1 show enhanced sensitivity to Fas death receptor-triggered cell death through a signaling pathway involving nNOS-mediated NO• production (Raoul et al. 2002). In vivo, the nNOS inhibitor AR-R 17,477 prolonged the survival of mSOD1 mice, but other nNOS inhibitors were ineffective (Facchinetti et al. 1999; Upton-Rice et al. 1999). mSOD1 mice without nNOS (α isoform) do not have prolonged survival (Facchinetti et al. 1999), but these mice still produce catalytically active β and γ isoforms of nNOS, utilizing alternate translation start sites that exclude the regions targeted by the knockout strategy (Mungrue et al. 2003), and these mice have two other NOS genes (iNOS and NOS3). iNOS has properties that are different from NOS1 and NOS3. Homodimeric iNOS is always catalytically active when expressed, because it is calcium-independent and active for extended periods (hours to days) with a Vmax ~ tenfold greater that other NOS isoforms, yielding a very high output of NO• (MacMicking et al. 1997; Lowenstein and Padalko 2004). We reported that G93Ahigh-mSOD1 mouse MNs have increased NO• production and that these mice without iNOS have significantly prolonged survival (Martin et al. 2007). However, survival of G93A-mSOD1 mice expressing a low copy number of transgene seems unaffected by iNOS gene deletion (Son et al. 2001), suggesting that the disease mechanisms in G93Ahigh-and G93Alow-expressing mice are different. Here, we show that drugs that selectively inhibit iNOS have beneficial effects in mSOD1 mice with a rapid disease onset. Thus, iNOS has a role in the pathobiology of ALS in this severe mouse model.
This study expands on the idea that changes in NO• signaling pathways are causally related to the initiation or progression of ALS. We focused on iNOS because earlier studies on mice (Almer et al. 1999; Sasaki et al. 2001a, b; Martin et al. 2007) and humans (Phul et al. 2002) have indicated that this isoform of NOS could be important in ALS, and its role in the pathobiology of ALS has been under-appreciated. We used several different methods to interrogate iNOS. iNOS was present constitutively in normal mouse spinal cord and brainstem as seen at the mRNA level using RT-PCR and protein level using WB and IP, corroborating an earlier study demonstrating iNOS immunoreactivity and activity in MNs at low levels (Martin et al. 2005). WB showed that iNOS was greater in membrane-enriched subcellular fractions than in cytoplasmic fractions, suggesting potential iNOS associations with mitochondria and microsomes; these observations were confirmed subsequently by immunohistochemical dual labeling. A salient finding was that iNOS mRNA expression and protein levels were up-regulated highest in mSOD1 mice early in the course of disease. Up-regulated iNOS mRNA in spinal cord of early symptomatic mice has been found by others (Almer et al. 1999). We then used immunohistochemistry to show that iNOS was present in normal MNs at very low levels and was up-regulated dramatically in brainstem and spinal MNs of mSOD1 mice before a robust microglial up-regulation occurring at later stages of disease. Sasaki et al. (2001a, b) and Kiaei et al. (2005) also found iNOS immunoreactivity in spinal MNs of G93A-mSOD1 mice. In addition, Sasaki et al. (2001a, b) reported that reactive astrocytes were immunostained frequently with iNOS antibody in the spinal cord at early symptomatic (32 weeks) and end-stage (35 weeks) disease in G93Alow-expressing mice, and they found in the autopsy of spinal cords of human ALS iNOS-positive MNs and astrocytes that were not observed in controls (Sasaki et al. 2000). We observed, using different antibodies, very occasional iNOS-positive astroglial elements in ALS mice with rapid disease, with the up-regulation of iNOS in microglia being much more prominent when mice were at end-stage disease. There are important distinctions between our study and the previous work by Sasaki et al. (2001a, b), notably the use of different tg mouse lines expressing very different transgene copy numbers and disease progressions, and we used complementary quantitative approaches. Almer et al. (1999) stated that iNOS was identified only in glial cells and not in neurons of G93Ahigh-mSOD1 mice, but their illustrations suggest otherwise. It is possible that abnormalities in the spinal cord neuropil microenvironment in mSOD1 mice are responsible for the iNOS induction in MNs, because many pro-inflammatory cytokines can modulate iNOS gene expression through NF-κB, JAK/STAT, and HIF-1 (Lowenstein and Padalko 2004).
