Abstract
Free full text
A high fat diet increases mitochondrial fatty acid oxidation and uncoupling to decrease efficiency in rat heart
Abstract
Elevated levels of cardiac mitochondrial uncoupling protein 3 (UCP3) and decreased cardiac efficiency (hydraulic power/oxygen consumption) with abnormal cardiac function occur in obese, diabetic mice. To determine whether cardiac mitochondrial uncoupling occurs in non-genetic obesity, we fed rats a high fat diet (55% kcal from fat) or standard laboratory chow (7% kcal from fat) for 3 weeks, after which we measured cardiac function in vivo using cine MRI, efficiency in isolated working hearts and respiration rates and ADP/O ratios in isolated interfibrillar mitochondria; also, measured were medium chain acyl-CoA dehydrogenase (MCAD) and citrate synthase activities plus uncoupling protein 3 (UCP3), mitochondrial thioesterase 1 (MTE-1), adenine nucleotide translocase (ANT) and ATP synthase protein levels. We found that in vivo cardiac function was the same for all rats, yet oxygen consumption was 19% higher in high fat-fed rat hearts, therefore, efficiency was 21% lower than in controls. We found that mitochondrial fatty acid oxidation rates were 25% higher, and MCAD activity was 23% higher, in hearts from rats fed the high fat diet when compared with controls. Mitochondria from high fat-fed rat hearts had lower ADP/O ratios than controls, indicating increased respiratory uncoupling, which was ameliorated by GDP, a UCP3 inhibitor. Mitochondrial UCP3 and MTE-1 levels were both increased by 20% in high fat-fed rat hearts when compared with controls, with no significant change in ATP synthase or ANT levels, or citrate synthase activity. We conclude that increased cardiac oxygen utilisation, and thereby decreased cardiac efficiency, occurs in non-genetic obesity, which is associated with increased mitochondrial uncoupling due to elevated UCP3 and MTE-1 levels.
Introduction
The metabolic syndrome, defined as central obesity accompanied by any two of hypertriglyceridaemia, decreased HDL cholesterol, hyperglycaemia and hypertension [1], is increasingly common in Western populations and is associated with increased risk of type 2 diabetes and cardiovascular disease [15]. Humans with type 2 diabetes have impaired cardiac high energy phosphate metabolism, which precedes changes in contractile function [39] and may arise from abnormal mitochondrial respiration impairing ATP synthesis. Isolated, perfused hearts from mice with type 2 diabetes have decreased efficiency when compared with wild-type mouse hearts [4, 23], possibly caused by increased mitochondrial uncoupling protein 3 (UCP3) levels [4]. UCPs allow protons to re-enter the mitochondrial matrix independent of ATP synthase (Fig. 1), a process inhibited by nucleoside di- and triphosphates, including GDP [53]. Cardiac UCP3 mRNA [50] and protein [29] levels increase with high plasma free-fatty acid (FFA) concentrations, acting via peroxisome proliferator-activated receptor α (PPARα) [31]. Levels of UCP3 in human heart have been found to correlate positively with plasma FFA concentrations [29] and lowering plasma FFAs decreased UCP3 levels and normalised efficiency in the diabetic mouse heart [23]. High plasma FFAs also increase mitochondrial uncoupling, because fatty acids are intrinsic UCP activators [36]. Activation of PPARα, via increased plasma FFAs, can also upregulate mitochondrial thioesterase-1 (MTE-1), which is believed to work in conjunction with UCP3 to increase mitochondrial uncoupling and/or fatty acid oxidation [45]. Therefore, high plasma FFAs are associated with increased mitochondrial UCP3 levels and uncoupling.
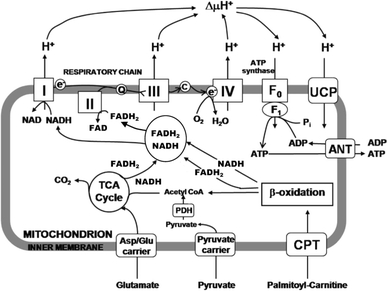
The metabolic fates of mitochondrial energy substrates. 1 Glutamate enters the mitochondrion via the aspartate/glutamate carrier and is oxidised to α-ketoglutarate by glutamate dehydrogenase. 2 Palmitoyl carnitine is imported by the carnitine palmitoyl transferase (CPT) shuttle, and oxidised by β-oxidation, to reduce FAD+ and NAD+ to FADH2 and NADH, respectively, producing acetyl CoA. 3 Pyruvate enters via the pyruvate carrier and is oxidised by pyruvate dehydrogenase (PDH) reducing NAD+ and producing acetyl CoA. Acetyl CoA enters the tricarboxylic acid (TCA) cycle, producing NADH and FADH2, which are oxidised by complexes I and II, respectively, of the electron transport chain, which consists of complexes I–IV. Electrons are transferred through the chain to the final acceptor, O2. The free energy from electron transfer is used to pump H+ out and generate an electrochemical gradient, ΔμH+, across the inner mitochondrial membrane, which is the driving force for ATP synthesis via ATP synthase. Alternatively, H+ can re-enter the mitochondria independent of ATP synthesis, via the uncoupling proteins (UCPs), resulting in the dissipation of energy
Studies that associate high cardiac UCP levels with elevated circulating FFA concentrations [4, 23, 29] have used the obese ob/ob and diabetic db/db mouse models, which have mutations in the leptin and leptin receptor genes, respectively [27, 35]. It is unknown whether cardiac UCP3 levels and mitochondrial uncoupling are elevated in milder, pre-diabetic forms of obesity, and thereby form an early stage of disease progression. Consequently, we fed rats a high fat diet (55% kcal from saturated fat) or normal laboratory chow (7% kcal from saturated fat) to induce obesity, but not overt insulin resistance. We measured cardiac function in vivo using magnetic resonance imaging (MRI) and function and efficiency (hydraulic power/O2 consumption) in isolated, perfused working hearts from these rats. We then isolated cardiac interfibrillar mitochondria and measured mitochondrial respiration rates and ADP/O ratios with glutamate, palmitoyl-carnitine or pyruvate as substrates (Fig. 1). We also measured mitochondrial UCP3, MTE-1, adenine nucleotide translocase (ANT) and F0F1 ATP synthase protein levels, as well as activities of the mitochondrial oxidation enzymes, medium chain acyl-CoA dehydrogenase (MCAD) and citrate synthase. We hypothesised that diet-induced high plasma FFA concentrations would increase cardiac mitochondrial UCP3 protein levels, thereby increasing mitochondrial uncoupling and reducing cardiac efficiency.
