Abstract
Free full text

Cell Type Specific Loss of BDNF Signaling Mimics Optogenetic Control of Cocaine Reward
Abstract
The nucleus accumbens is a key mediator of cocaine reward, but the distinct roles of the two subpopulations of nucleus accumbens projection neurons, those expressing dopamine D1 vs. D2 receptors, are poorly understood. We show that deletion of TrkB, the brain-derived neurotrophic factor (BDNF) receptor, selectively from D1+ or D2+ neurons oppositely affects cocaine reward. Since loss of TrkB in D2+ neurons increases their neuronal excitability, we next used optogenetic tools to control selectively the firing rate of D1+ and D2+ nucleus accumbens neurons and studied consequent effects on cocaine reward. Activation of D2+ neurons, mimicking the loss of TrkB, suppresses cocaine reward, with opposite effects induced by activation of D1+ neurons. These results provide insight into the molecular control of D1+ and D2+ neuronal activity as well as the circuit level contribution of these cell types to cocaine reward.
The nucleus accumbens (NAc) plays a crucial role in mediating the rewarding effects of drugs of abuse (1). However, little is known about the specific function of the two major populations of NAc projection neurons, which together comprise >95% of all NAc neurons, in regulating these behaviors. These neurons, like those in the dorsal striatum, are medium spiny neurons (MSNs) divided into two subtypes based on their distinct projections through cortical-basal ganglia circuits and their differential gene expression, including enrichment of dopamine D1 vs. D2 receptors (2). These two MSN subtypes, in dorsal striatum, exert balanced but antagonistic influences on their downstream outputs and behaviors, most notably motor behaviors (3–5), but their role, in NAc, in regulating reward behaviors still needs to be determined.
While activation of both D1 and D2 receptors contributes to the rewarding effects of cocaine (6), current biochemical evidence has focused primarily on cocaine-induced molecular and structural changes in D1+ MSNs (7–11). For example, the extracellular signal-regulated kinase (ERK) pathway is induced in D1+ MSNs after cocaine exposure (8), an effect thought to be mediated directly via activation of D1 receptors (12, 13). However, ERK activation by cocaine may occur through other mechanisms, such as brain-derived neurotrophic factor (BDNF) signaling (13), since BDNF and the activated form of its receptor, TrkB, are both upregulated in the NAc after cocaine exposure (14–16). Furthermore, manipulations of BDNF and its TrkB receptor in this brain region potently modify rewarding responses to cocaine (15–19). Despite these important insights into BDNF signaling and dopaminergic transmission in the NAc, it remains unclear which MSN subtype is involved in these phenomena.
We first determined whether TrkB mRNA is differentially expressed in either MSN subtype using fluorescence activated cell sorting (FACS) to purify each MSN population from NAc and dorsal striatum of bacterial artificial chromosome (BAC) transgenic mice (20, 21) expressing enhanced green fluorescent protein (eGFP) in D1+ or D2+ MSNs (D1-GFP or D2-GFP mice) (Fig S1) (22). TrkB gene expression was observed in both neuron populations (Fig 1A), similar to previous studies demonstrating TrkB protein in each MSN subtype (23), but we observed a significant enrichment of TrkB mRNA in D2+ MSNs (Fig 1A). Further studies are needed to confirm this enrichment in D2+ MSNs of the NAc specifically (8).

