Abstract
Free full text

Kinetics and Phospholipid Specificity of Apolipoprotein N-Acyltransferase
Abstract
The enzyme apolipoprotein N-acyltransferase (Lnt) is an integral membrane protein that catalyzes the last step in the post-translational modification of bacterial lipoproteins. Lnt undergoes covalent modification in the presence of phospholipids resulting in a thioester acyl-enzyme intermediate. It then transfers the acyl chain to the α-amino group of the N-terminal diacylglyceryl-modified cysteine of apolipoprotein, leading to the formation of mature triacylated lipoprotein. To gain insight into the catalytic mechanism of this two-step reaction, we overproduced and purified the enzyme of Escherichia coli and studied its N-acyltransferase activity using a novel in vitro assay. The purified enzyme was fully active, as judged by its ability to form a stable thioester acyl-enzyme intermediate and N-acylate the apo-form of the murein lipoprotein Lpp in vitro. Incorporation of [3H]palmitate and mass spectrometry analysis demonstrated that Lnt recognized the synthetic diacylglyceryl-modified lipopeptide FSL-1 as a substrate in a mixed micelle assay. Kinetics of Lnt using phosphatidylethanolamine as an acyl donor and FSL-1 as a substrate were consistent with a ping-pong type mechanism, demonstrating slow acyl-enzyme intermediate formation and rapid N-acyl transfer to the apolipopeptide in vitro. In contrast to earlier in vitro observations, the N-acyltransferase activity was strongly affected by the phospholipid headgroup and acyl chain composition.
Introduction
The post-translational modification of proteins by covalent lipid attachment is universal to all organisms. In bacteria, 1–3% of the genome encodes lipoproteins that are anchored to the cell envelope, where they fulfill various key functions, such as maintenance of the cell wall integrity, nutrient uptake, secretion, extra-cytoplasmic protein folding, and virulence (1–7). Lipoproteins are synthesized as prolipoproteins that are translocated across the cytoplasmic membrane via the Sec or the Twin arginine translocation (Tat) machineries (8–10). With few exceptions (11), all of them have a hydrophobic signal peptide that includes a highly conserved amino acid motif, called the lipobox (Leu(Ala, Val)−4-Leu−3-Ala(Ser)−2-Gly(Ala)−1-Cys+1). The invariant cysteine is fatty-acylated by the sequential action of phosphatidylglycerol:prolipoprotein diacylglyceryl transferase (Lgt), which adds a phosphatidylglycerol-derived thioether-linked diacylglyceryl residue, and of prolipoprotein signal peptidase (Lsp), which cleaves the hydrophobic signal peptide to liberate the α-amino group of Cys+1. In a final step, apolipoprotein-N-acyltransferase (Lnt) transfers a preferential phosphatidylethanolamine-derived sn-1-acyl chain to the free α-amino group of Cys+1, resulting in mature tri-acylated lipoprotein (Fig. 1) (12–15). All three enzymes are essential for growth and viability of Gram-negative bacteria and are located in the inner (cytoplasmic) membrane (16–18). In proteobacteria, N-acylation of lipoproteins is a prerequisite for transfer of lipoproteins to the outer membrane via the Lol machinery (17, 19, 20). The gene encoding Lnt was originally identified in Salmonella enterica sv. Typhimurium and is conserved in proteobacteria and in mycobacteria (21, 22). Recent findings demonstrated that N-acylated lipoproteins exist in various staphylococcal species, suggesting that apolipoprotein N-acyltransferase activity is present in firmicutes, although an lnt gene could not be identified in the genomes of this class of bacteria (23, 24).
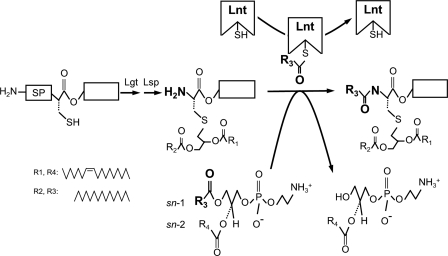
Lipoprotein modification pathway in E. coli. An unmodified prolipoprotein with a hydrophobic signal peptide (SP) containing the conserved lipobox sequence is first modified by the enzymes phosphatidylglycerol:prolipoprotein diacylglyceryl transferase (Lgt) and prolipoprotein signal peptidase (Lsp). This apolipoprotein is further modified by apolipoprotein N-acyltransferase (Lnt), which attacks the sn-1 acyl chain of a phospholipid, forms a thioester acyl-enzyme intermediate, and transfers the acyl group to the α-amino group of the diacylglyceryl-cysteine, leading to a mature triacylated lipoprotein. The acyl donor indicated is based on one of the major E. coli phospholipids, 1-palmitoyl-2-vaccenoyl-sn-glycero-3-phosphoethanolamine (16:0, cis-Δ11,12-18:1).
Initial studies of Escherichia coli Lnt assigned its first enzymatic properties, e.g. a pH optimum of 6.5–7.4, thermostability up to 60 °C, and activity dependence upon the presence of detergents (14). More recently, it was demonstrated that Lnt is anchored in the inner membrane by at least six transmembrane helices with a nitrilase-like periplasmic domain containing the catalytic triad Glu267–Lys335–Cys387 (17, 22). The formation of an extra-cytoplasmic thioester acyl-enzyme intermediate suggested that Lnt functions by an initial nucleophilic attack of the activated thiol of Cys387 on the carbonyl group of the sn-1-acyl chain of a phospholipid like phosphatidylethanolamine (PE)3 (25). The by-product of this first step, a lysophospholipid (Fig. 1), is flipped across the inner membrane by the lysophospholipid transporter LplT and is recycled by the bifunctional enzyme 2-acyl-PE acyltransferase/acyl-ACP synthetase (26, 27). In the second step of the Lnt reaction, the acyl-enzyme intermediate is resolved by the α-amino group of an incoming apolipoprotein. Mycobacterial lipoproteins are modified with a diacylglyceryl moiety containing mycobacterium-specific fatty acids (21). The observation that Lnt of Mycobacterium smegmatis is nonfunctional in E. coli suggests that the diacylglyceryl group also plays a role in substrate specificity. It is currently not known how Lnt binds its substrates or how it removes the acyl group from phospholipids and subsequently attaches it onto a lipoprotein. We developed an apolipoprotein N-acyltransferase in vitro assay based on purified enzyme, a synthetic lipopeptide substrate and various phospholipid acyl donors. We present its kinetic parameters and determined specificity of fatty acid chains and phospholipid headgroups of apolipoprotein N-acyltransferase.