Materials and methods
Animals
Male Wistar rats (~300 g) (n = 36) were obtained from a commercial breeder (Harlan, Oxfordshire, UK) and housed in groups of three under controlled conditions for temperature, humidity and light, in accordance with the UK Home Office and US NIH guidelines, with water available ad libitum. All rats were initially fed standard laboratory chow ad libitum (Rat and Mouse No. 1 Maintenance, Special Diet Services, Witham, Essex, UK), which had an Atwater Fuel Energy (AFE) of 3.3 kcal/g, comprising 7.5% from oil, 17.5% from protein and 75% from carbohydrate. Three days after arriving, half the rats were switched from standard chow to a custom-produced high fat diet (Special Diet Services, Witham, Essex, UK), which had an AFE of 5.1 kcal/g, comprising 55% from oil, 29% from protein and 16% from carbohydrate. The remaining rats continued on chow. Rats were fed these diets for up to 3 weeks ad libitum, with food intake and body weights measured each day. A 3-week duration of high fat feeding was chosen as that sufficient to significantly elevate plasma FFA as compared to control animals.
Cardiac magnetic resonance imaging
After 3 weeks of feeding, anaesthesia was induced in one group of control (n = 5) and high fat-fed (n = 5) rats using 4% isoflurane in 100% oxygen. Rats were placed in a purpose built cradle for subsequent vertical positioning in the magnet bore [48]. Maintenance anaesthesia was 1.5–2% isoflurane at 1 l min−1 oxygen flow. Heart electrical activity and respiration were monitored, using an in-house electrocardiography and respiratory gating device, via subcutaneous needle electrodes inserted into the fore limbs, and a motion loop on the abdomen, respectively.
As described previously [48], magnetic resonance imaging was carried out on an 11.7 T (500 MHz) vertical bore (123-mm internal diameter) system (Magnex Scientific, Oxon, UK), using a Bruker Avance console (Bruker Medical, Ettlingen, Germany) with a linear transmit and receive, 500 MHz birdcage coil with an inner diameter of 60 mm (constructed in-house).
Long- and short-axis scout images of the heart, using a cardiac- and respiration-gated segmented fast low angle shot sequence, were used to position 7–8 contiguous, short-axis slices (slice thickness 1.5 mm) covering the entire heart. The sequence was electrocardiogram and respiration gated and between 20 and 30 frames per heart cycle were acquired, depending on the heart rate. The field of view was 5.12 × 5.12 cm with a matrix size of 256, TE/TR = 1.43/4.6 ms. One phase-encoding step was acquired per heart beat and four or eight phase encoded steps acquired per respiration cycle, resulting in a total experimental time of approximately 1 h.
Image reconstruction was performed using purpose-written software in Matlab (Mathworks, Natick, Maryland, USA). Raw data were isotropically zero-filled by a factor of two and filtered (modified third-order Butterworth filter) before Fourier transformation, resulting in an in-plane voxel size of 100 × 100 μM. Image data were exported into Scion Image (Scion Corporation, Frederick, Maryland, USA). End-diastolic and end-systolic frames were selected according to maximal and minimal ventricular volumes. In both frames, the epicardial border and left ventricular cavity were outlined and left ventricular mass was obtained by multiplying the volume by the specific gravity of myocardial tissue (1.05 g/cm3). Based on the end-systolic volumes (ESV) and end-diastolic volumes (EDV), all parameters characterising cardiac function, such as stroke volume (SV = EDV − ESV), ejection fraction (EF = SV/EDV) and cardiac output (CO = SV × heart rate) were calculated.