(A) TrkB mRNA is expressed in D1+ and D2+ MSNs FACS-purified from D1-GFP and D2-GFP transgenic mice, but is significantly enriched in D2+ MSNs (n = 4 per group); Student’s t-test, p < 0.05). (B) D1-Cre–flTrkB (n = 9) mice displayed enhanced cocaine conditioned place preference (CPP) relative to littermate controls (n = 10), whereas (C) D2-Cre–flTrkB mice (n = 14) exhibited decreased cocaine CPP compared to littermate controls (n = 16) (cocaine dose: 7.5 mg/kg ip; Student’s t-test, **p < 0.01, *p < 0.05). (D,E) D1-Cre–flTrkB and D2-Cre–flTrkB mice and littermate controls were treated with saline on day 0 and with cocaine (10 mg/kg) on days 1–7 and locomotor activity was assessed over a 30-min time period. (D) D1-Cre–flTrkB mice (n = 6) displayed enhanced cocaine-induced locomotor activity after repeated cocaine administration compared to littermate controls (n = 7) (Repeated Measures Two-Way ANOVA, genotype effect: F(1,11) = 6.20, p < 0.05; day effect: F(6,66) = 5.50, p < 0.01), while (E) D2-Cre–flTrkB mice (n = 10) showed decreased locomotor activity to acute and repeated cocaine relative to controls (n = 14) (Repeated Measures Two-Way ANOVA, genotype effect: F(1,22) = 9.98, p < 0.01; day effect: F(6,132)= 4.00, p < 0.01). Post-hoc analysis reveals significant differences on specific cocaine days (Student’s t-test, **p <0.01, *p < 0.05). Data represented as mean ± SEM. (F–I) c-Fos induction was examined 90 min after acute cocaine (20 mg/kg) by double immuno-labeling of c-Fos (green) and Cre (red) in the NAc. (F,H) D1-Cre–flTrkB mice exhibited a significant decrease in double-labeled c-Fos (green) and Cre (red) neurons in the NAc after cocaine exposure compared to D1-Cre control mice and this down-regulation is specific to the NAc shell. (G,I) In contrast, D2-Cre–flTrkB mice, relative to D2-Cre controls, displayed an increase in double-labeled c-Fos and Cre neurons in the NAc, an effect also specific to the NAc shell (n = 4 per group, Student’s t-test, **p < 0.01, *p < 0.05). Images displayed are from the NAc shell. Arrows represent neurons double labeled with c-Fos and Cre. Arrowheads represent c-Fos neurons that are not Cre positive. Scale bars, 20 µm. Data represented as mean ± SEM. (J) Sample traces obtained by 200 pA current injection (holding potential at −80 mV) in NAc shell MSNs in D1-Cre-flTrkB, D2-Cre-flTrkB, and their control mice injected with DIO-AAV-EYFP into the NAc for visualization of D1+ or D2+ MSNs. (K,L) D2+ MSNs in D2-Cre-flTrkB NAc (n=3 animals), but not from D1+ MSNs in D1-Cre-flTrkB NAc (n=4), display increased cell excitability following incremental steps in current injections (100, 150, and 200 pA) compared to respective controls, D2-Cre (n=5) and D1-Cre (n=8). Two-way ANOVA, F(1,7) = 13.23, p = 0.002 (for D2+ MSNs), F(1,11) = 4.04, p = 0.054 (for D1+ MSNs). Post hoc analysis reveals significant effects for 100 and 150 pA currents in D2+ MSNs, Student’s t-test, *p<0.05.
To assess the functional role of BDNF-TrkB signaling in D1+ and D2+ MSNs, we used D1-Cre or D2-Cre BAC transgenic mice in which Cre recombinase is expressed under D1 or D2 promoters and their regulatory elements (Fig S2) (21, 24), combined with conditional floxed TrkB mice (flTrkB) (25). We then evaluated behavioral responses to cocaine in these mice when TrkB was selectively deleted from D1+ MSNs (D1-Cre–flTrkB) or D2+ MSNs (D2-Cre–flTrkB) in the NAc and dorsal striatum. In an unbiased cocaine conditioned place preference (CPP) paradigm, D1-Cre–flTrkB mice displayed a significant increase in cocaine preference relative to littermate controls (Fig 1B). This increase in preference is opposite to findings from previous studies that disrupted BDNF-TrkB signaling non-selectively (i.e., in both MSNs subtypes) in the NAc (15–19). However, our observations in the D2-Cre–flTrkB mice parallel these previous studies (ibid.): D2-Cre–flTrkB mice exhibited a significant decrease in cocaine preference compared to their littermate controls (Fig 1C). Importantly, these behavioral responses are likely mediated by loss of TrkB in D1+ and D2+ MSNs in the adult NAc, because both phenotypes were rescued by expressing TrkB, using herpes simplex virus (HSV-TrkB-GFP-mCherry), in the NAc of each mutant mouse line (Fig S3). The lack of developmental consequences of the TrkB deletion is also supported by several normal baseline behaviors in these mice (Fig S4).
To further explore the effects of selective deficits in BDNF-TrkB signaling on cocaine action, we analyzed locomotor activity and sensitization in the D1-Cre–flTrkB and D2-Cre–flTrkB mice. Cocaine and other stimulants increase locomotor activity upon initial exposures, with further increases (sensitization) seen after repeated exposure to the drug. Locomotor sensitization is thought to reflect biochemical adaptations that contribute to drug addiction and relapse (26). In agreement with our CPP experiments, we observed increased locomotor sensitization in D1-Cre–flTrkB mice compared to littermate controls and the opposite effect in D2-Cre–flTrkB mice (Fig 1D, E, Fig S5).