EXPERIMENTAL PROCEDURES
Chemicals and Materials
[9,10-3H]Palmitate, 5 mCi (185 MBq), were purchased from PerkinElmer Life Sciences. The cysteine alkylation reagent mPEG-MAL (5 kDa) was obtained from Creative PEG Works, Winston Salem, NC. All phospholipids used in the study were from Avanti Polar Lipids, Alabaster, AL. The lipopeptide FSL-1-Biotin was obtained from EMC Microcollections, Tübingen, Germany. Plasmid pASK-IBA3+ anhydrotetracycline, Streptactin®-Sepharose, and desthiobiotin were purchased from IBA GmbH, Göttingen, Germany. The protease inhibitor 4-(2-aminoethyl) benzenesulfonyl fluoride hydrochloride was from Interchim SA, Montluçon, France. All molecular biology enzymes were either from New England Biolabs, Ipswich, MA, or from Fermentas GmbH, St. Leon Rot, Germany. All other chemicals, including oligonucleotides, were either obtained from Sigma or from Carl Roth GmbH, Karlsruhe, Germany, and were at least of analytical grade.
Bacterial Strains and Plasmids
All strains and plasmids used in the study are listed in Table 1. PAP105 was used as an E. coli strain in all experiments unless indicated otherwise. Plasmid pCHAP9510 was generated by cloning a synthetic DNA fragment (Sigma) encoding the myc tag amino acids in-frame with the Strep tag sequence of pASK-IBA3+ using NcoI and PstI restriction sites. The lnt gene from E. coli was PCR-amplified from genomic DNA using the oligonucleotides Lnt_20a_BsaI (5′-GGATAGGGTCTCAAATGGCTTTTGCCTCATTAATTG-3′) and Lnt_20b_BsaI (5′-CACGATGGTCTCGGCGCTTTTACGTCGCTGACGCAG-3′) as primers and cloned into the pASK-IBA3+ using BsaAI restriction sites. The Lnt(K335A) and the single cysteine Lnt(Cys387) variant were obtained by PCR using plasmids pCHAP7650 and pCHAP7556 as templates, respectively (17, 22). PCR fragments were cloned either in pASK-IBA3+ using BsaAI restriction sites (Lnt(K335A)) or in pCHAP9510 (Lnt(Cys387)) using EcoRI and XhoI restriction sites.
TABLE 1
Strains and plasmids used in the study
Strain/plasmid | Characteristics | Source or Ref. |
---|---|---|
E. coli strains | ||
![]() ![]() ![]() ![]() | E. coli K-12 Δ(lac-pro) F′ (lacIq ΔlacZM15 pro+Tn10) | Laboratory collection |
![]() ![]() ![]() ![]() | BW25113 ybeX-(kan-rpoCter-paraB)-lnt | 17 |
plasmids | ||
![]() ![]() ![]() ![]() | pUC18 lnt(C23A,C62A) | 25 |
![]() ![]() ![]() ![]() | pUC18 lnt(K335A) | 22 |
![]() ![]() ![]() ![]() | Expression vector with AHT inducible promoter, Strep-tag, AmpR | IBA GmbH |
![]() ![]() ![]() ![]() | pASK-IBA3+-lntEc-strep | This study |
![]() ![]() ![]() ![]() | pASK-IBA3+-lntEc(K335A)-strep | This study |
![]() ![]() ![]() ![]() | pASK-IBA3+-myc-strep | This study |
![]() ![]() ![]() ![]() | pASK-IBA3+-lntEc(C23A,C62A)-myc-strep | This study |
Growth Conditions for Overproduction of Lnt
Myc-Strep. E. coli PAP105 with plasmids pCHAP9515, pCHAP9519, or pCHAP9531 was grown in 10 ml of LB with 100 μg ml−1 ampicillin from a single colony at 37 °C overnight. This preculture was used to inoculate 1 liter of LB medium containing 0.1% glucose, and bacteria were initially grown at 30 °C under aeration at 180 rpm on a rotary shaker. At an A600 of 0.6–0.8, lnt expression was induced by the addition of anhydrotetracycline to 100 ng μl−1, and the culture was transferred to 25 °C, which allowed further growth, albeit at a drastically lowered rate. After 4–5 h and at an A600 of 1.3–1.6, cells were harvested by centrifugation and washed with buffer P1 (20 mm Tris-HCl, pH 8, containing 150 mm NaCl, and 0.5 mm EDTA). This buffer was used in all subsequent steps, and modifications are as indicated. The pellet was used directly for the preparation of membranes or stored up to 36 h at −25 °C.
Membrane Preparation and Solubilization of Lnt
All steps were carried out at 4 °C. The frozen pellet was resuspended in 25 ml of P1 with 0.1 μg ml−1 DNase and 0.2 mm 4-(2-aminoethyl)benzenesulfonyl fluoride hydrochloride. Cells were broken by three passages through a French pressure cell at 10,000 p.s.i. Unbroken cells and debris were removed by centrifugation at 6,000 × g for 10 min. The membrane fraction was prepared from the supernatant by ultracentrifugation at 120,000 × g for 60 min. The membrane pellets were either stored for up to 48 h at −25 °C or immediately washed and resuspended in 9 ml of P1. Triton X-100 was added to a final concentration of 2% (v/v) to solubilize membrane proteins, and the suspension was incubated for 1 h with intermittent inversion on a rotation apparatus. All insoluble material was removed by centrifugation at 40,000 × g for 30 min.
Protein Purification
Lnt was purified by Strep-Tactin® affinity chromatography using a prepacked Strep-Tactin® Superflow® high capacity column (IBA) coupled to an Äkta Purifier FPLC system (GE Healthcare). The column was equilibrated with 2 column volumes (CV) of P1 containing 2% Triton X-100. After binding 10 ml of the Triton X-100-solubilized membrane fraction, the column was washed with at least 4 CV of P1 containing 0.1% Triton X-100. A second wash with 4 CV of P1 containing 0.05% DDM replaced the Triton X-100 as observed by a decrease in UV absorbance 280 nm. The protein was eluted from the column with 3 CV of P1 containing 0.05% DDM and 2.5 mm desthiobiotin. Purity was judged after SDS-PAGE and staining with Coomassie Brilliant Blue, and the identity of the protein was confirmed by immunoblotting. The protein concentration was determined by measuring the absorbance at 280 nm and by the BCA method using BSA as a standard. Purified protein was stored in the elution buffer at −25 °C for at least 5 months without a detectable loss in activity.
Alkylation with Mal-PEG
The presence of free thiol groups in wild type Lnt and the single cysteine Lnt(C23A,C62A) was determined by their accessibility to the alkylating agent Maleimide-conjugated polyethylene glycol, 5 kDa (Mal-PEG) as described previously (25). Briefly, purified protein was incubated at 37 °C in the presence or absence of phospholipids for 5 min and denatured by adding of 0.5% SDS in 1 m Tris-HCl, pH 8. Mal-PEG was added to a final concentration of 0.2 mm and incubated for 30 min at room temperature. An excess of cysteine (2 mm) was added to terminate the reaction. As the Mal-PEG adds roughly 5 kDa to their molecular mass, alkylated proteins could be identified easily by SDS-PAGE followed by immunoblotting.