We also studied the biochemical activity of iNOS and nNOS. iNOS activity was detected in nuclear, soluble, and mitochondrial-enriched membrane fractions in control and mSOD1 tg mice. nNOS activity was detected in soluble and mitochondrial-enriched membrane fractions. The changes in activity in mSOD1 mice were very selective. iNOS and nNOS activities were significantly increased only in mitochondrial-enriched membrane fractions of mice at early symptomatic and pre-symptomatic stages of disease, respectively. Almer et al. (1999) found in total spinal cord extracts nNOS activity to be unaltered early in disease and iNOS activity increased in early symptomatic and end-stage mice. Our results show an interesting disconnect between the robust microglial immunoreactivity for iNOS and low enzyme activity and mRNA in mSOD1 mice at end-stage disease. This finding might mean that our biochemical approach using subcellular fractions is disadvantageous in this regard, resulting in loss of activity, or the finding suggests that the presence of protein does not necessarily mean catalytically active enzyme due to interactions with proteins such as NAP110 (Ratovitski et al. 1999).
MNs seem to be unique among neurons regarding NO• production because they express constitutively low levels of iNOS, and iNOS is strongly up-regulated in MNs in ALS mice; thus, iNOS is the likely source of NO• in MNs degenerating in ALS. The iNOS promoter is activated by IRF-1 and NF-κB and is usually engaged by inflammation-mediated stimulation (Heneka and Feinstein 2001; Mungrue et al. 2003). This mechanism would be consistent with the concept that mSOD1 has aberrant oxidative chemistry causing an oxidative microenvironment (Liochev and Fridovich 2003), and thus high levels of NO• would favor the diffusion-limited reaction with superoxide to form peroxynitrite (Beckman et al. 1993). nNOS could also be a source of NO in degenerating MNs in mSOD1 mice (Sasaki et al. 2002), but we showed previously that NADPH diaphorase activity and iNOS immunoreactivity were induced in mSOD1 mouse MNs, but not nNOS immunoreactivity (Martin et al. 2007). Our new work here corroborates this finding with quantitative analyses using different complementary methods. mSOD1 mice develop profound mitochondrial damage in MNs (Wong et al. 1995; Kong and Xu 1998; Bendotti et al. 2001; Higgins et al. 2002; Sasaki et al. 2004; Martin 2006; Martin et al. 2007). We found that iNOS immunoreactivity becomes localized to mitochondria before and after they become swollen. Mitochondria produce NO through a reaction catalyzed by a mitochondrial form of NOS (mtNOS) with similar cofactor and substrate requirements as constitutive NOS, but mtNOS can cross-react immunologically with antibodies to iNOS (Lacza et al. 2003; Giulivi 2003). Our work extends this idea to ALS mice and demonstrates that iNOS is catalytically active in mitochondrial-enriched membrane fractions of mouse spinal cord at a time when iNOS protein accumulates in MNs but not in microglia. Our findings are consistent with an iNOS produced NO-mediated mechanism for mitochondriopathy in MNs of mSOD1 mice.
It is not completely clear to us whether the NOS activity accumulating in MNs and their mitochondria of mSOD1 mice should be called iNOS or mtNOS. True iNOS (NOS2) has a high NO output capacity and is Ca2+ independent, though it does bind calmodulin (MacMicking et al. 1997; Lowenstein and Padalko 2004); thus, its activity within MNs should be insensitive to the abnormal increases in intracellular Ca2+ in mSOD1 mouse MNs observed by us (Martin et al. 2007) and others (Siklos et al. 1998). But, if this NOS activity in MN mitochondria is indeed mtNOS, then intracellular Ca2+ fluxes could be important pathophysiologically because mitochondrial Ca2+ uptake stimulates NO production in mitochondria (Dedkova et al. 2004). Regardless of the specific isoform of NOS, abnormal NO production could drive the formation of peroxynitrite in mitochondria and the nitration of respiratory chain enzymes (e.g., cytochrome c oxidase subunit I) and mitochondrial antioxidant enzymes (e.g., SOD2) (Martin et al. 2007). Abnormal NO production in mitochondria could also explain the nitration of cyclophilin D (the ppif gene product) and the nitration of adenine nucleotide translocase seen pre-symptomatically and the formation of the mitochondrial permeability transition pore that has a critical role in the disease mechanisms of ALS mice, possibly by driving the apoptosisnecrosis cell death continuum (Martin et al. 2009; Martin 2009). Other studies have found increased protein nitration in animal models of ALS (Martin et al. 1999; Casoni et al. 2005; Martin et al. 2005). Thus, iNOS or mtNOS might be a relevant new mechanism-based target for ALS treatment.