To gain insight into the functional effect of the loss of BDNF-TrkB signaling from D1+ and D2+ neurons, we first measured c-Fos induction, a marker of neuronal function, in the NAc of D1-Cre–flTrkB and D2-Cre–flTrkB mice after acute cocaine exposure (Fig 1F–I, Fig S6). Prior studies have shown the selective induction of c-Fos in D1+ MSNs in response to cocaine (8, 12). D1-Cre–flTrkB mice exhibited a significant decrease in c-Fos+ D1+ MSNs in the NAc, an effect specific to the NAc shell and not seen in the NAc core, relative to control mice (Fig 1F, H, Fig S6). In striking contrast, D2-Cre–flTrkB mice displayed a significant increase in c-Fos+ D2+ MSNs in the NAc, also specific to shell, compared to controls (Fig 1G, I, Fig S6). We observed no difference in c-Fos expression in dorsal striatum, and saline-treated controls displayed minimal c-Fos induction (Fig S6).
The c-Fos data suggest that loss of BDNF-TrkB signaling alters the function of both D1+ and D2+ NAc neurons, although the nature of the functional changes are difficult to infer, since c-Fos induction, which is often used as a marker of neuronal activation, can also indicate changes in signaling cascades without a change in firing or even neuronal inhibition (27, 28). We thus directly investigated the excitability of each MSN subtype in the NAc shell after loss of TrkB. We observed a dramatic increase in neuronal firing in response to current injections in D2+ MSNs in the D2-Cre-flTrkB mice (Fig J, L). Although no significant change in baseline excitability was observed in D1+ MSNs in the D1-Cre-flTrkB mice, there was a trend for increased excitability (p=0.054 (Fig 1J, K), and we found down-regulation of five K+ channel subunits in the NAc of D1-Cre-flTrkB mice after repeated exposure to cocaine (Table S1), consistent with enhanced excitability of these MSNs as well.
Given these direct links between loss of TrkB and enhanced excitability of NAc MSNs, we next studied directly the influence of increased activity of these MSN subtypes on behavioral responses to cocaine using optogenetic technologies (29–31). We expressed channelrhodopsin-2 (ChR2), a 473 nm blue light-activated cation channel (29), in D1+ or D2+ MSNs in the NAc using D1-Cre and D2-Cre mice and conditional adeno-associated viruses (AAVs), DIO-AAV-ChR2-EYFP and the control DIO-AAV-EYFP, that express only in the presence of Cre recombinase (31) (Fig 2A, B). The vectors were stereotaxically injected into the NAc of D1-Cre and D2-Cre mice followed by a cannula implant to which an optic fiber was secured to deliver blue light directly into the virus-infected NAc (Fig 2A–C). This approach enables temporally precise control of NAc neuronal firing (Fig 2D). To further validate the technique in vivo, we demonstrated c-Fos induction after 10 Hz blue light pulses in D1+ and D2+ MSNs expressing DIO-AAV-ChR2-EYFP (Fig 2E–G, Fig S7).

(A,B) DIO-AAV-ChR2-EYFP or DIO-AAV-EYFP was injected into the NAc of D1-Cre and D2-Cre mice resulting in ChR2-EYFP or EYFP expressing neurons (green) that also express Cre (red). Scale bars, 50 µm (low power images) and 20 µm (high power images). (C) Diagram of D1+ or D2+ ChR2 expressing MSNs and blue light emission from the optic fiber. (D) Control of neuronal firing when a NAc MSN expressing DIO-AAV-ChR2-EYFP is exposed to blue light at 1.4 or 1.0 Hz. (E) c-Fos (red) expression is induced in D1+ or D2+ MSNs expressing ChR2 (green) that have been activated with 10 Hz blue light stimulation but not EYFP expressing MSNs. Scale bars, 20 µm. (F) Quantification of E shows a significant increase in c-Fos expressing ChR2 expressing D1+ and D2+ MSNs compared to EYFP expressing controls after blue light exposure (n = 3 per group, Student’s t-test, *p < 0.01). (G) c-Fos mRNA is significantly upregulated in the NAc after blue light pulses in DIO-AAV-ChR2-EYFP expressing D1-Cre and D2-Cre mice (n = 4–5 per group, Student’s t-test, **p < 0.01, *p < 0.05).