Isolated working heart perfusion
Another group of control (n = 7) and high fat-fed (n = 7) rats were anaesthetised with a 0.8 ml i.p. injection of 60 mg ml−1 sodium pentobarbitone (Sagatal, Rhône Mèrieux, Dublin, Ireland). Following the cessation of peripheral nervous function, hearts were excised and arrested using ice-cold modified Krebs–Henseleit buffer (118 mM NaCl, 4.7 mM KCl, 1.2 mM MgSO4, 1.75 mM CaCl2, 0.5 mM Na2EDTA, 25 mM NaHCO3, 1.2 KH2PO4). Hearts were weighed before perfusion, and then perfused in Langendorff mode for 10 min with modified Krebs–Henseleit buffer containing 11 mM glucose, 0.2 mM pyruvate and 0.5 mM lactate as substrates. After left atrial cannulation, hearts were switched to the working mode for 20 min with 200 ml of recirculating buffer. Preload was 15 mmHg and afterload was 80 mmHg. Hearts were then perfused with buffer containing 0.2 mM palmitate pre-bound to essentially fatty acid free 1.5% wt/vol bovine serum albumin (BSA) for 20 min. This was followed by 20 min perfusion with buffer containing 1 mM palmitate pre-bound to 1.5% BSA. In four experiments, hearts were perfused with buffer containing 1 mM palmitate, then 0.2 mM palmitate to ensure that the order of buffer change did not affect the results. Aortic and coronary flows were measured every 10 min and, using online measurement of aortic developed pressure, cardiac power was calculated [3]. Atrial venous oxygen concentration differences were determined using online chemifluorescence methods, which simultaneously monitored temperature-compensated oxygen partial pressure in the atrial inflow line, and from the pulmonary trunk. The oxygen content of buffer flowing in the left atrium was maintained above 550 mmHg throughout all experiments. Heart temperature was maintained at 37.4 ± 0.2°C. Myocardial oxygen consumption was calculated using the arterio-venous differences and coronary flows. Cardiac efficiency was calculated from cardiac power and myocardial oxygen consumption [3]. Hearts were snap-frozen in liquid nitrogen for subsequent biochemical analyses.
Isolation of mitochondria
A further group of control (n = 6) and high fat-fed (n = 6) rats were anaesthetised as described in the perfusion study. After the cessation of peripheral nervous function, hearts were quickly excised and arrested in ice-cold mitochondrial isolation solution (100 mM KCl, 50 mM MOPS, 1 mM EGTA, 5 mM MgSO4.7H2O, 1 mM ATP, pH 7.4). A sample of whole left ventricle (~80 mg) was washed in mitochondrial isolation solution, frozen using pre-cooled Wollenberger clamps under liquid nitrogen and stored at −80°C for later analysis of enzyme activity. The remaining left ventricular tissue was used for mitochondrial isolation. Epididymal fat pads were removed from the carcass and weighed, as an indicator of whole body adiposity [52].
Interfibrillar mitochondria were isolated from rat hearts as previously described [33] except that a modified Chappell–Perry buffer (containing 100 mM KCl, 50 mM MOPS, 1 mM EGTA, 5 mM MgSO4.7H2O, and 1 mM ATP, pH 7.4 at 4°C) was used. The final mitochondrial suspension was immediately used for measurement of mitochondrial respiratory parameters, and the remaining preparation later stored at −80°C for production of protein lysates for immunoblotting.
Measurement of mitochondrial respiratory parameters
Respiratory rates of rat heart mitochondria were measured using a Clark-type oxygen electrode (Strathkelvin Instruments Ltd, Glasgow, UK). Respiration rates were recorded and quantified using 782 Oxygen System v3.0 software (Strathkelvin Instruments). Oxygen concentrations were measured in 0.5 ml respiration medium (100 mM KCl, 50 mM MOPS, 1.0 mM KH2PO4, 1.0 mg/ml defatted BSA, pH 7.4) in two respiration chambers maintained at 30°C containing magnetic stirrers [25]. The chambers were treated identically throughout the experiment, except that one contained 1 μmol GDP (dissolved in 10 μl of H2O, pH 7.4), added to the respiratory medium at the start of the experiment (i.e. before mitochondria were added) and allowed to mix. GDP is an inhibitor of the mitochondrial uncoupling proteins [53], such that differences between the two chambers indicate the influence of UCP3 on oxygen utilisation. Oxygen concentrations were first recorded in the absence of mitochondria, and after the addition of approximately 0.5 mg mitochondrial protein, assayed using a protein assay kit (Pierce, Rockford IL, USA). Glutamate (20 mM), malate (5 mM) plus pyruvate (0.5 mM) or malate (5 mM) plus palmitoyl carnitine (0.04 mM), were added as substrates, before State III respiration was stimulated by the addition of 100 nmol of ADP. State IV respiration, which occurred when all the ADP added to the respiration medium had been phosphorylated, was subsequently measured. State III and state IV measurements were repeated after addition of a further 100 nmol of ADP. Acceptor control ratios (ACRs) were calculated as an indication of mitochondrial integrity by dividing state III respiration rates by state IV respiration rates. ADP/O ratios (the ratios of molecules of ADP phosphorylated for each oxygen atom consumed) were calculated as described by Estabrook [16]. ADP/O ratios are independent of the rates of oxygen consumption and give a more sensitive and precise measure of mitochondrial respiratory coupling than can be inferred from respiration rates alone.
Enzyme analysis
Whole snap-frozen heart tissue was crushed under liquid nitrogen and activities of the mitochondrial β-oxidation enzyme MCAD and citrate synthase, a measure of mitochondrial content, were measured using methods described previously [20].