Preparation of Apo-Lpp
An lnt conditional null mutant expressing the lnt gene from an arabinose-inducible promoter was used to obtain the apo-form of Lpp, essentially as described earlier (17). Lnt was depleted when cells were grown in LB at 37 °C with d-fucose (0.2% (w/v)) to repress the arabinose promoter. After 8 generations (~180 min), growth arrest was observed, and the A600 remained constant. Cells were harvested 30 min after the growth arrest, which was accompanied by the accumulation of apolipoprotein. Appearance of the apo-form of Lpp was confirmed by immunoblotting using polyclonal anti-Lpp antibodies. Spheroplasts of these samples were used to isolate the membrane fraction that was either frozen or directly used to analyze Lnt activity.
Lnt in Vitro Assay with Unlabeled Phospholipids
When apo-Lpp was used as a substrate, the assay was carried out using the membrane fraction of Lnt-depleted cells as a source of acyl donor and apolipoprotein substrate. Membranes were added to a final protein concentration of 250 ng μl−1 to the standard reaction buffer composed of 50 mm Tris-HCl, pH 7.2, 150 mm NaCl, and 0.1% Triton X-100. A reaction mixture of 50 μl was briefly sonicated in a sonicator bath and preincubated at 37 °C prior to the addition of purified enzyme at 10 ng μl−1. Samples were removed at time intervals, and Lnt was rapidly inactivated by adding 5% SDS and heating to 100 °C. The relative levels of apo- and mature Lpp were monitored by SDS-PAGE and immunoblotting using anti-Lpp antibodies.
The standard assay for Lnt activity using the lipopeptide FSL-1 was carried out in a reaction volume of 20 μl containing commercial phospholipids and FSL-1 at concentrations of 500 and 5 μm, respectively. Phospholipids were added as mixed micelles in 0.1% Triton X-100. Following a brief sonication and preincubation at 37 °C, 3.5 nm (0.2 ng μl−1) of Lnt was added. To determine the initial rates of the Lnt reaction, samples were taken at least at four time points within the first 120 min of the reaction. The time course of the first (slow) step of the reaction was 0, 5, 10, and 20 min and of the second (fast) step was 0, 2, 5, and 10 min. Samples of 2 μl were removed at time intervals and heat-inactivated in the presence of 5% SDS. Aliquots of the samples were analyzed by SDS-PAGE and immunoblotting.
Preparation of [3H]Palmitate-labeled Membrane Vesicles
Radioactive labeling of whole cells was performed as described previously (25). Cells were grown at 37 °C to A600 0.2, and [3H]palmitate was added to 100 μCi ml−1. After 60 min, cells were harvested by centrifugation and washed with P1 buffer. The total membrane fraction was prepared from spheroplasts as described previously (28). The membranes were heated at 100 °C for 10 min to inactivate endogenous Lnt and stored as 50-μl aliquots at −25 °C.
Lnt Assay with [3H]Palmitate Membrane Vesicles
The assay was performed essentially as described for apo-Lpp, except that [3H]palmitate-labeled membrane vesicles at 200 nCi μl−1 were used as source of phospholipids, and the apolipoprotein substrate was 20 ng μl−1 of FSL-1-Biotin peptide. Following heat inactivation of Lnt, FSL-1 was purified by its biotin tag using the streptavidin mutein matrix (Roche Applied Science). Purification was carried out according to the manufacturer's instructions except that 0.1% of Triton X-100 was added to the wash and elution buffers. Elution was performed in three 100-μl fractions that were combined and added to 5 ml of scintillation liquid (GE Healthcare). Radioactivity incorporation into the peptide was determined by measuring [3H]palmitate with the Tri-Carb-2100-TR liquid scintillation analyzer (PerkinElmer Life Sciences) and was expressed as the percentage of total radioactivity in the reaction mixture.
SDS-PAGE, Immunoblotting, and Quantification
Purified Lnt was detected after Tris-glycine-SDS-PAGE (10% acrylamide) and immunoblotted using α-Myc, anti-Strep-tag II, and α-Lnt antibodies. Rabbit polyclonal antibodies against Lnt were raised using purified His-tagged periplasmic domain of the enzyme as antigen (Genscript). This polypeptide was produced in inclusion bodies in the cytoplasm and was purified by Co2+ affinity chromatography under denaturing conditions. To distinguish between the diacylglyceryl- and the N-acyldiacylglyceryl forms of Lpp or FSL-1, samples were separated in high resolution Tricine-buffered SDS-PAGE (29). Acrylamide was taken from a 40% (w/v) stock solution containing 2.1% of bisacrylamide as a cross-linker. The percentage of polyacrylamide was 6% in the stacking gel and 16.5% for the separating gel. To enhance the resolution of the different forms of the lipopeptide FSL-1, 6 m urea was added to the gels, and the 18.5% separating gel was overlaid by an 11% acrylamide spacer gel. Following electrophoresis, the proteins were blotted onto nitrocellulose membranes and hybridized against a streptavidin-peroxidase conjugate. ECL Plus Western blotting detection reagent (GE Healthcare) was used as HRP substrate. Fluorescent signals were detected with a Storm840 optical scanner (GE Healthcare) and quantified using ImageQuant 5.0 (GE Healthcare).
MALDI Measurements of FSL-1
MALDI analyses were performed to monitor in vitro N-acylation of the FSL-1 peptide by Lnt. 2,5-Dihydroxybenzoic acid (10 mg ml−1) was dissolved in 20% acetonitrile and 0.1% HCOOH. Samples were diluted into the matrix solution to reach a 10 μm concentration of FSL-1. Crystals were obtained using the dried droplet method with 1 μl of each sample. MALDI measurements were carried out on a Voyager-DE STR MALDI-TOF mass spectrometer in the positive reflectron mode (Applied Biosystems, Foster City. CA). External calibration was applied, and mass spectra were processed by Data Explorer software (Applied Biosystems). The expected mass of FSL-1 (C112H188N20O23S2) is 2245,3598.
RESULTS
Overproduction and Purification of Lnt from E. coli
The lnt (cutE) gene was modified to allow production of a C-terminal Myc-Strep-tag II fusion protein in E. coli that was then recovered from the membrane fraction. The protein was not released from the membrane in 2 m NaCl or 6 m urea, as expected for an integral membrane protein and indicating that overproduction of Lnt does not result in the accumulation of membrane-associated aggregates. The detergents CHAPS, n-octylpolyoxyethylene, β-octyl glucoside, dodecyl maltoside (DDM), and Triton X-100 were tested in concentrations up to 2% (w/v) to solubilize Lnt. Triton X-100 (2%) was found to be the most efficient of these detergents, as judged by immunoblotting of detergent-soluble and -insoluble membrane fractions. This is in agreement with earlier observations that apolipoprotein N-acyltransferase activity was detected in Triton X-100-solubilized E. coli membranes (14). The solubilized membrane fraction was used directly for purification of Lnt via Strep-Tactin® affinity chromatography. During this procedure, the concentration of Triton X-100 was either reduced to 0.1% or the detergent was completely exchanged with 0.05% DDM without affecting protein solubility or enzyme activity. On SDS-PAGE, purified Lnt-Myc-Strep-tag II migrated as a 54-kDa protein, slightly faster than its theoretical molecular mass of 61 kDa (Fig. 2A; Ref. 39). The identity of the fusion protein was confirmed by immunoblotting using antibodies against the periplasmic domain of Lnt and against the myc and strep tag (Fig. 2B) (data not shown). The purified enzyme preparations were stored in elution buffer at −25 °C for up to 5 months without significant loss of activity. Overall, this protocol yielded up to 1 mg of protein/liter of cell culture in a single-step purification.