To evaluate the behavioral response to cocaine when D1+ vs. D2+ MSNs are activated selectively, we used a CPP paradigm, in which D1-Cre and D2-Cre mice, expressing DIO-AAV-ChR2-EYFP or DIO-AAV-EYFP in the NAc, were conditioned to cocaine plus 10 Hz blue light pulses in one chamber, with saline and no light used for the opposite chamber (Fig 3A). D1-Cre mice expressing DIO-AAV-ChR2-EYFP in the NAc displayed a significant increase in cocaine/blue light preference compared to the D1-Cre DIO-AAV-EYFP control group (Fig 3B). In contrast, D2-Cre mice expressing DIO-AAV-ChR2-EYFP exhibited a significant attenuation of cocaine/blue light preference relative to controls (Fig 3C). We observed no difference in blue light preference in D1-Cre or D2-Cre mice expressing DIO-AAV-ChR2-EYFP in the absence of cocaine (Fig S8). These data implicate a role for activation of D1+ MSNs in enhancing the rewarding effects of cocaine, with activation of D2+ MSNs antagonizing cocaine reward. These findings are consistent with previous studies, in which disruption of glutamatergic NMDA receptor signaling or loss of c-Fos in D1+ MSNs reduced cocaine sensitization or CPP (11, 32). Conversely, ablation of D2+ MSNs increases the locomotor and rewarding effects of another stimulant, amphetamine (33).
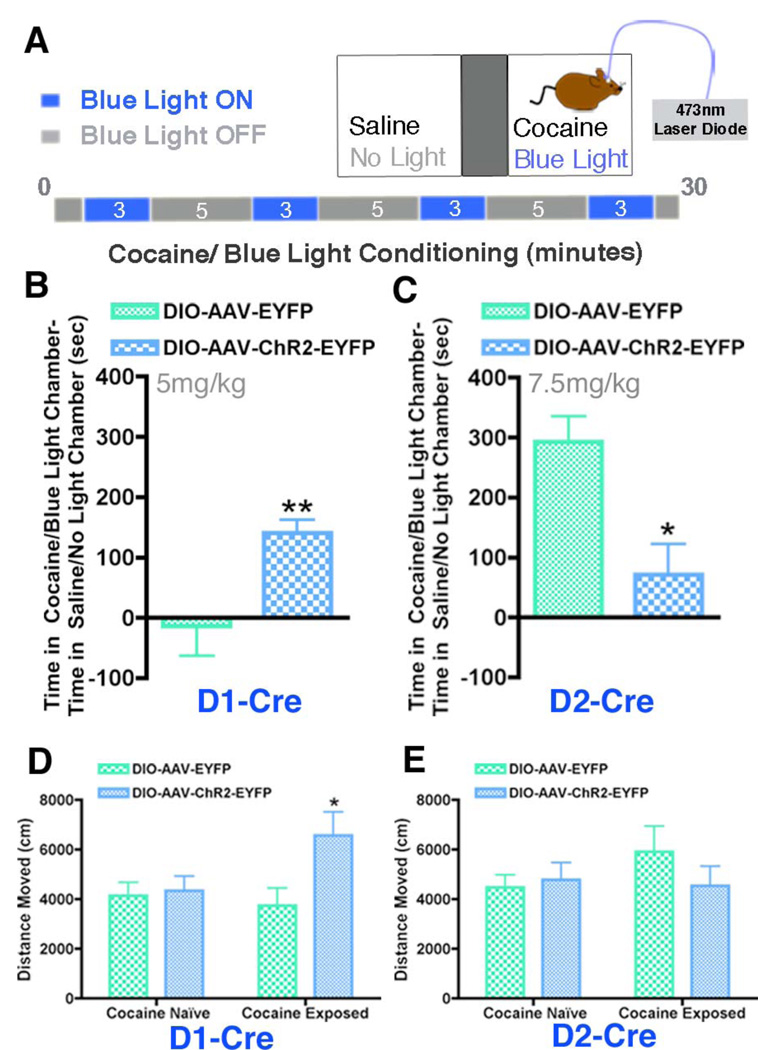
(A) Illustration of the CPP cocaine/blue light paradigm. Mice were conditioned to a cocaine/blue light chamber and a saline/no light chamber for 30 min. Blue light pulses (10 Hz) were delivered for four 3-min periods during the 30-min conditioning. (B,C) Activating D1+ MSNs in D1-Cre DIO-AAV-ChR2-EYFP mice (n = 9) during cocaine (5 mg/kg)/blue light CPP enhances cocaine reward compared to D1-Cre DIO-AAV-EYFP controls (n = 10), whereas activating D2+ MSNs in D2-Cre DIO-AAV-ChR2-EYFP mice (n = 7) during cocaine (7.5 mg/kg)/ blue light CPP attenuates cocaine reward relative to D2-Cre DIO-AAV-EYFP controls (n = 4) (Students t-test, **p < 0.01, *p < 0.05). (D,E) Optical control of D1+ and D2+ MSNs in D1-Cre and D2-Cre mice expressing DIO-AAV-ChR2-EYFP or DIO-AAV-EYFP in a cocaine naïve state and 24 hours after 6 days of repeated cocaine (15 mg/kg) results in increased locomotor activity only when D1+ MSNs are activated after cocaine exposure (Two Way ANOVA, genotype effect: F(1,22) = 4.37, p < 0.05; Student’s t-test *p<0.05)
We next evaluated locomotor activity when the two NAc MSN subtypes are activated under cocaine-naïve and exposed conditions. We detected no change in locomotion in cocaine-naïve mice when either MSN subtype was activated relative to non-stimulated controls (Fig 3D, E). However, 24 hours after 6 days of repeated cocaine administration (15 mg/kg), blue light pulses to the NAc in D1-Cre mice expressing DIO-AAV-ChR2-EYFP increased locomotor activity compared to controls (Fig 3D), with no change observed upon activation of D2+ MSNs (Fig 3E). These data are in accordance with the prevailing model of dorsal striatal function (3–5), which implicates activation of D1+ MSNs in promoting motor function, and suggest that repeated exposure to cocaine enhances the output of D1+ MSNs of the NAc.