Immunoblotting
Levels of cardiac UCP3 protein were measured in mitochondrial preparations by immunoblotting, as described previously [30], using a polyclonal rabbit anti-UCP3 antibody (Alpha Diagnostic International, San Antonio, TX) at a concentration of 1:1,000 in 5% milk TBS–Tween. MTE-1 protein levels were measured in mitochondrial preparations as described previously [26], using a polyclonal rabbit anti-MTE-1 antibody (gift of Dr Stefan Alexson, Karolinska Institutet, Stockholm, Sweden) at a concentration of 1:2,000 in 5% milk TBS–Tween. Levels of ANT protein were determined using a polyclonal goat anti-ANT antibody (Santa Cruz Biotechnology, CA, USA) as described previously [29]. Levels of F0F1 ATP synthase α-subunit protein were determined using a polyclonal mouse anti-α subunit F0F1 ATP synthase antibody (Mitosciences Inc, Eugene, USA) at a concentration of 1:2,000 in 5% milk TBS–Tween. Total cellular GLUT 4 protein content was determined using a monoclonal anti-COOH-terminal GLUT4 antibody (kind gift, G.D. Holman, Bath), as described previously [34]. The secondary antibody used, in all cases, was the appropriate horseradish peroxidase conjugate polyclonal antibody (Autogen Bioclear, Wiltshire, UK) at a concentration of 1:3,500 in 5% milk TBS–Tween. Even protein loading and transfer were confirmed by Ponceau staining, and protein levels were related to internal standards to ensure homogeneity between samples and gels. Bands were quantified using UN-SCAN-IT gel software (Silk Scientific, USA), and all samples were run in duplicate on separate gels to confirm results.
To measure oxidative modification of mitochondrial proteins, protein carbonylation was measured using an OxyBlot kit (Millipore, MA, USA). In brief, carbonyl groups were derivatised to 2,4-dinitrophenylhydrazone (DNP) by 2,4-dinitrophenylhydrazine, and proteins were then separated on a gel, using western blotting techniques described above. Blots were incubated with a DNP-specific antibody contained in the kit, and the sum of the separated protein bands was quantified as total protein carbonylation in the sample.
Blood plasma analyses
At the point of heart excision, blood was taken from the chest cavity, rapidly centrifuged at 1,000×g at 4°C, and the plasma frozen at −80°C for biochemical analysis. Lipoprotein lipase inhibitor (2%) was added to an aliquot of plasma for non-esterified FFA analysis. Plasma levels of glucose, triglycerides, total cholesterol, HDL cholesterol and LDL cholesterol were measured using an ABX Pentra Clinical Chemistry bench-top analyser (Horiba ABX, Montpellier, France). Plasma levels of FFAs were measured using an NEFA assay kit (Wako Chemicals GmbH, Neuss, Germany).
Statistics
Grouped data (mean ± SEM) were analysed using two-way ANOVAs. Data involving time or a change in perfusion substrate were analysed using a mixed design ANOVA with repeated measures, using Bonferroni’s correction where appropriate. Correlations were tested for significance using Pearson’s product moment statistics. The results were considered significantly different at p < 0.05.
Results
Morphological parameters and in vivo cardiac function
All rats consumed a similar weight of food each day (Table 1); however, the high fat diet had a greater caloric density than the standard laboratory chow, so the high fat-fed rats consumed 86% more calories than chow-fed controls (p < 0.0001). The greater calorie consumption resulted in the high fat-fed rats having 12% greater body weights than controls after 3 weeks (p < 0.05). Correspondingly, the hearts of the high fat-fed rats were 10% heavier than those from controls (p < 0.05), and in proportion to the increase in body weight, as all rats had the same heart/body weight ratios. High fat-fed rats had greater adiposity, as suggested by 67% larger epididymal fat pads (p < 0.01). In vivo ejection fractions, stroke volumes, heart rates and cardiac outputs were the same for all rats (Table 1).
Table 1
Daily dietary intake, morphological parameters, cardiac function and blood plasma metabolites from control (chow-fed) and high fat-fed rats
Control (n = 6) | High fat-fed (n = 6) | |
---|---|---|
Dietary intake | ||
Food intake (g/day) | 17.5 ± 1.7 | 20 ± 0.8 |
Calorie intake (kcal/day) | 58 ± 6 | 102 ± 4**** |
Morphological data | ||
Body weight (g) | 415 ± 13 | 466 ± 17* |
Heart weight (g) | 0.98 ± 0.03 | 1.08 ± 0.03* |
HW/BW × 1,000 | 2.38 ± 0.05 | 2.34 ± 0.06 |
Epididymal fat weight (g) | 11.4 ± 0.9 | 19.0 ± 1.8** |
In vivo cardiac MRI | ||
Left ventricle mass (g) | 0.45 ± 0.03 | 0.49 ± 0.04 |
Septal wall thickness (mm) | 1.70 ± 0.06 | 1.75 ± 0.1 |
Ejection fraction (%) | 77 ± 1 | 74 ± 1 |
End-diastolic volume | 306 ± 23 | 307 ± 20 |
End-systolic volume | 70 ± 6 | 79 ± 7 |
Stroke volume (μl) | 236 ± 17 | 228 ± 16 |
Heart rate (bpm) | 442 ± 9 | 473 ± 27 |
Cardiac output (ml.min−1) | 104 ± 8 | 108 ± 10 |
Fasting plasma metabolites | ||
Glucose (mM) | 9.5 ± 0.7 | 11.2 ± 1.1 |
Triglycerides (mM) | 1.6 ± 0.1 | 1.9 ± 0.2 |
Free-fatty acids (mM) | 0.25 ± 0.03 | 0.38 ± 0.04* |
Total cholesterol (mM) | 1.6 ± 0.1 | 2.0 ± 0.1* |
LDL cholesterol (mM) | 0.04 ± 0.01 | 0.19 ± 0.02**** |
HDL cholesterol (mM) | 0.65 ± 0.04 | 0.88 ± 0.11 |
* p < 0.05, ** p < 0.01, **** p < 0.0001 versus controls (HW/BW = heart weight/body weight)
Fasting blood plasma metabolites
All rats had similar fasting plasma levels of glucose and triglycerides (Table 1). Fasting plasma levels of free-fatty acids were elevated by 52% to 0.38 mM in the high fat-fed rats when compared with 0.25 mM in the control rats (p < 0.05). Total blood cholesterol levels were 25% higher in the high fat-fed rats at 2 mM when compared with 1.6 mM in controls (p < 0.05), with an increased LDL cholesterol fraction of 0.19 mM when compared with 0.04 mM in controls (p < 0.0001), and no difference in the HDL cholesterol fraction.