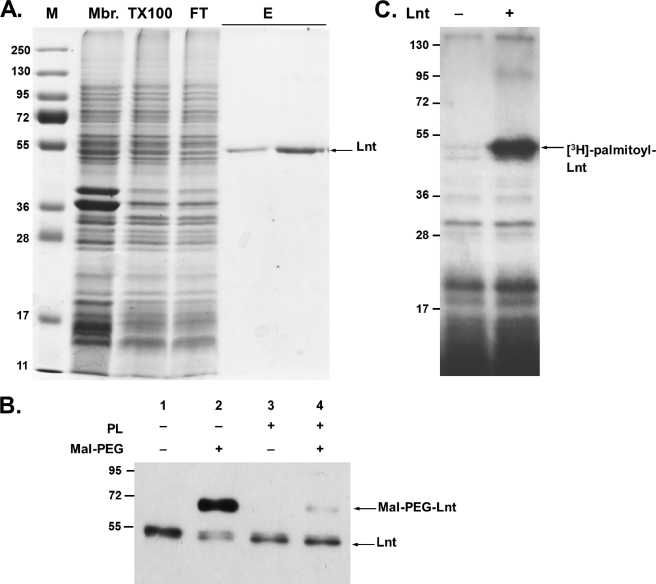
Purification of Lnt and formation of the acyl-enzyme intermediate in vitro. A, SDS-PAGE of protein fractions at different stages of Lnt purification. Proteins were stained with Coomassie Brilliant Blue and molecular mass standards (M) are given in kDa. Lnt was overproduced and purified from the membrane fraction (Mbr), solubilized in Triton X-100 (TX100), and bound specifically to a Strep-Tactin® column. After washing, purified Lnt was eluted from the column (E). FT indicates flow-through. See “Experimental Procedures” for details. B, alkylation of Lnt by Mal-PEG. Purified Lnt(C23A,C62A) was incubated in the absence (lanes 1 and 2) or presence (lanes 3 and 4) of phospholipids (PL) and subsequently treated with the thiol-specific alkylation reagent Mal-PEG (lanes 2 and 4). Lnt was detected by immunoblotting using α-Myc antibodies. C, purified Lnt forms a palmitoyl enzyme intermediate in vitro. Lnt was added to phospholipid vesicles isolated from [3H]palmitate-labeled cells and analyzed by SDS-PAGE and fluorography.
Purified Lnt Exists Mainly in the Nonacylated Form
Lnt exists as an extra-cytoplasmic acyl-enzyme intermediate in vivo because of the formation of a stable thioester between the active site cysteine and a palmitoyl residue, as demonstrated by [3H]palmitate labeling and by cysteine alkylation with Mal-PEG (25). To determine whether purified Lnt was in its acylated form, detergent-solubilized and delipidated enzyme was treated with Mal-PEG after incubation in the presence or absence of phospholipids. To facilitate analysis of the acylation state of Lnt, a variant in which cysteines at positions 23 and 62 were replaced by alanine was also purified. The variant enzyme Lnt(C23A,C62A) was previously shown to complement fully an lnt conditional null mutant (25) and to be fully active in vitro (see below). In the absence of phospholipids, Lnt was modified by Mal-PEG (5 kDa), resulting in a slower migrating band in SDS-PAGE (Fig. 2B, lane 2). In the presence of phospholipids, Lnt was protected from alkylation, indicating that the thioester blocked the thiol of the active site cysteine (Fig. 2B, lane 4). Further evidence for the absence of a stable thioester in the purified enzyme was provided by incubating it with [3H]palmitate phospholipids in labeled membrane vesicles. In this assay, Lnt formed a stable thioester acyl-enzyme intermediate that was detected by SDS-PAGE and fluorography (Fig. 2C). These results showed that purified Lnt is mainly unacylated but in an active conformation such that it can attack the sn-1-acyl chain of a phospholipid and become acylated.
Purified Lnt N-Acylates Endogenous Apo-Lpp in Vitro
Lpp is one of the most abundant proteins in E. coli, exceeding 500,000 molecules per cell (30, 31). Lnt catalyzes the N-acylation of apo-Lpp in vivo, and its correct lipid modification was shown to be essential for cell envelope integrity and for viability (17, 32). Hence, the lipidation intermediates of Lpp have often been used as model substrates to study lipoprotein modification in vitro and in vivo (17, 33, 34). To test whether the detergent-solubilized and -purified Lnt was active under in vitro conditions, we analyzed N-acylation of apo-Lpp in mixed detergent-phospholipid micelles. Apo-Lpp was prepared from a conditional lnt null mutant (17). In this strain, the lnt gene is exclusively expressed from an arabinose-inducible promoter. The absence of arabinose leads to depletion of Lnt and accumulation of apo-Lpp (17, 22). The apo-form can be distinguished from the mature (triacylated) form by its faster migration in a high resolution Tricine-SDS-PAGE (32). A mixture of Lpp species corresponding to the mature form, the accumulated apo-form, and Lpp linked to peptides of the peptidoglycan backbone was detected in vesicles prepared from spheroplasts of Lnt-depleted cells (Fig. 3A, 0 min). Upon incubation of Lnt with detergent-solubilized vesicles, apo-Lpp was converted over time into mature Lpp, as indicated by the disappearance of the apo-Lpp (Fig. 3A, 0–45 min). These data indicate that Lnt N-acylated endogenous apolipoprotein in vitro.