It is unclear what behavioral effects occur when both MSN subtypes in the NAc are activated during cocaine exposure, which likely occurs through glutamatergic inputs to this brain region. There is evidence implicating a hypoactive NAc in the cocaine-addicted state (34, 35), but data also support activation of some neurons during operant cocaine behaviors and context-dependent cocaine sensitization (34, 36). Additionally, high frequency deep brain stimulation of the NAc, which may inhibit neuronal activity, attenuates an animal’s reinstatement to cocaine seeking, and excitatory AMPA receptor-mediated glutamatergic transmission has been shown to induce relapse to cocaine addiction (37, 38). We injected herpes simplex viruses, HSV-ChR2-mCherry or HSV-mCherry, into the NAc of wildtype mice (Fig 4A) to optically control global NAc neuronal activity during cocaine/blue light CPP (Fig 3A). We found that HSV-ChR2-mCherry is expressed equally in D1+ and D2+ MSNs (Fig 4D, F) and that blue light exposure induces equivalent c-Fos levels in these neurons (Fig 4B, C, E, F, Fig S7). Mice expressing HSV-ChR2-mCherry in the NAc displayed enhanced reward to cocaine/blue light relative to control mice (Fig 4G).

(A) HSV-mCherry or HSV-ChR2-mCherry (red) were injected into the NAc of wildtype mice. Scale bar 50 µm. (B) Activation of ChR2 with blue light (10 Hz) stimulation induces c-Fos (green) expression in HSV-ChR2-mCherry neurons (red), but not in HSV-mCherry neurons (red), after 10 Hz blue light. Scale bar 25 µm. (C) Quantification of c-Fos positive neurons in B and c-Fos mRNA after 10 Hz blue light shows a significant increase in c-Fos in HSV-ChR2-mCherry expressing NAc compared to HSV-mCherry expressing controls (n = 3–5 per group, Student’s t-test, **p < 0.01, *p < 0.05). (D) HSV-ChR2-mCherry (red) injection into the NAc of D1-GFP and D2-GFP mice mediates transgene expression in both D1+ and D2+ MSNs (GFP, green) and (E) c-Fos (blue) is induced by light in each MSN (green). Scale bar 10 µm. (F) Quantification of D and E shows HSV-ChR2-mCherry and blue light induced c-Fos equally in D1+ and D2+ MSNs. (G) Mice expressing HSV-ChR2-mCherry (n = 9) in the NAc displayed enhanced preference for the cocaine (5 mg/kg)/blue light chamber compared to control mice expressing HSV-mCherry (n = 8) (Student’s t-test, p < 0.05). (H,I) Blue light simulation results in a significant decrease in phospho:total ERK levels (pERK42/ERK42 and pERK44/ERK44) in the NAc of D1-Cre DIO-AAV-ChR2-EYFP mice and mice expressing HSV-ChR2-mCherry relative to their controls (DIO-AAV-EYFP or HSV-mCherry) (n = 5–8 per group, Student’s t-test *p < 0.05).