An early abnormality in human ALS patients seen by neurologists is skeletal muscle denervation (Rowland and Shneider 2001). Similar abnormalities occur in G93A-mSOD1 mice (Fischer et al. 2004; Schaefer et al. 2005; Pun et al. 2006). These findings have fostered the proposal that MN distal axonopathy is an early initiating mechanism of ALS (Fischer et al. 2004). The possible mechanisms for this distal axonopathy could involve mitochondria. We have found that MNs in mSOD1 mice at pre-symptomatic disease accumulate mitochondria from their distal axons/terminals (Martin et al. 2007, 2009). MNs in mSOD1 mice at pre-symptomatic disease also generate higher levels of superoxide, NO, and peroxynitrite than MNs in tg mice expressing human wtSOD1 (Martin et al. 2007). We show here that Schwann cells could be another source of NO through the catalytic activity of iNOS. In peripheral nerve, Schwann cell paranodal regions and axon nodes of Ranvier have high local concentrations of mitochondria (Perkins et al. 2008), which could generate superoxide, and in combination with Schwann cell-produced NO, to form peroxynitrite locally. Moreover, we show that iNOS accumulation in peripheral nerve axons is associated with the accumulation of p75NTR. Copray et al. (2003) have also seen p75NTFR accumulate in degenerating axons and Schwann cells of ventral roots in G93A-mSOD1 mice. Interestingly, genetic deletion of p75NTR results in delayed disease onset and extended lifespan in female, but not male, G93A-mSOD1 mice (Kust et al. 2003). In human ALS, p75NTR is also up-regulated in degenerating axons and surrounding Schwann cells (Kerkhoff et al. 1991). Thus, our study implicates for the first time Schwann cells in the mechanisms of distal axonopathy in mouse ALS through their expression of iNOS, possibly triggering MN axonal damage at the nodes of Ranvier.
The molecular pathogenesis of ALS is far from being understood completely, and thus effective therapies for this disease are lacking. That is why the study of iNOS in ALS could be worthwhile. Currently, the only FDA-approved pharmaceutical to treat ALS is Riluzole, a Na+ channel blocking drug with an uncertain mechanism of action in ALS and conferring only minimal improvement in patient quality of life (Cozzolino et al. 2008). In this study, we identify 1400W as a drug that has beneficial effects in mSOD1 mice with a rapid disease onset and is known to selectively inhibit iNOS. The treatment regimen used was conservative (low dose, every other day) and we did not observe overt side effects even with chronic treatment of tg and non-tg mice, although future studies should carefully monitor blood pressure. Another important consideration is that the post-transcriptional regulation of iNOS expression is different in mouse and human cells (Pautz et al. 2009). Nevertheless, people who die from ALS also have an aberrant up-regulation of iNOS in the spinal cord (Phul et al. 2000) and importantly in MNs (Sasaki et al. 2000). Nitration of tyrosines, a signature of peroxynitrite-mediated damage, is also elevated in human ALS nervous tissues (Abe et al. 1995; Beal et al. 1997; Sasaki et al. 2000). Thus, data are available that support the involvement of iNOS in the pathobiology of human ALS. Our experiments here expand on previous work (Martin et al. 2009) demonstrating that iNOS might be a relevant mechanism-based target for human ALS treatment.
Acknowledgments
The authors thank Yan Pan, Ann Price, and Debora Flock for their technical assistance. This work was supported by grants from the US Public Health Service, NIH-NINDS (NS065895, NS052098) and NIH-NIA (AG016282).
Contributor Information
Kevin Chen, Division of Neuropathology, Department of Pathology, Johns Hopkins University School of Medicine, 558 Ross Building, 720 Rutland Avenue, Baltimore, MA 21205-2196, USA.
Frances J. Northington, Department of Pediatrics, Johns Hopkins University School of Medicine, Baltimore, MA, USA.
Lee J. Martin, Division of Neuropathology, Department of Pathology, Johns Hopkins University School of Medicine, 558 Ross Building, 720 Rutland Avenue, Baltimore, MA 21205-2196, USA, Pathobiology Graduate Program, Johns Hopkins University School of Medicine, Baltimore, MA, USA, Department of Neuroscience, Johns Hopkins University School of Medicine, Baltimore, MA, USA.