Isolated working heart
Consistent with in vivo measurement of cardiac function, aortic flows were the same for all rat hearts at all palmitate concentrations (Fig. 2). Coronary flows were 15% greater in high fat-fed rat hearts under all perfusion conditions (p < 0.04), but cardiac outputs were the same for all hearts. Therefore, high fat feeding had no significant effect on cardiac function, whether measured in vivo (Table 1) or in the isolated heart (Fig. 2).
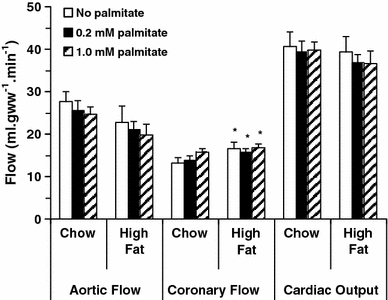
Isolated working heart aortic flows, coronary flows and cardiac outputs (left to right) following 3 weeks of high fat feeding. Hearts were perfused with three buffers, all of which contained 11 mM glucose, 0.2 mM pyruvate and 0.5 mM lactate as substrates (clear bars), and the addition of 0.2 mM palmitate (shaded bars), or 1.0 mM palmitate (hatched bars). *p < 0.05 versus chow fed
Oxygen consumption in chow-fed rat hearts was significantly increased on perfusion with 1 mM palmitate, when compared with perfusion with no palmitate, or with 0.2 mM palmitate (Fig. 3, p < 0.02). Overall, oxygen consumption in high fat-fed rat hearts was 19% higher than chow-fed rat hearts (p < 0.01), but was independent of palmitate concentration. Consistent with measurements of in vivo function, cardiac power in high fat-fed rat hearts (8.8 ± 0.7 mW) was the same as chow-fed rat hearts (9.4 ± 0.6 mW) under all perfusion conditions. Cardiac efficiency was higher in chow-fed rat hearts when perfused without palmitate than with either 0.2 mM (p < 0.01) or 1.0 mM palmitate (p < 0.02). Cardiac efficiency was on average 21% lower in high fat-fed rat hearts than in chow-fed rat hearts (Fig. 3), as a consequence of the increased oxygen consumption. High fat-fed rat hearts had 43% lower efficiency as compared to chow-fed rat hearts when perfused without palmitate (p < 0.01), and 28% lower efficiency when perfused with 1.0 mM palmitate (p < 0.05).
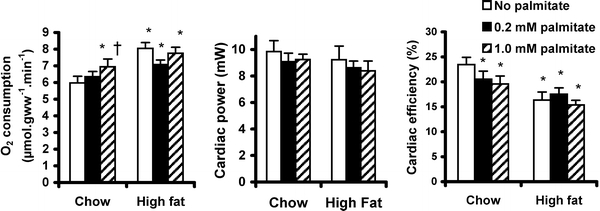
Oxygen consumption (left), cardiac power (middle) and cardiac efficiency (right) in isolated rat hearts following high fat feeding. Hearts were perfused with three buffers, all of which contained 11 mM glucose, 0.2 mM pyruvate and 0.5 mM lactate as substrates (clear bars), and the addition of 0.2 mM palmitate (shaded bars), or 1.0 mM palmitate (hatched bars). *p < 0.05 versus chow-fed rat hearts perfused without palmitate, † p < 0.05 versus chow-fed rat hearts perfused with 0.2 mM palmitate
Respiration in isolated mitochondria
State 3 respiration rates were higher in isolated cardiac mitochondria from high fat-fed rats than chow-fed rats (p < 0.05) in the presence of palmitoyl carnitine and malate (Fig. 4), but were unchanged with pyruvate and malate or glutamate as substrates. The addition of the UCP3 inhibitor, GDP, did not significantly change state 3 respiration rates. With all substrates, state 4 respiration rates (Fig. 4) were not significantly different in cardiac mitochondria from high fat-fed when compared with chow-fed animals, and were unchanged with the addition of GDP. ADP/O ratios were lower in cardiac mitochondria from high fat-fed rats as compared to those from chow-fed animals, irrespective of substrate (p < 0.01). The addition of GDP did not alter ADP/O ratios of mitochondria from chow-fed rat hearts (Fig. 4), but normalised the ADP/O ratio in high fat-fed rat heart mitochondria (p < 0.01) with all substrates.