Finally, we probed for changes in the phosphorylated (active) form of ERK (pERK), which is downstream of BDNF-TrkB signaling (13) and is dynamically regulated by both neuronal activation and inhibition (39–41), to further link loss of TrkB with ChR2-induced activation of D1+ and D2+ MSNs. We observed down-regulation of pERK42 and pERK44 in D1-Cre DIO-AAV-ChR2-EYFP activated NAc compared to controls, with no change seen in D2-Cre DIO-AAV-ChR2-EYFP activated NAc (Fig 4H, I). Similar to selective activation of D1+ MSNs, we observed decreased pERK42 and pERK44 in HSV-ChR2-mCherry activated NAc (Fig H, I). Thus, optogenetic stimulation of D1+ MSNs impairs a downstream target of BDNF-TrkB signaling, which implicates common downstream effects upon loss of TrkB, and optogenetic activation, in D1+ MSNs, consistent with the common behavioral effects seen under these conditions. While no change in pERK was observed in stimulated D2+ MSNs, data cited above revealed common induction of c-Fos and increased neuronal excitability upon loss of TrkB and optogenetic stimulation (Fig 1G, I, J, L, Fig 2D–G).
The present study indicates opposite roles for D1+ and D2+ MSNs in mediating the behavioral effects of cocaine. The potent influence of BDNF-TrkB signaling in the NAc on cocaine reward (15–19) is mediated through D2+ MSNs, because TrkB mRNA is enriched in these neurons and selective deletion of TrkB from D2+ MSNs attenuates cocaine reward. Furthermore, deletion of TrkB from D2+ MSNs increases their neuronal excitability and reactivity to cocaine (as evidenced by increased c-Fos induction), and mimics the ability of direct activation of these neurons, via optogenetic tools, to attenuate behavioral responses to cocaine.
Our D1+ MSN data are more complex. We observe enhanced cocaine reward when D1+ MSNs are activated optogenetically and when TrkB is deleted selectively from them, however, the latter results in decreased c-Fos induction by cocaine without a significant change in baseline neuronal excitability. Nonetheless, the down-regulation of several K+ channel subunits in the NAc of D1-Cre-flTrkB mice suggests enhanced neuronal activity in response to cocaine exposure, which is consistent with the behavioral effects seen with optogenetic activation of D1+ MSNs. Our finding of enhanced behavioral responses to cocaine in the D1 TrkB knockouts, when cocaine induction of c-Fos is lost, is partly consistent with the report that deletion of c-Fos in D1+ MSNs potentiates cocaine reward (11), although our data contrast with other observations of this prior study which may be due to loss of c-Fos in whole striatum in that report. Furthermore, the ability of acute cocaine to induce c-Fos, which occurs selectively in D1+ MSNs, declines with repeated drug administration (8, 12, 42). The blunted c-Fos response, which we see after acute cocaine exposure in D1+ MSNs lacking TrkB, is therefore similar to wildtype D1+ MSNs that have been exposed to repeated cocaine. Finally, we cannot rule out trans-synaptic effects in these phenomena, for example, the possibility that D2+ MSNs, expressing normal levels of TrkB, may alter cocaine responses of D1+ MSNs in the D1-Cre-flTrkB mice resulting in loss of c-Fos induction.