References
- Abe K, Pan L-H, Watanabe M, Kato T, Itoyama Y. Induction of nitrotyrosine-like immunoreactivity in the lower motor neuron of amyotrophic lateral sclerosis. Neurosci Lett. 1995;199:152–154. [Abstract] [Google Scholar]
- Akiyama H, McGeer PL. Brain microglia constitutively express β-2 integrins. J Neuroimmunol. 1990;30:81–93. [Abstract] [Google Scholar]
- Almer G, Vukosavic S, Romero N, Przedborski S. Inducible nitric oxide synthase up-regulation in a transgenic mouse model of familial amyotrophic lateral sclerosis. J Neurochem. 1999;72:2415–2425. [Abstract] [Google Scholar]
- Autilio-Gambetti L, Sipple J, Sudilovsky O, Gambetti P. Intermediate filaments of Schwann cells. J Neurochem. 1982;38:774–780. [Abstract] [Google Scholar]
- Beal MF, Ferrante RJ, Browne SE, Matthews RT, Kowall NW, Brown RH., Jr Increased 3-nitrotyrosine in both sporadic and familial amyotrophic lateral sclerosis. Ann Neurol. 1997;42:644–654. [Abstract] [Google Scholar]
- Beckman JS, Carson M, Smith CD, Koppenol WH. ALS, SOD and peroxynitrite. Nature. 1993;364:548. [Abstract] [Google Scholar]
- Beckman JS, Estévez AG, Crow JP, Barbeito L. Superoxide dismutase and the death of motoneurons in ALS. Trends Neurosci. 2001;24:S15–S20. [Abstract] [Google Scholar]
- Bendotti C, Carrì MT. Lessons from models of SOD1-linked familial ALS. Trends Mol Med. 2004;10:393–400. [Abstract] [Google Scholar]
- Bendotti C, Calvaresi N, Chiveri L, Prelle A, Moggio M, Braga M, Silani V, De Biasi S. Early vacuolization and mitochondrial damage in motor neurons of FALS mice are not associated with apoptosis or with changes in cytochrome oxidase histochemical reactivity. J Neurol Sci. 2001;191:25–33. [Abstract] [Google Scholar]
- Bhardwaj A, Northington FJ, Martin LJ, Hanley DF, Traystman RJ, Koehler RC. Characterization of metabotropic glutamate receptor-mediated nitric oxide production in vivo. J Cereb Blood Flow Metab. 1997;17:153–160. [Abstract] [Google Scholar]
- Borchelt DR, Lee MK, Slunt HH, Guarnieri M, Xu Z-S, Wong PC, Brown RH, Jr, Price DL, Sisodia SS, Cleveland DW. Superoxide dismutase 1 with mutations linked to familial amyotrophic lateral sclerosis possesses significant activity. Proc Natl Acad Sci USA. 1994;91:8292–8296. [Europe PMC free article] [Abstract] [Google Scholar]
- Casoni F, Basso M, Massignam T, Gianazza E, Cheroni C, Salmona M, Bendotti C, Bonetto V. Protein nitration in a mouse model of familial amyotrophic lateral sclerosis. J Biol Chem. 2005;280:16295–16304. [Abstract] [Google Scholar]
- Copray JCVM, Jaarsma D, Kunst BM, Bruggeman RWG, Mantingh I, Brouwer N, Boddeke HWGM. Expression of the low affinity neurotrophin receptor p75 in spinal motoneurons in a transgenic mouse model for amyotrophic lateral sclerosis. Neuroscience. 2003;116:685–694. [Abstract] [Google Scholar]
- Cozzolino M, Ferri A, Carri MT. Amyotrophic lateral sclerosis: from current developments in the laboratory to clinical implications. Antioxid Redox Signal. 2008;10:405–443. [Abstract] [Google Scholar]
- Dal Canto MC, Gurney ME. Development of central nervous system pathology in a murine transgenic model of human amyotrophic lateral sclerosis. Am J Pathol. 1994;145:1271–1279. [Europe PMC free article] [Abstract] [Google Scholar]
- Dedkova EN, Ji X, Lipsius SL, Blatter LA. Mitochondrial calcium uptake stimulates nitric oxide production in mitochondria of bovine vascular endothelial cells. Am J Physiol Cell Physiol. 2004;286:C406–C415. [Abstract] [Google Scholar]
- Deng H-X, Hentati A, Tainer JA, Iqbal Z, Cayabyab A, Hung W-Y, Getzoff ED, Hu P, Herzfeldt B, Roos RP, Warner C, Deng G, Soriano E, Smyth C, Parge HE, Ahmed A, Roses AD, Hallewell RA, Pericak-Vance MA, Siddique T. Amyotrophic lateral sclerosis and structural defects in Cu, Zn superoxide dismutase. Science. 1993;261:1047–1051. [Abstract] [Google Scholar]
- Estévez AG, Crow JP, Sampson JB, Reiter C, Zhuang Y, Richardson GJ, Tarpey MM, Barbeito L, Beckman JS. Induction of nitric oxide-dependent apoptosis in motor neurons by zinc-deficient superoxide dismutase. Science. 1999;286:2498–2500. [Abstract] [Google Scholar]
- Facchinetti F, Sasaki M, Cutting FB, Zhai P, MacDonald JE, Reif D, Beal MF, Huang PL, Dawson TM, Gurney ME, Dawson VL. Lack of involvement of neuronal nitric oxide synthase in the pathogenesis of a transgenic mouse model of familial amyotrophic lateral sclerosis. Neuroscience. 1999;90:1483–1492. [Abstract] [Google Scholar]