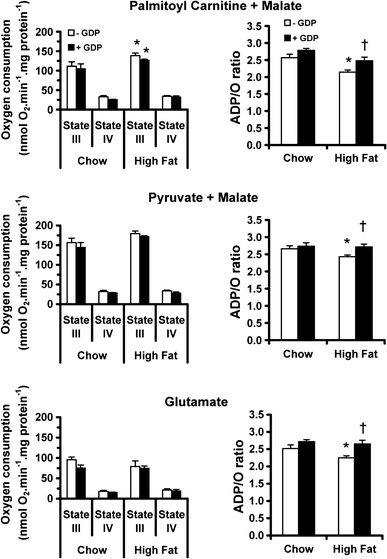
State 3 respiration rates and ADP/O ratios in rat left ventricular mitochondria with palmitoyl-carnitine plus malate (upper), pyruvate plus malate (middle) or glutamate (lower) as substrates. In addition to the control condition (clear bars), mitochondria were incubated with the UCP3 inhibitor GDP (shaded bars). *p < 0.05 versus chow fed, † p < 0.05 versus high fat-fed without GDP
MCAD and citrate synthase activity and GLUT 4 protein expression
Cardiac MCAD activity (Fig. 5) was 23% higher in high fat-fed rat hearts (p < 0.05). Citrate synthase activity was the same for all hearts (119 ± 4 μmol gww−1 min−1 after chow feeding and 124 ± 6 μmol gww−1 min−1 after high fat feeding), indicating higher cardiac β-oxidation in high fat-fed animals with unchanged cardiac mitochondrial content. MCAD activity correlated positively (p < 0.001) with oxygen consumption in hearts perfused with 0.2 mM palmitate (Fig. 5, p < 0.001). Total cardiac protein levels of the insulin-sensitive glucose transporter, GLUT 4, were unchanged with high fat feeding (1.0 ± 0.06 after chow feeding, 0.93 ± 0.05 after high fat feeding).
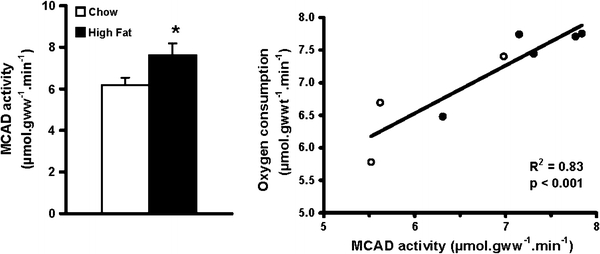
Cardiac medium-chain acyl-CoA dehydrogenase (MCAD) activity (left) in chow-fed (clear bars) and high fat-fed rats (shaded bars) *p < 0.05 versus chow fed. Correlation between cardiac MCAD activity and oxygen consumption (right) in isolated perfused working heart from chow (open symbols) and high fat-fed rats (closed symbols)
Mitochondrial protein expression and modification
UCP 3 and MTE-1 protein levels (Fig. 6) were both increased by 20% in high fat-fed rat hearts (p < 0.05). Cardiac ANT and F0F1 ATP synthase protein levels did not differ with high fat feeding (Fig. 6), supporting the finding that mitochondrial content had not changed with the high fat diet. Levels of protein carbonylation were the same in all cardiac mitochondria.
Discussion
In this study, we investigated cardiac function, efficiency and mitochondrial respiration in a rat model of diet-induced obesity in the absence of overt diabetes. A high fat diet increased body and epididymal fat pad weights, signifying greater body adiposity. Fasting plasma FFA levels were 52% higher in the high fat-fed animals, with increased total and LDL cholesterol. The high fat-fed rats were neither, however, hyperglycaemic in the fasting state, nor was cardiac GLUT 4 content decreased suggesting that cardiac glucose metabolism was normal in this short-term high fat feeding model. We found that isolated, perfused hearts from high fat-fed rats had decreased efficiency. The decreased cardiac efficiency following high fat feeding was solely due to increased oxygen consumption, and we suggest that this was caused by increased fatty acid oxidation and mitochondrial uncoupling.
Cardiac insulin resistance
We set out in this study to examine cardiac metabolism in a pre-diabetic model of early dietary-induced obesity, so there is a possibility that animals were insulin resistant after 3 weeks of high fat feeding. 4 weeks of feeding a 34% fat diet elevated plasma insulin [17], and 6–8 weeks of a 60% fat diet produced systemic glucose intolerance [12, 42]. However, cardiac insulin sensitivity was normal after 8 weeks of feeding a 60% fat diet, a diet similar to that in the present study [12]. The heart appears to maintain a normal insulin response, despite systemic glucose intolerance, for a protracted period. For example, the obese Zucker rat had not developed cardiac insulin resistance by 6 months of age, and only had mild cardiac insulin resistance at 12 months [44]. Therefore, short-term high fat feeding has only a low likelihood of inducing cardiac insulin resistance.
Cardiac function and efficiency
Mechanical factors may have contributed to increased cardiac oxygen consumption in our rat hearts. Increased myocardial oxygen consumption has been closely linked to increased left ventricular wall stress [24, 46], however in vivo MRI measurements of cardiac function and morphology showed no abnormalities in end-diastolic volume or wall thickness in our high fat-fed rat hearts. In another study [43], high fat feeding increased systolic blood pressure by ~40%, which would increase wall stress, but afterload and hydraulic resistance were constant in our isolated working heart preparation, so the effects of blood pressure were not relevant. These results indicated to us that the increase in oxygen consumption was due to changes in cardiac metabolism, rather than mechanical factors. High fat-fed rats had normal cardiac function when measured in vivo or when hearts were perfused in working mode, and thus it seems that ATP synthesis was maintained. However, oxygen consumption was increased, with the result that cardiac efficiency was decreased in high fat-fed rat hearts.