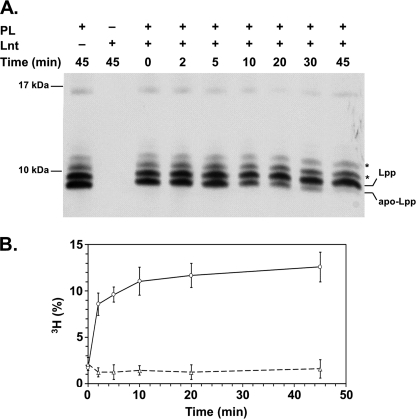
Purified Lnt is functional in vitro. A, Lnt N-acylates apo-Lpp in vitro. Purified Lnt was incubated with detergent-solubilized membrane vesicles derived from Lnt-depleted cells containing apo-Lpp as substrate and phospholipids (PL) as acyl donors. Samples were taken at different time points and analyzed by Tricine-SDS-PAGE and immunoblotting using anti-Lpp antibodies. Asterisks indicate Lpp covalently cross-linked to peptidoglycan fragments. B, Lnt-dependent [3H]palmitoyl incorporation in FSL-1. The diacylglyceryl-lipopeptide FSL-1 was mixed with phospholipid vesicles isolated from [3H]palmitate-labeled cells. Following the addition of Lnt (○) or inactive Lnt(K335A) (), the reaction mixture was incubated at 37 °C, and samples were removed at time intervals. The peptide was purified via streptavidin-biotin affinity, and [3H]palmitate incorporation was determined by scintillation counting. The amount of [3H]palmitate incorporated into the peptide is expressed as a percentage of the total amount of radioactivity in the reaction mixture from three independent experiments.
Lnt N-Acylates a Synthetic Diacylglyceryl Lipopeptide in Vitro
Initial attempts to study the kinetics of the apolipoprotein N-acyltransferase were hindered by the fact that defined substrates were unavailable and that entire membranes were used as the sources of the enzyme. To overcome this problem, we analyzed whether a synthetic diacylated lipopeptide could be used as a substrate to study Lnt activity. FSL-1-Biotin (FSL-1) is a synthetic peptide based on a lipoprotein from Mycoplasma pneumoniae and has often been used as model peptide to study the activation of Toll-like receptors (35, 36). This peptide carries a thioether-linked dipalmitoyl-glyceryl modification on its N-terminal cysteine residue and contains a free α-amino group, resembling perfectly the N terminus of an apolipoprotein. When FSL-1 was incubated with Lnt and [3H]palmitate-labeled heat-inactivated vesicles in the presence of detergent, more than 10% of total radioactivity was specifically incorporated into FSL-1 (Fig. 3B). The incorporation of [3H]palmitate in FSL-1 was strictly dependent on active Lnt, because [3H]palmitoyl transfer to FSL-1 was not observed when using an inactive Lnt variant in which Lys335 was replaced by Ala (Fig. 3B, dashed line) (22). These data suggested that FSL-1 was N-acylated by Lnt. To confirm that palmitate was attached to FSL-1, an Lnt-dependent reaction containing sn-1-(16:0)-2-(18:1)-PE was analyzed by MALDI-TOF mass spectrometry. The m/z 2246.4 peak corresponding to FSL-1 (average mass accuracy = 30 ppm) was detected both in the absence and in the presence of Lnt. In contrast, the m/z 2484.7 peak was only observed after completion of the reaction with Lnt (Fig. 4). The mass shift of 238.3 between the two peaks demonstrated that Lnt catalyzed the N-palmitoylation of FSL-1 (expected mass of palmitoyl moiety = 238.4 Da). Similar results were obtained by electrospray ionization-MS analyses (data not shown). These data provided direct evidence that the FSL-1 lipopeptide is recognized as an Lnt substrate in vitro and also confirmed that the sn-1-acyl chain (C16:0, 238.4 Da) and not the sn-2-chain (C18:1 and 264.4 Da) is transferred from the phospholipid to the N terminus of apolipoprotein.
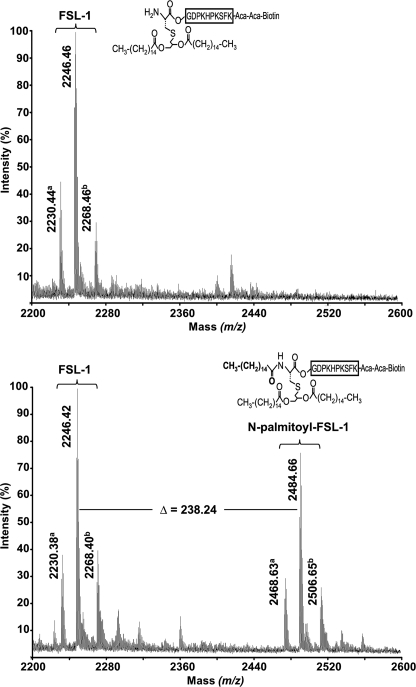
In vitro N-palmitoylation of FSL-1 by Lnt. Comparison of MALDI mass spectra of FSL-1 after the N-acylation reaction in the absence (upper panel) and in the presence (lower panel) of Lnt. The m/z 2200–2600 region of the MALDI spectra is shown, and only monoisotopic significant peaks (S/N ratio >-3) are annotated. Y axis represents the relative signal intensity. a peaks correspond most probably to a secondary product of the FSL-1 synthesis (m/z = 2230.38) and its N-palmitoylated form (m/z = 2468.63). b peaks are sodium adducts of FSL-1 and N-palmitoyl-FSL-1 peptides.
Enzymatic Properties of Lnt
The use of FSL-1 and chemically defined phospholipids together with purified Lnt facilitated studies on the substrate and acyl donor specificity and allowed the determination of kinetic parameters. Time-dependent N-acylation using polar lipid extract from E. coli (Fig. 5A) or synthetic phospholipids as acyl donors was analyzed on high resolution Tricine-SDS-PAGE followed by blotting onto nitrocellulose membranes and detection of biotinylated FSL-1 using a streptavidin-peroxidase conjugate. In the absence of enzyme or phospholipids, FSL-1 migrated aberrantly with an apparent size of ~5 kDa, despite its theoretical molecular mass of 2246 Da that was confirmed by MALDI and electrospray ionization-MS. Addition of Lnt resulted in a time-dependent appearance of a slightly slower migrating variant of the peptide, corresponding to the N-acylated form of FSL-1 (Fig. 5A). The quantification of the ratio between diacylated and triacylated FSL-1 over time revealed a typical progress curve of an enzymatic reaction in which almost all FSL-1 was converted to the N-acylated form (Fig. 5B). The enzyme was fully dependent on the presence of detergent. Triton X-100 was reported earlier to be the optimal detergent for the conservation of Lnt activity in membrane preparations of E. coli (33). When the standard concentration of 0.5 mm sn-1-(16:0)-2-(18:1)-PE was used as an acyl donor in the assay, the highest enzymatic activity was seen at Triton X-100 concentrations reaching from 0.05 to 0.1% (0.8–1.6 mm) (Fig. 5C). Triton X-100 at 0.1% exceeded the critical micelle concentration by a factor of 6–8 leading to a detergent-phospholipid ratio of 3:1 and was maintained as the standard detergent concentration in all assays.