- Fischer LR, Culver DG, Tennant P, Davis AA, Wang M, Castellano-Sanchez A, Khan J, Polak MA, Glass JD. Amyotrophic lateral sclerosis is a distal axonopathy: evidence in mice and man. Exp Neurol. 2004;18:232–240. [Abstract] [Google Scholar]
- Garvey EP, Oplinger JA, Furfine ES, Kiff RJ, Laszlo F, Whittle BJR, Knowles RG. 1400 W is a slow, tight binding, and highly selective inhibitor of inducible nitric-oxide synthase in vitro and in vivo. J Biol Chem. 1997;272:4959–4963. [Abstract] [Google Scholar]
- Giulivi C. Characterization and function of mitochondrial nitric oxide synthase. Free Radic Biol Med. 2003;34:397–408. [Abstract] [Google Scholar]
- Golden WC, Brambrink AM, Traystman RJ, Shaffner DH, Martin LJ. Nitration of the striatal NA, K-ATPase α3 isoform occurs in normal brain development but is not increased during hypoxia–ischemia in newborn piglets. Neurochem Res. 2003;28:1883–1889. [Abstract] [Google Scholar]
- Gurney ME, Pu H, Chiu AY, Dal Canto MC, Polchow CY, Alexander DD, Caliendo J, Hentati A, Kwon YW, Deng HX, Chen W, Zhai P, Sufit RL, Siddique T. Motor neuron degeneration in mice that express a human Cu, Zn superoxide dismutase mutation. Science. 1994;264:1772–1775. [Abstract] [Google Scholar]
- Heneka MT, Feinstein DL. Expression and function of inducible nitric oxide synthase in neurons. J Neuroimmunol. 2001;114:8–18. [Abstract] [Google Scholar]
- Higgins CM, Jung C, Ding H, Xu Z. Mutant Cu, Zn superoxide dismutase that causes motoneuron degeneration is present in mitochondria in the CNS. J Neurosci. 2002;22(6):RC215. [Europe PMC free article] [Abstract] [Google Scholar]
- Johnson EM, Jr, Taniuchi M, DiStefano PS. Expression and possible function of nerve growth factor receptors on Schwann cells. Trends Neurosci. 1988;11:299–304. [Abstract] [Google Scholar]
- Kabashi E, Valdmains PN, Dion P, Spiegelman D, McConkey BJ, Vande Velde C, Bouchard J-P, Lacomblez L, Pochigaeva K, Salachas F, Pradat P-F, Camu W, Meininger V, Dupre N, Rouleau GA. TARDBP mutations in individuals with sporadic and familial amyotrophic lateral sclerosis. Nat Genet. 2008;40:572–574. [Abstract] [Google Scholar]
- Kerkhoff H, Jennekens FG, Troost D, Veldman H. Nerve growth factor receptor immunostaining in the spinal cord and peripheral nerves in amyotrophic lateral sclerosis. Acta Neuropathol. 1991;81:649–656. [Abstract] [Google Scholar]
- Kiaei M, Kipiani K, Chen J, Calingasan NY, Beal MF. Peroxisome proliferator-activated receptor-gamma agonist extends survival in transgenic mouse model of amyotrophic lateral sclerosis. Exp Neurol. 2005;191:331–336. [Abstract] [Google Scholar]
- Kong J, Xu Z. Massive mitochondrial degeneration in motor neurons triggers the onset of amyotrophic lateral sclerosis in mice expressing a mutant SOD1. J Neurosci. 1998;18:3241–3250. [Europe PMC free article] [Abstract] [Google Scholar]
- Kust BM, Brouwer N, Mantingh IJ, Boddeke HWGM, Copray JCVM. Reduced p75NTR expression delays disease onset only in female mice of a transgenic model of familial amyotrophic lateral sclerosis. ALS. 2003;4:100–105. [Abstract] [Google Scholar]
- Lacza Z, Snipes JA, Zhang J, Horváth EM, Figueroa JP, Szabó C, Busija DW. Mitochondrial nitric oxide synthase is not eNOS, nNOS or iNOS. Free Radic Biol Med. 2003;35:1217–1228. [Abstract] [Google Scholar]
- Liochev SI, Fridovich I. Mutant Cu, Zn superoxide dismutases and familial amyotrophic lateral sclerosis: evaluation of oxidative hypotheses. Free Radic Biol Med. 2003;34:1383–1389. [Abstract] [Google Scholar]
- Lowenstein CJ, Padalko E. iNOS (NOS2) at a glance. J Cell Sci. 2004;117:2865–2867. [Abstract] [Google Scholar]
- Lyons CR, Orloff GJ, Cunningham JM. Molecular cloning and functional expression of an inducible nitric oxide synthase from a murine macrophage cell line. J Biol Chem. 1992;267:6370–6374. [Abstract] [Google Scholar]
- MacMicking J, Xie Q-w, Nathan C. Nitric oxide and macrophage function. Annu Rev Immunol. 1997;15:323–350. [Abstract] [Google Scholar]
- Martin LJ. Mitochondriopathy in Parkinson disease and amyotrophic lateral sclerosis. J Neuropathol Exp Neurol. 2006;65:1103–1110. [Abstract] [Google Scholar]
- Martin LJ. The mitochondrial permeability transition pore: a molecular target for ALS therapy. Biochim Biophys Acta. 2009 (in press) [Europe PMC free article] [Abstract] [Google Scholar]