Together, our data support a model where loss of TrkB in D2+ MSNs enhances their excitability, which then directly desensitizes the rewarding effects of cocaine. In contrast, loss of TrkB in D1+ MSNs may similarly enhance their activity, but only when exposed to cocaine as evidenced by K+ channel down-regulation, with such activity increasing the rewarding effects of cocaine. These opposite effects exerted by activation of each MSN subtype on cocaine reward is consistent with current models of basal ganglia function, which posit that D1+ vs. D2+ MSNs act in opposition through the direct and indirect pathways, respectively, to produce balanced behavioral output (3–5). It is plausible that, in the addicted brain, there may be an imbalance of these two MSNs. This imbalance may occur through an overactive D1+ MSN pathway as well as through decreased activity of D2+ MSNs, the latter mediated via enhanced BDNF-TrkB signaling. Expanding our understanding of the complex control of drug reward by the two main subtypes of NAc MSNs could help steer the development of novel treatments of drug addiction targeted selectively to D1+ vs. D2+ MSN subtypes.
References and Notes
Full text links
Read article at publisher's site: https://doi.org/10.1126/science.1188472
Read article for free, from open access legal sources, via Unpaywall:
https://europepmc.org/articles/pmc3011229?pdf=render
Subscription required at www.sciencemag.org
http://www.sciencemag.org/cgi/content/full/330/6002/385
Free to read at www.sciencemag.org
http://www.sciencemag.org/cgi/content/abstract/330/6002/385
Subscription required at www.sciencemag.org
http://www.sciencemag.org/cgi/reprint/330/6002/385.pdf
Citations & impact
Impact metrics
Citations of article over time
Alternative metrics

Discover the attention surrounding your research
https://www.altmetric.com/details/102362966
Article citations
Impaired striatal glutathione-ascorbate metabolism induces transient dopamine increase and motor dysfunction.
Nat Metab, 28 Oct 2024
Cited by: 0 articles | PMID: 39468205
Cell type-specific epigenetic priming of gene expression in nucleus accumbens by cocaine.
Sci Adv, 10(40):eado3514, 04 Oct 2024
Cited by: 1 article | PMID: 39365860 | PMCID: PMC11451531
Behavioral video coding analysis of chronic morphine administration in rats.
Biomed Rep, 21(5):168, 12 Sep 2024
Cited by: 0 articles | PMID: 39345955 | PMCID: PMC11428083
The activity-regulated cytoskeleton-associated protein (Arc) functions in a cell type- and sex-specific manner in the adult nucleus accumbens to regulate non-contingent cocaine behaviors.
Genes Brain Behav, 23(4):e12910, 01 Aug 2024
Cited by: 1 article | PMID: 39164860 | PMCID: PMC11335578
NPAS4 supports cocaine-conditioned cues in rodents by controlling the cell type-specific activation balance in the nucleus accumbens.
Nat Commun, 15(1):5971, 08 Aug 2024
Cited by: 2 articles | PMID: 39117647 | PMCID: PMC11310321
Go to all (561) article citations
Other citations
Data
Data behind the article
This data has been text mined from the article, or deposited into data resources.
BioStudies: supplemental material and supporting data
Similar Articles
To arrive at the top five similar articles we use a word-weighted algorithm to compare words from the Title and Abstract of each citation.
Loss of BDNF signaling in D1R-expressing NAc neurons enhances morphine reward by reducing GABA inhibition.
Neuropsychopharmacology, 39(11):2646-2653, 23 May 2014
Cited by: 56 articles | PMID: 24853771 | PMCID: PMC4207344
Tropomyosin-related kinase B in the mesolimbic dopamine system: region-specific effects on cocaine reward.
Biol Psychiatry, 65(8):696-701, 06 Nov 2008
Cited by: 83 articles | PMID: 18990365 | PMCID: PMC2738869
In vivo imaging identifies temporal signature of D1 and D2 medium spiny neurons in cocaine reward.
Proc Natl Acad Sci U S A, 113(10):2726-2731, 01 Feb 2016
Cited by: 187 articles | PMID: 26831103 | PMCID: PMC4791010
Neural mechanisms of the nucleus accumbens circuit in reward and aversive learning.
Neurosci Res, 108:1-5, 28 Jan 2016
Cited by: 40 articles | PMID: 26827817
Review
Funding
Funders who supported this work.
NIDA NIH HHS (9)
Grant ID: R01 DA007359-22
Grant ID: T32 DA007135-26A2
Grant ID: R01 DA007359
Grant ID: R01 DA014133-10
Grant ID: P01 DA008227
Grant ID: P01 DA008227-20
Grant ID: R01 DA014133
Grant ID: R01 DA014133-11
Grant ID: R01 DA014133-12
NIMH NIH HHS (3)
Grant ID: R01 MH051399-20
Grant ID: R01 MH051399-19
Grant ID: R01 MH051399