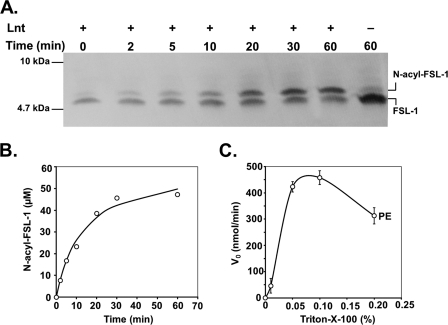
Lnt N-acylation activity with FSL-1. A, Lnt (10 nm) was added to a reaction mixture composed of polar lipid extract from E. coli (500 μm) and FSL-1 (60 μm). Samples were removed at time intervals from the reaction and analyzed by Tricine-SDS-PAGE and immunoblotting against the biotin tag of FSL-1. B, concentration of N-acylated FSL-1 in the reaction is represented as a function of time. C, initial rates of N-acylation activity under standard assay conditions (see “Experimental Procedures”) were strictly dependent on the presence of detergent and are displayed as function of the concentration of Triton X-100.
The existence of an acyl-enzyme intermediate in vivo (Fig. 2B) (25) and in vitro (Fig. 2C) suggested a two-step reaction via a “ping-pong” mechanism, where the initial attack of the phospholipid induces the formation of the intermediate (ping) and is followed by the N-acyl transfer to the apolipoprotein (pong). To verify that kinetics were consistent with this type of mechanism, the acyl donor concentrations were varied with different constant concentrations of FSL-1. When plotting the reciprocal of V0 versus the reciprocal of the sn-1-(16:0)-2-(18:1)-PE concentration, parallel lines were obtained (Fig. 6). These results show that the N-acyl transfer catalyzed by Lnt proceeds via ping-pong mechanism.
Kinetics and Polar Headgroup Specificity
Previous in vivo studies on the site-specific turnover of PE and, more recently, acyl transfer from phospholipids carrying acyl chains selectively labeled with [14C]palmitate or [3H]palmitate demonstrated that the sn-1-acyl chain is the primary acyl donor for Lnt (15, 19). This was further confirmed here by mass spectrometry. The mechanism, however, by which Lnt recognizes phospholipids as acyl donor is still unknown. It has been proposed that Lnt does not show any preference for a particular type of phospholipid with respect to headgroup structure or acyl chain length and composition. This proposal was based on the observation that PE, the major phospholipid in E. coli, was not essential for the N-acylation of lipoproteins in vivo and that all three phospholipids of E. coli (PE, PG, and cardiolipin) could serve as acyl donors in vitro (14, 37). The broad specificity of Lnt was further supported by a nonkinetic analysis using purified Lnt (19).
The in vitro Lnt assay using FSL-1 as an acceptor peptide allowed us to determine N-acylation kinetics in the presence of defined phospholipids. In a first approach, phospholipid-dependent recognition was analyzed by using pure PE, PG, PC, phosphatidylserine, or PA, all possessing identical diacylglyceride structures with palmitoyl (C16:0) and oleoyl (C18:1) residues as acyl chains in the sn-1 and sn-2 position, respectively, representing one of the most common acyl chain combinations in E. coli (38). As a result of the different biophysical properties of each of the phospholipids, we initially tested which phospholipid concentrations supported optimal enzyme activity under standard assay conditions (0.1% Triton X-100, 5 μm FSL-1, and 3.5 nm Lnt). PE and PG showed similar profiles with highest enzyme activities determined at a concentration of 500 μm (Fig. 7A). At this concentration, relatively low activity was seen with PA compared with its narrow optimum around 100 μm. Overall, N-acyltransferase activity was only observed with four different phospholipids under standard assay conditions (Fig. 7B). PE was most effective with a Km value for FSL-1 of 8.0 μm. Accordingly, the Vmax value was determined as 350 μmolFSL-1 min−1 μmol−1Lnt, corresponding to a specific activity of 5.6 ± 0.3 μmolFSL-1 min−1 mg−1Lnt. PG and PA also served as acyl donors, albeit at reduced rates. Their Km and Vmax values were 10.4 μm and 127 μmolFSL-1 min−1 μmol−1Lnt for PG and 3.8 μm and 106 μmolFSL-1 min−1 μmol−1Lnt for PA, respectively. The catalytic efficiencies of the enzyme in the presence of these three phospholipids were similar with kcat/Km values ranging in the same order of magnitude with 7.3 × 105 for PE, 4.8 × 105 for PA, and 2.0 × 105 for PG. Only trace activity was observed when using PC (Km:,18.2 μm; Vmax, 27 μmolFSL-1 min−1 μmol−1Lnt; and kcat/Km, 9.2 × 102 m−1 s−1). For phosphatidylserine, enzyme activity was below detection limits (data not shown). Variations in the length and composition of the acyl chains on PC did not increase FSL-1 N-acylation. This result provided additional evidence that the polar headgroup plays a crucial role in recognition by Lnt and that differences in micelle-phospholipid structure did not account for reduced activity.
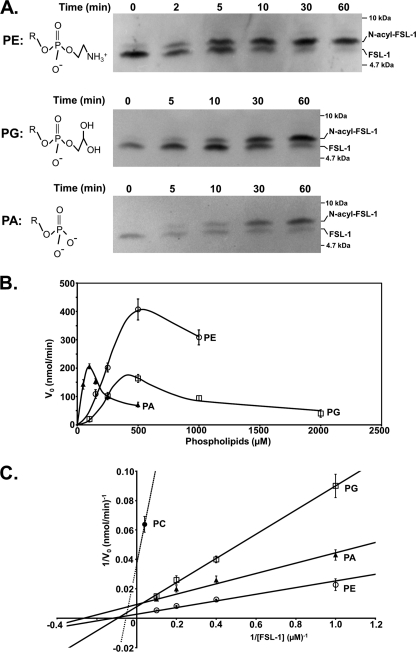
Kinetics and phospholipid headgroup specificity of Lnt. A, time-dependent formation of N-acyl-FSL-1 using PE (500 μm), PG (500 μm), and PA (100 μm) as acyl donors was analyzed by Tricine-SDS-PAGE and immunoblotting. Structures of the headgroups of PE, PA, and PG are displayed with R indicating the 1-palmitoyl-2-oleoyl-sn-glyceryl group. B, initial Lnt activities are displayed as function of the phospholipid concentration using PE (○), PA (), and PG (□). C, double-reciprocal plot showing initial Lnt activities with FSL-1 at various concentrations (1–5 μm) using either PE, PG, PC (500 μm, dashed line), or PA (100 μm) as acyl donor. Data points represent averages of three independent experiments.