- Martin LJ, Liu Z. Injury-induced spinal motor neuron apoptosis is preceded by DNA single-strand breaks and is p53- and bax-dependent. J Neurobiol. 2002;5:181–197. [Abstract] [Google Scholar]
- Martin LJ, Liu Z. Opportunities for neuroprotection in ALS using cell death mechanism rationales. Drug Discov Today Dis Models. 2004;1:135–143. [Google Scholar]
- Martin LJ, Kaiser A, Price AC. Motor neuron degeneration after sciatic nerve avulsion in adult rat evolves with oxidative stress and is apoptosis. J Neurobiol. 1999;40:185–201. [Abstract] [Google Scholar]
- Martin LJ, Price AC, McClendon KB, Al-Abdulla NA, Subramaniam JR, Wong PC, Liu Z. Early events of target deprivation/axotomy-induced neuronal apoptosis in vivo: oxidative stress, DNA damage, p53 phosphorylation and subcellular redistribution of death proteins. J Neurochem. 2003;85:234–247. [Abstract] [Google Scholar]
- Martin LJ, Chen K, Liu Z. Adult motor neuron apoptosis is mediated by nitric oxide and Fas death receptor linked by DNA damage and p53 activation. J Neurosci. 2005;25:6449–6459. [Europe PMC free article] [Abstract] [Google Scholar]
- Martin LJ, Liu Z, Chen K, Price AC, Pan Y, Swaby JA, Golden WC. Motor neuron degeneration in amyotrophic lateral sclerosis mutant superoxide dismutase-1 transgenic mice: mechanisms of mitochondriopathy and cell death. J Comp Neurol. 2007;500:20–46. [Abstract] [Google Scholar]
- Martin LJ, Gertz B, Pan Y, Price AC, Molkentin JD, Chang Q. The mitochondrial permeability transition pore in motor neurons: involvement in the pathobiology of ALS mice. Exp Neurol. 2009;218(2):333–346. [Europe PMC free article] [Abstract] [Google Scholar]
- Massa R, Marlier LN, Martorana A, Cicconi S, Pierucci D, Giacomini P, De Pinto V, Castellani L. Intracellular localization and isoform expression of the voltage-dependent anion channel (VDAC) in normal and dystrophic skeletal muscle. J Muscle Res Cell Motil. 2000;21:433–442. [Abstract] [Google Scholar]
- McCord JM, Fridovich I. Superoxide dismutase, an enzymic function for erythrocuprein (hemocuprein) J Biol Chem. 1969;244:6049–6055. [Abstract] [Google Scholar]
- Mungrue IN, Bredt DS, Stewart DJ, Husain M. From molecules to mammals: what’s NOS got to do with it? Acta Physiol Scand. 2003;179:123–135. [Abstract] [Google Scholar]
- Pacher P, Beckman JS, Liaudet L. Nitric oxide and peroxynitrite in health and disease. Physiol Rev. 2007;87:315–424. [Europe PMC free article] [Abstract] [Google Scholar]
- Pautz A, Linker K, Altenhofer S, Heil S, Schmidt N, Art J, Knauer S, Stauber R, Sadri N, Pont A, Schneider RJ, Kleinert H. Similar regulation of human inducible nitric-oxide synthase expression by different isoforms of the RNA-binding protein AUF. J Biol Chem. 2009;284:2755–2766. [Abstract] [Google Scholar]
- Perkins GA, Sosinsky GE, Ghassemzadeh S, Perez A, Jones Y, Ellisman MH. Electron tomographic analysis of cytoskeletal cross-bridges in the paranodal region of the node of Ranvier in peripheral nerves. J Struct Biol. 2008;161:469–480. [Europe PMC free article] [Abstract] [Google Scholar]
- Phul R, Shaw PJ, Ince PG, Smith ME. Expression of nitric oxide synthase isoforms in spinal cord in amyotrophic lateral sclerosis. ALS Other Motor Neuron Disord. 2000;1:259–267. [Abstract] [Google Scholar]
- Pun S, Santos AF, Saxena S, Xu L, Caroni P. Selective vulnerability and pruning of phasic motoneuron axons in motoneuron disease alleviated by CNTF. Nat Neurosci. 2006;3:408–419. [Abstract] [Google Scholar]
- Rakhit R, Crow JP, Lepock JR, Kondejewski LH, Cashman NR, Chakrabartty A. Monomeric Cu, Zn-superoxide dismutase is a common misfolding intermediate in the oxidation models of sporadic and familial amyotrophic sclerosis. J Biol Chem. 2004;279:15499–15504. [Abstract] [Google Scholar]
- Raoul C, Estévez AG, Nishimune H, Cleveland DW, de Lapeyrière O, Henderson CE, Haase G, Pettmann B. Motoneuron death triggered by a specific pathway downstream of Fas: Potentiation by ALS-linked SOD1 mutations. Neuron. 2002;35:1067–1083. [Abstract] [Google Scholar]
- Ratovitski E, Alam MR, Quick RA, McMillan A, Bao C, Kozlovsky C, Hand TA, Johnson RC, Mains RE, Eipper BA, Lowenstein CJ. Kalirin inhibition of inducible nitric-oxide synthase. J Biol Chem. 1999;274:993–999. [Abstract] [Google Scholar]