Fatty acid oxidation
We found that high fat-fed rat hearts had increased mitochondrial fatty acid oxidation when compared with control rat hearts. Mitochondria from high fat-fed rat hearts had increased state 3 respiration only in the presence of palmitoyl-carnitine, whereas respiration was normal with pyruvate or glutamate. It is likely, therefore, that the increased respiration rate was associated with the upregulation of enzymes of fat metabolism, such as MCAD (Fig. 1), which increases in heart following PPARα activation [19]. MCAD activity was elevated by 23% in high fat-fed rat hearts, similar to the increase in mitochondrial fatty acid oxidation, but citrate synthase activity and protein levels of F0F1 ATP synthase and ANT were unaltered, therefore an increase in the number of mitochondria could not account for these differences.
Our finding of increased fatty acid oxidation and MCAD activity is consistent with long-term high fat feeding studies [32, 37]. Increased fatty acid oxidation has been reported in isolated, perfused working rat hearts after 4 weeks of feeding a 60% saturated fat diet, but this was not associated with decreased cardiac efficiency [51]. Fatty acid oxidation is unaltered in rats bred for low aerobic capacity with moderate obesity [41]. In the present study, a switch in substrate preference away from more oxygen efficient glucose oxidation, towards fatty acid oxidation with high fat feeding [5] may have contributed to an increase in oxygen consumption and decrease in cardiac efficiency. This effect would be relatively minor, as the maximum difference in oxygen consumption between glucose oxidation alone to palmitate oxidation alone is 10–12% [5, 49]. Therefore, increased fatty acid oxidation would have played a minor role in decreasing cardiac efficiency with high fat feeding.
High FFAs increased oxygen consumption by up to 40% in either unloaded or arrested isolated hearts on perfusion with >1 mM fatty acids [2, 11], and in vivo infusion of 10% intra-lipid increased oxygen consumption by 26% [28]. In our study, there was a 20% increase in oxygen consumption on perfusion with 1 mM fatty acids, and in proportion to the exogenous fatty acid supply (Fig. 3). The mechanisms responsible for such increases in oxygen consumption in the normal heart remain unclear, but may be related to direct action of FFAs on mitochondrial uncoupling [36]. We found that mitochondrial ADP/O ratios from chow-fed rat hearts were not affected by GDP, indicating that uncoupling proteins were not responsible for the increased oxygen consumption in hearts from chow-fed animals. However, this does not discount the possibility that direct action of FFAs cause uncoupling [36] in the absence of changes in mitochondrial proteins. In contrast to chow-fed rat hearts, high fat-fed rat hearts had significantly elevated oxygen consumption even when no exogenous FFA was present, with reduced cardiac efficiency, which cannot be explained by the mechanisms described above [2, 36, 38]. This, therefore, points to abnormalities in oxidative metabolism downstream of substrate supply (Fig. 1).
Mitochondrial respiration and UCP protein expression
The finding that oxygen consumption in the high fat-fed isolated heart was increased may be explained by differences in mitochondrial metabolism. Evidence from both mitochondrial respiration and protein expression indicate that mitochondria of high fat-fed hearts were more uncoupled than chow-fed hearts. In earlier work, we found that decreased efficiency in the hyperthyroid or chronically infarcted rat heart was associated with increased mitochondrial UCP3 protein levels and increased uncoupling [3, 30]. In high fat-fed rats, increased UCP3 levels were associated with decreased phosphorylation/oxidation coupling of interfibrillar mitochondria with all substrates tested (Fig. 1), which may explain why cardiac oxygen consumption in high fat-fed isolated hearts was increased irrespective of perfusion substrate. In isolated high fat-fed rat cardiac mitochondria, inhibition of UCP3 increased phosphorylation efficiency to control levels. Cardiac levels of UCP3 are elevated in severe rodent models of type 2 diabetes [31], type 1 diabetes [31], hyperthyroidism [3] and heart failure [30], all of which are characterised by elevated plasma FFA levels. However, increased cardiac UCP3 levels in disease models have not always been found [4, 9, 10], despite increased proton leak in isolated cardiac mitochondria [4, 22]. Here, we found that diet-induced elevation of plasma FFAs was sufficient to elevate cardiac levels of both UCP3 and MTE-1 by 20%. UCP3 and MTE-1 are upregulated in heart via activation of PPARα [18, 45], and it has been suggested that the two proteins might act in concert to export fatty acid anions from the mitochondrial matrix as a protective mechanism [21] or to increase fatty acid oxidation [45]. High fat feeding did not, however, alter cardiac levels of ANT, another putative mitochondrial uncoupler of rat heart mitochondria [7, 40], or the α-subunit of the F0F1 ATP synthase. Therefore, mitochondrial uncoupling, not increased numbers of mitochondria, may explain the increased oxygen consumption and decreased efficiency of the high fat-fed rat heart.
A positive consequence of an increase in UCP3 protein and uncoupling of mitochondrial respiration may be that cardiac ROS production from the electron transport chain is reduced [6, 14] as the electrochemical gradient driving the electron transport chain becomes dissipated. Several studies report reduced ROS production with an increase in UCP3 protein [13, 47], a relationship supported by the reverse observation, in that decreased cardiac UCP3 protein is accompanied by increased ROS production [8]. We found no evidence of mitochondrial oxidative protein modification with high fat feeding, indicating that ROS-mediated damage was unaltered. More prolonged high fat feeding may be required to produce oxidative protein modification; others have shown that although total myocardial protein carbonylation was unaltered after 4 weeks, it had increased after 16 weeks of feeding a 60% fat diet [51]. Thus, the duration of high fat feeding was probably insufficient for the increased UCP3 proteins to have a measureable effect on ROS generation and oxidative protein modification.