Acyl Chain Specificity
In a second approach, we studied the effect of the acyl chain length and composition on Lnt activity by monitoring the formation of the thioester acyl-enzyme intermediate. After incubation with standard PE carrying sn-1-(16:0) and sn-2-(18:1) acyl chains, Lnt was largely inaccessible to alkylation by Mal-PEG (Fig. 8A, 3rd lane). This result suggested that formation of the acyl-enzyme thioester intermediate was most efficient with this phospholipid, in agreement with optimal enzyme activity observed under these conditions (Figs. 7B and and88B). Similar results were obtained when PE with acyl chains shorter than 16 carbons or with two unsaturated C16 acyl chains were used (Fig. 8A, 4th and 6th lanes). Acyl-enzyme intermediate formation did not occur when sn-1,2-(16:0)-PE, sn-1,2-(18:0)-PE, or branched hydrocarbon chains like archaeal type phytanyl residues (sn-1,2-(16:0;4ME)-PE) were used (Fig. 8A, 5th, 7th, and 8th lanes). To analyze acyl chain-dependent phospholipid recognition by Lnt in more detail, we used the kinetic assay with various concentrations for each type of PE (Fig. 8B). The ability to form a thioester acyl-enzyme intermediate with different types of PE was reflected by their kinetics. Highest activity relative to standard PE (sn-1-(16:0)-sn-2-(18:1), kcat/Km: 4.8 × 103 m−1 s−1, Km: 462 μm, kcat: 2.2) was observed with PE carrying a saturated C18 on sn-1 and an unsaturated C18 on sn-2 (sn-1-(18:0)-sn-2-(18:1), kcat/Km, 2.0 × 103 m−1 s−1; Km, 2941 μm; kcat, 6.0). PE with sn-1,2-(12:0) or with sn-1,2-(16:1) acyl chains served as functional acyl donors albeit with reduced efficiencies with kcat/Km values of 8.5 × 102 and 8.9 × 102 m−1 s−1, respectively. The corresponding Km and kcat values were determined as 476 μm and 0.4 s−1 (12:0) and 363 μm and 0.3 s−1 (16:1), respectively. Furthermore, sn-1-(16:0) lysophosphatidic acid, which lacks an sn-2 acyl chain, did not serve as a donor. As expected, activity with sn-1,2-(16:0)-PE, sn-1,2-(18:0)-PE, and sn-1,2-(16:0;4ME)-PE was below detection limits (data not shown). These data indicate that also the nontransferred sn-2 acyl chain plays an important role in acyl donor recognition.
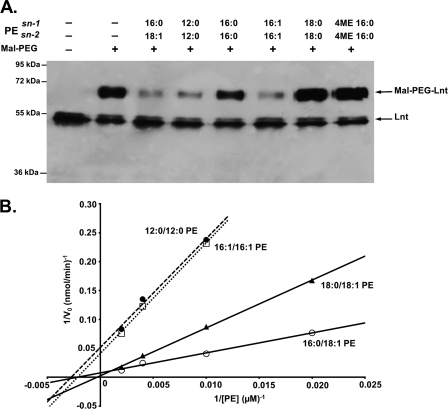
Phospholipid acyl chain specificity of Lnt. A, acyl-enzyme intermediate formation in the presence of PE with different acyl chain lengths and composition. Lnt(C23A,C62A) was incubated in the presence of phospholipids for 10 min and subsequently treated with the cysteine-specific alkylation reagent Mal-PEG. Samples were analyzed by SDS-PAGE and immunoblotting using α-Myc antibodies. B, double-reciprocal plot showing the initial N-acyltransferase activities of Lnt with 5 μm FSL-1 as an acceptor and PE with different acyl compositions as acyl donors (sn-1-(16:0)-sn-2-(18:1), ○; sn-1-(18:0)-sn-2-(18:1), ; sn-1-(16:1)-sn-2-(16:1), □; sn-1-(12:0)-sn-2-(12:0), ●) (40–500 μm).
DISCUSSION
The enzyme apolipoprotein N-acyltransferase (Lnt) catalyzes the phospholipid dependent N-acylation of bacterial lipoproteins, resulting in mature triacylated protein (14, 19). To gain deeper insight into this unique two-step reaction mechanism, we developed a quantitative in vitro assay that allowed us to determine kinetic parameters and acyl donor specificity. In cell extracts, Lnt exists mainly as a thioester acyl-enzyme intermediate with a stable thioester link between a fatty acid residue and the active site cysteine (22, 25). The purified enzyme was mainly nonacylated, as indicated by the fact that the active site thiol was accessible to alkylation by Mal-PEG. It is likely that the acyl-enzyme intermediate resolves during purification as a result of the lipid-detergent exchange. The purified enzyme could reform a stable thioester in the presence of phospholipids as demonstrated by [3H]palmitate labeling and cysteine inaccessibility to alkylation. Auto-acylation of Lnt and the formation of mature Lpp in vitro illustrated that Lnt remained in an overall active conformation upon purification and reconstitution in detergent micelles. We used the small dipalmitoylglyceryl-modified lipopeptide FSL-1 as a substrate to study the kinetics of N-acylation. This modification was sufficient for its recognition by Lnt, as shown by incorporation of [3H]palmitate and also demonstrated directly using mass spectrometry.
Lnt catalyzes a unique two-step reaction, the kinetics of which are in agreement with a ping-pong type mechanism. Kinetic parameters were determined for each of the two steps using standard PE (sn-1-(16:0)-sn-2-(18:1)) as acyl donor and FSL-1 as substrate. The determined kcat/Km value for the second step exceeded the one for the thermodynamically unfavorable first step of the reaction in which the oxygen ester-linked sn-1 acyl group of PE is transferred to the Cys387 thiol, by more than 2 orders of magnitude (4.8 × 103 for PE versus 7.3 × 105 for FSL-1). Lnt is thus highly efficient in the second step that involves binding of the apolipopeptide and N-acyl transfer. The fact that Lnt exists as a stable acyl-enzyme intermediate in vivo reflects the large differences in concentration and accessibility between the two substrates, phospholipids and apolipoprotein. The determined Vmax value for Lnt of E. coli led us to predict that 100–200 molecules of enzyme would be sufficient to achieve the N-acylation of all Lpp produced per cell (500,000) during one generation of growth suggesting that the enzyme is of low abundance but is highly efficient. Only few described membrane-bound acyltransferases use phospholipids as acyl donors. The lecithin-retinol acyltransferase transfers the sn-1-acyl chain from PC to retinol and, like Lnt, proceeds through an acyl-enzyme intermediate (40, 41). PC-dependent N-acyltransferase activity was reported for a very similar enzyme, the lecithin-retinol acyltransferase-like protein RLP-1, which is involved in the synthesis of precursors for bioactive N-acylethanolamines (42). The specific activities reported for both enzymes are at least 1 or 2 orders of magnitude lower than those determined for Lnt (42, 43). A prominent example of another membrane-bound bacterial acyltransferase is PagP. This enzyme is an integral outer membrane protein that transfers the sn-1-palmitoyl chain derived from PE to a lipid A molecule with 2 units of 3-deoxy-d-manno-2-octulosonic acid linked at the 6′ position (44). When sn-1-(16:0)-sn-2-(18:1)-PE was used as an acyl donor in the presence of Triton X-100, PagP catalyzed the O-acylation of lipid A at a rate of 7.22 μmol min−1 mg−1PagP (45). Using the same phospholipid as an acyl donor, similar kinetics (5.6 μmolFSL-1 min−1 mg−1Lnt) were determined for the N-acyltransferase activity of Lnt. Despite a completely different second step reaction, amide versus acyloxoacyl formation, both acyltransferases showed comparable enzymatic activities that are well reflected by the high abundance of their substrates, 5 × 105 for Lpp (30, 31) and 2 × 106 for lipid A (46).