- Rosen DR, Siddique T, Patterson D, Figiewicz DA, Sapp P, Hentati A, Donalsson D, Goto J, O’Regan JP, Deng H-X, Rahmani Z, Krizus A, McKenna-Yasek D, Cayabyab A, Gaston AM, Berger R, Tanzi RE, Halperin JJ, Harzfeldt B, Van den Bergh R, Hung W-Y, Bird T, Deng G, Mulder DW, Smyth C, Laing NG, Soriano E, Pericak-Vance MA, Haines J, Rouleau GA, Gusella JS, Horvitz HR, Brown RH., Jr Mutations in Cu/Zn superoxide dismutase gene are associated with familial amyotrophic lateral sclerosis. Nature. 1993;362:59–62. [Abstract] [Google Scholar]
- Rowland LP, Shneider NA. Amyotrophic lateral sclerosis. N Engl J Med. 2001;344:1688–1700. [Abstract] [Google Scholar]
- Sasaki S, Shibata N, Komori T, Iwata M. iNOS and nitrotyrosine immunoreactivity in amyotrophic lateral sclerosis. Neurosci Lett. 2000;291:44–48. [Abstract] [Google Scholar]
- Sasaki S, Shibata N, Iwata M. Neuronal nitric oxide synthase immunoreactivity in the spinal cord in amyotrophic lateral sclerosis. Acta Neuropathol. 2001a;101:351–357. [Abstract] [Google Scholar]
- Sasaki S, Warita H, Abe K, Iwata M. Inducible nitric oxide synthase (iNOS) and nitrotyrosine immunoreactivity in the spinal cords of transgenic mice with G93A mutant SOD1 gene. J Neuropath Exp Neurol. 2001b;60:839–846. [Abstract] [Google Scholar]
- Sasaki S, Warita H, Abe K, Iwata M. Neuronal nitric oxide synthase (nNOS) immunoreactivity in the spinal cord of transgenic mice with G93A mutant SOD1 gene. Acta Neuropathol. 2002;103:421–427. [Abstract] [Google Scholar]
- Sasaki S, Warita H, Murakami T, Abe K, Iwata M. Ultrastructural study of mitochondria in the spinal cord of transgenic mice with a G93A mutant SOD1 gene. Acta Neuropathol. 2004;107:461–474. [Abstract] [Google Scholar]
- Schaefer AM, Sanes JR, Lichtman JW. A compensatory subpopulation of motor neurons in a mouse model of amyotrophic lateral sclerosis. J Comp Neurol. 2005;490:209–219. [Abstract] [Google Scholar]
- Schymick JC, Talbot K, Traynor GJ. Genetics of amyotrophic lateral sclerosis. Hum Mol Genet. 2007;16:R233–R242. [Abstract] [Google Scholar]
- Siklos L, Engelhardt JI, Alexianu ME, Gurney ME, Siddique T, Appel SH. Intracellular calcium parallels motoneuron degeneration in SOD-1 mutant mice. J Neuropathol Exp Neurol. 1998;57:571–587. [Abstract] [Google Scholar]
- Son M, Fathallah-Shaykh HM, Elliott JL. Survival in a transgenic model of FALS is independent of iNOS expression. Ann Neurol. 2001;50:273. [Abstract] [Google Scholar]
- Southan GJ, Szabo C, Thiemermann C. Isothioureas: potent inhibitors of nitric oxide synthases with variable isoform selectivity. Br J Pharmacol. 1995;114:510–516. [Europe PMC free article] [Abstract] [Google Scholar]
- Turner BJ, Talbot K. Transgenics, toxicity and therapeutics in rodent models of mutant SOD1-mediated familial ALS. Prog Neurobiol. 2008;85:94–134. [Abstract] [Google Scholar]
- Upton-Rice MN, Cudkowicz ME, Mathew RK, Reif D, Brown RH., Jr Administration of nitric oxide synthase inhibitors does not alter disease course of amyotrophic lateral sclerosis SOD1 mutant transgenic mice. Ann Neurol. 1999;45:413–414. [Abstract] [Google Scholar]
- Wong PC, Pardo CA, Borchelt DR, Lee MK, Copeland NG, Jenkins NA, Sisodia SS, Cleveland DW, Price DL. An adverse property of a familial ALS-linked SOD1 mutation causes motor neuron disease characterized by vacuolar degeneration of mitochondria. Neuron. 1995;14:1105–1116. [Abstract] [Google Scholar]
- Wu F, Wilson JX, Tyml K. Ascorbate inhibits iNOS expression and preserves vasoconstrictor responsiveness in skeletal muscle of septic mice. Am J Physiol Regul Integr Comp Physiol. 2003;285:R50–R56. [Abstract] [Google Scholar]
- Yim MB, Kang J-H, Yim H-S, Kwak H-S, Chock PB, Stadtman ER. A gain-of-function of an amyotrophic lateral sclerosis-associated Cu, Zn-superoxide dismutase mutant: an enhancement of free radical formation due to a decrease in Km for hydrogen peroxide. Proc Natl Acad Sci USA. 1996;93:5709–5714. [Europe PMC free article] [Abstract] [Google Scholar]
Full text links
Read article at publisher's site: https://doi.org/10.1007/s00429-009-0226-4
Read article for free, from open access legal sources, via Unpaywall:
https://europepmc.org/articles/pmc3010349?pdf=render
Citations & impact
Impact metrics
Citations of article over time
Smart citations by scite.ai
Explore citation contexts and check if this article has been
supported or disputed.