Conclusion
In conclusion, we have demonstrated that high fat diet-induced obesity, in the absence of overt diabetes, increased mitochondrial UCP3 levels and the capacity for fatty acid oxidation in cardiac mitochondria, resulting in mitochondrial uncoupling. In the absence of changes in cardiac function, oxygen consumption increased, thereby decreasing cardiac efficiency. Thus, mitochondrial uncoupling may underlie the cardiac energetic abnormalities observed in diabetic patients prior to the development of functional impairment [39].
Acknowledgments
We thank Emma Carter for her invaluable technical assistance, and Dr Charles L Hoppel and his research group for their generous help in teaching us their mitochondrial isolation and respiration protocols. Stefan Alexson is thanked for his generous gift of the MTE-1 antibody. Sara McAleese thanks the Wellcome Trust for her Undergraduate Vacation Studentship. This study was supported by the British Heart Foundation, grant number RG/02/009/13631.
Conflict of interest
The authors declare that they have no conflict of interest.
Open Access
This article is distributed under the terms of the Creative Commons Attribution Noncommercial License which permits any noncommercial use, distribution, and reproduction in any medium, provided the original author(s) and source are credited.
Abbreviations
UCP | Uncoupling protein |
FFA | Free-fatty acids |
PPAR | Peroxisome proliferator activated receptor |
MRI | Magnetic resonance imaging |
MTE-1 | Mitochondrial thioesterase-1 |
ANT | Adenine nucleotide translocase |
MCAD | Medium chain acyl-CoA dehydrogenase |
ACR | Acceptor control ratio |
gww | Gram wet weight |
BSA | Bovine serum albumin |
References
Full text links
Read article at publisher's site: https://doi.org/10.1007/s00395-011-0156-1
Read article for free, from open access legal sources, via Unpaywall:
https://link.springer.com/content/pdf/10.1007%2Fs00395-011-0156-1.pdf
Citations & impact
Impact metrics
Citations of article over time
Alternative metrics
Smart citations by scite.ai
Explore citation contexts and check if this article has been
supported or disputed.
https://scite.ai/reports/10.1007/s00395-011-0156-1
Article citations
Haplotype variability in mitochondrial rRNA predisposes to metabolic syndrome.
Commun Biol, 7(1):1116, 11 Sep 2024
Cited by: 0 articles | PMID: 39261587 | PMCID: PMC11391015
SCD4 deficiency decreases cardiac steatosis and prevents cardiac remodeling in mice fed a high-fat diet.
J Lipid Res, 65(9):100612, 31 Jul 2024
Cited by: 0 articles | PMID: 39094772 | PMCID: PMC11402454
The role of acetylation in obesity-induced cardiac metabolic alterations.
J Pharm Pharm Sci, 27:13080, 23 Jul 2024
Cited by: 0 articles | PMID: 39109269 | PMCID: PMC11300292
Review Free full text in Europe PMC
Obesity-Related Atrial Fibrillation: Cardiac Manifestation of a Systemic Disease.
J Cardiovasc Dev Dis, 10(8):323, 30 Jul 2023
Cited by: 0 articles | PMID: 37623336 | PMCID: PMC10455513
Review Free full text in Europe PMC
High-fat diet causes mitochondrial damage and downregulation of mitofusin-2 and optic atrophy-1 in multiple organs.
J Clin Biochem Nutr, 73(1):61-76, 25 May 2023
Cited by: 5 articles | PMID: 37534099 | PMCID: PMC10390808
Go to all (106) article citations
Data
Similar Articles
To arrive at the top five similar articles we use a word-weighted algorithm to compare words from the Title and Abstract of each citation.
UCP3 regulates cardiac efficiency and mitochondrial coupling in high fat-fed mice but not in leptin-deficient mice.
Diabetes, 61(12):3260-3269, 21 Aug 2012
Cited by: 38 articles | PMID: 22912419 | PMCID: PMC3501860
Metabolic adaptation follows contractile dysfunction in the heart of obese Zucker rats fed a high-fat "Western" diet.
Obesity (Silver Spring), 18(10):1895-1901, 28 Jan 2010
Cited by: 20 articles | PMID: 20111021 | PMCID: PMC3623948
Increased mitochondrial uncoupling proteins, respiratory uncoupling and decreased efficiency in the chronically infarcted rat heart.
J Mol Cell Cardiol, 44(4):694-700, 09 Feb 2008
Cited by: 80 articles | PMID: 18328500
Physiological role of UCP3 may be export of fatty acids from mitochondria when fatty acid oxidation predominates: an hypothesis.
Exp Biol Med (Maywood), 226(2):78-84, 01 Feb 2001
Cited by: 149 articles | PMID: 11446442
Review
Funding
Funders who supported this work.
British Heart Foundation (3)
Substrates, transcription and control of cardiac energy metabolism and function
Kieran Clarke, University of Oxford
Grant ID: RG/07/004/22659
Cardiovascular physiological biochemistry
Kieran Clarke, University of Oxford
Grant ID: PS/02/002/14893
Metabolic control of cardiac energetics and efficiency: glucose, free fatty acids, nuclear peroxisome proliferator-activated receptors and mitochondrial uncoupling proteins
Kieran Clarke
Grant ID: RG/02/009/13631