Lnt was reported to accept a wide range of phospholipid acyl donors in vivo (15, 37). Here, we addressed its acyl donor specificity in more detail using the in vitro assay. PE was most efficient of the phospholipids tested in the N-acyltransferase reaction, which is not very surprising, because PE was reported to be the preferred acyl donor of Lnt in vivo (15). In exponentially growing E. coli cells, PE constitutes between 70 and 80% of cellular phospholipids, making it by far the most abundant component of the membrane (38). Both PG and PA supported apolipoprotein N-acyltransferase activity, although less efficiently than PE. PG is the second most abundant E. coli phospholipid (~20%), and a mutant lacking PE apparently N-acylated lipoproteins normally, providing evidence that PG can substitute for PE in vivo (37). PA, a minor component of E. coli membranes (~0.2%), is a key intermediate in phospholipid metabolism that accumulates in the absence of PG and is thought to compensate for it in the recruitment of peripheral membrane proteins (49). Phospholipids carrying small headgroups (PE and PG) are preferred over those with more bulky ones (phosphatidylserine and PC). Phospholipid recognition by Lnt was also dependent on the length and composition of the acyl chains. Enzyme activity and acyl enzyme formation was significant only when unbranched acyl chains in position sn-1 were accompanied by unsaturated ones in position sn-2, with the exception of short chain acyl groups (12:0). The length of the acyl chain in position sn-1 was less important as similar catalytic efficiencies were determined when either C16:0 or C18:0 at sn-1 was combined with C18:1 at sn-2. Fukuda et al. (19) showed that Lnt from E. coli has a broad specificity of fatty acyl chains using N-acylation of apo-Pal by Lnt in vitro. Phospholipids with varying chain lengths and degrees of saturation (C6:0 to C22:6) and different phospholipid headgroups were used, but the rate of N-acylation was not determined, which might explain their observation that all phospholipids serve equally well as substrates for Lnt.
Fatty acid specificity of acyltransferases is generally based on the presence of amino acid motifs that act as acyl chain binding pockets. These hydrocarbon rulers were identified as highly selective determinants for the recognition of the transferred sn-1 acyl chain by the phospholipid-dependent PagP and two soluble enzymes involved in lipid A metabolism, LpxA and LpxD (46–48). It is unlikely that Lnt employs a similar mechanism, as the enzyme seems to be less selective for the length of the sn-1 acyl chains. Although the role of the sn-2 position in phospholipid recognition is currently not clear, it has been reported for PagP that an oleate residue (C18:1) at this position was beneficial for enzyme activity (45). Future structural analysis of the enzyme will elucidate how the acyl chain is bound to Lnt.
Acknowledgments
We thank all members of the Molecular Genetics Unit for support and helpful discussions. We are grateful to Tony Pugsley, Nicolas Bayan, and Daniel Ladant for critical reading of the manuscript. We also thank Hajime Tokuda for antibodies against Lpp.
Footnotes
3The abbreviations used are:
- PE
- phosphatidylethanolamine
- PA
- phosphatidic acid
- PC
- phosphatidylcholine
- PG
- phosphatidylglycerol; lysophosphatidic acid
- DDM
- n-dodecyl β-d-maltoside
- Mal-PEG
- maleimide-polyethyleneglycol
- Tricine
- N-[2-hydroxy-1,1-bis(hydroxymethyl)ethyl]glycine
- CV
- column volume.
REFERENCES
Articles from The Journal of Biological Chemistry are provided here courtesy of American Society for Biochemistry and Molecular Biology
Full text links
Read article at publisher's site: https://doi.org/10.1074/jbc.m111.243519
Read article for free, from open access legal sources, via Unpaywall:
http://www.jbc.org/article/S002192582057511X/pdf
HAL Open Archive
https://hal.archives-ouvertes.fr/hal-00629168
Citations & impact
Impact metrics
Citations of article over time
Article citations
Fatty acids of Helicobacter pylori lipoproteins CagT and Lpp20.
Microbiol Spectr, 12(5):e0047024, 19 Mar 2024
Cited by: 0 articles | PMID: 38501821 | PMCID: PMC11064636
Structure snapshots reveal the mechanism of a bacterial membrane lipoprotein N-acyltransferase.
Sci Adv, 9(26):eadf5799, 30 Jun 2023
Cited by: 3 articles | PMID: 37390210 | PMCID: PMC10313180
A comparative analysis of lipoprotein transport proteins: LolA and LolB from Vibrio cholerae and LolA from Porphyromonas gingivalis.
Sci Rep, 13(1):6605, 24 Apr 2023
Cited by: 1 article | PMID: 37095149 | PMCID: PMC10126205
A Defect in Lipoprotein Modification by Lgt Leads to Abnormal Morphology and Cell Death in Escherichia coli That Is Independent of Major Lipoprotein Lpp.
J Bacteriol, 204(9):e0016422, 08 Aug 2022
Cited by: 4 articles | PMID: 35938851 | PMCID: PMC9487459
Recombinant and endogenous ways to produce methylated phospholipids in Escherichia coli.
Appl Microbiol Biotechnol, 105(23):8837-8851, 28 Oct 2021
Cited by: 4 articles | PMID: 34709431 | PMCID: PMC8590670
Go to all (35) article citations
Data
Similar Articles
To arrive at the top five similar articles we use a word-weighted algorithm to compare words from the Title and Abstract of each citation.
The essential Escherichia coli apolipoprotein N-acyltransferase (Lnt) exists as an extracytoplasmic thioester acyl-enzyme intermediate.
Biochemistry, 49(2):341-346, 01 Jan 2010
Cited by: 29 articles | PMID: 20000742 | PMCID: PMC3100165
Residues located on membrane-embedded flexible loops are essential for the second step of the apolipoprotein N-acyltransferase reaction.
Mol Microbiol, 95(4):692-705, 16 Jan 2015
Cited by: 15 articles | PMID: 25471278
Structural insights into lipoprotein N-acylation by Escherichia coli apolipoprotein N-acyltransferase.
Proc Natl Acad Sci U S A, 114(30):E6044-E6053, 11 Jul 2017
Cited by: 26 articles | PMID: 28698362 | PMCID: PMC5544336
The N-acyltransferase Lnt: Structure-function insights from recent simultaneous studies.
Int J Biol Macromol, 117:870-877, 31 May 2018
Cited by: 4 articles | PMID: 29859843
Review