https://scite.ai/reports/10.1007/s00429-009-0226-4
Article citations
Nuclear Localization of Human SOD1 in Motor Neurons in Mouse Model and Patient Amyotrophic Lateral Sclerosis: Possible Links to Cholinergic Phenotype, NADPH Oxidase, Oxidative Stress, and DNA Damage.
Int J Mol Sci, 25(16):9106, 22 Aug 2024
Cited by: 0 articles | PMID: 39201793 | PMCID: PMC11354607
Newly Synthesized Indolylacetic Derivatives Reduce Tumor Necrosis Factor-Mediated Neuroinflammation and Prolong Survival in Amyotrophic Lateral Sclerosis Mice.
ACS Pharmacol Transl Sci, 7(7):1996-2005, 22 Jun 2024
Cited by: 0 articles | PMID: 39022351
Integrating Transcriptomic and Structural Insights: Revealing Drug Repurposing Opportunities for Sporadic ALS.
ACS Omega, 9(3):3793-3806, 10 Jan 2024
Cited by: 2 articles | PMID: 38284068 | PMCID: PMC10809234
S-Glutathionylation and S-Nitrosylation in Mitochondria: Focus on Homeostasis and Neurodegenerative Diseases.
Int J Mol Sci, 23(24):15849, 13 Dec 2022
Cited by: 15 articles | PMID: 36555492 | PMCID: PMC9779533
Review Free full text in Europe PMC
Aberrant DNA and RNA Methylation Occur in Spinal Cord and Skeletal Muscle of Human SOD1 Mouse Models of ALS and in Human ALS: Targeting DNA Methylation Is Therapeutic.
Cells, 11(21):3448, 31 Oct 2022
Cited by: 13 articles | PMID: 36359844 | PMCID: PMC9657572
Go to all (42) article citations
Similar Articles
To arrive at the top five similar articles we use a word-weighted algorithm to compare words from the Title and Abstract of each citation.
Inducible nitric oxide synthase up-regulation in a transgenic mouse model of familial amyotrophic lateral sclerosis.
J Neurochem, 72(6):2415-2425, 01 Jun 1999
Cited by: 153 articles | PMID: 10349851
Motor neuron degeneration in amyotrophic lateral sclerosis mutant superoxide dismutase-1 transgenic mice: mechanisms of mitochondriopathy and cell death.
J Comp Neurol, 500(1):20-46, 01 Jan 2007
Cited by: 191 articles | PMID: 17099894
Transgenic mice with human mutant genes causing Parkinson's disease and amyotrophic lateral sclerosis provide common insight into mechanisms of motor neuron selective vulnerability to degeneration.
Rev Neurosci, 18(2):115-136, 01 Jan 2007
Cited by: 34 articles | PMID: 17593875
Review
The mitochondrial permeability transition pore in motor neurons: involvement in the pathobiology of ALS mice.
Exp Neurol, 218(2):333-346, 09 Mar 2009
Cited by: 123 articles | PMID: 19272377 | PMCID: PMC2710399
Funding
Funders who supported this work.
NIA NIH HHS (3)
Grant ID: R01 AG016282
Grant ID: AG016282
Grant ID: R01 AG016282-09
NINDS NIH HHS (6)
Grant ID: NS065895
Grant ID: R01 NS052098-05
Grant ID: R01 NS065895
Grant ID: R01 NS065895-02
Grant ID: NS052098
Grant ID: R01 NS052098