Abstract
Free full text

Binding of Plasmodium merozoite proteins RON2 and AMA1 triggers commitment to invasion
Associated Data
Abstract
The commitment of Plasmodium merozoites to invade red blood cells (RBCs) is marked by the formation of a junction between the merozoite and the RBC and the coordinated induction of the parasitophorous vacuole. Despite its importance, the molecular events underlying the parasite’s commitment to invasion are not well understood. Here we show that the interaction of two parasite proteins, RON2 and AMA1, known to be critical for invasion, is essential to trigger junction formation. Using antibodies (Abs) that bind near the hydrophobic pocket of AMA1 and AMA1 mutated in the pocket, we identified RON2’s binding site on AMA1. Abs specific for the AMA1 pocket blocked junction formation and the induction of the parasitophorous vacuole. We also identified the critical residues in the RON2 peptide (previously shown to bind AMA1) that are required for binding to the AMA1 pocket, namely, two conserved, disulfide-linked cysteines. The RON2 peptide blocked junction formation but, unlike the AMA1-specific Ab, did not block formation of the parasitophorous vacuole, indicating that formation of the junction and parasitophorous vacuole are molecularly distinct steps in the invasion process. Collectively, these results identify the binding of RON2 to the hydrophobic pocket of AMA1 as the step that commits Plasmodium merozoites to RBC invasion and point to RON2 as a potential vaccine candidate.
Apicomplexa are a group of parasitic protozoa, including the human malaria parasite Plasmodium falciparum (Pf) and Toxoplasma gondii (Tg), that use apical secretory organelles to invade host cells. Invasion of Plasmodium spp. merozoites into erythrocytes begins with an initial weak attachment of the merozoite to the red blood cell (RBC) surface through yet-unidentified parasite receptor–RBC ligand interactions, followed by a reorientation that ultimately brings the apical end of the merozoite into close apposition with the RBC surface (1, 2). The merozoite then triggers the formation of a junction with the erythrocyte that by electron microscopy appears as a dense area below the erythrocyte membrane at the site of the merozoite’s apposed apical end. In addition, the merozoite secretes its rhoptry contents into the RBC that may facilitate the invasion of the merozoite (2–4). The merozoite subsequently moves through the junction as it pulls itself into the RBC through connections between parasite surface proteins and its actin–myosin motor (5). Hence, the formation of the junction and its connection with the molecular motor through the cytoplasmic tail of parasite receptors is critical for invasion (6, 7). Formation of the parasitophorous vacuole, created by the inward flow of the RBC membrane (8–10), occurs coordinately with the entry of the parasite into the RBC (4). At the end of invasion, the electron-dense junction becomes part of the parasitophorous vacuole that surrounds the newly invaded parasite (2). In cytochalasin-treated merozoites where actin polymerization is disrupted, the parasites apically reorient and induce both junction formation and invagination of the RBC membrane along the extent of the junction but are unable to invade (3). The cytochalasin-treated merozoites can also be observed to secrete the content of the rhoptries from their apical end into the erythrocytes that appear as vesicles in the RBC cytosol, structures that would form the parasitophorous vacuole during normal invasion (3, 4). These vesicles contain the known rhoptry bulb marker RAP1 (11), confirming their rhoptry origin. Understanding the molecular mechanisms by which the parasite induces junction formation is critical to understanding invasion.
Before junction formation, members of the parasite surface Duffy binding-like (DBL) and reticulocyte homology (RH) family ligands play an important role in invasion by interacting with RBC receptors and facilitating entry through multiple pathways (12, 13). Plasmodium knowlesi merozoites bind to human RBCs that lack the DBL receptor, the Duffy blood group antigen, and apically reorient but do not come into close apposition and do not form a junction (3). Plasmodium vivax invades squirrel monkey RBCs, although the single DBL ligand fails to bind squirrel monkey RBCs, indicating that invasion occurs through the P. vivax RH ligand. Furthermore, in Pf, either deletion of EBA175 or the selection of a sialic acid-independent pathway for invasion leads to the up-regulation of RH4 (12, 13). Hence, the DBL and RH family ligands appear to play a functionally redundant role in bringing the apically oriented merozoite in close apposition with the RBC membrane.
AMA1 has been shown to be essential for invasion (14–16), but its precise function in invasion is only beginning to emerge. AMA1, which is initially stored in the micronemes, is translocated to the parasite surface before the merozoite invades a new RBC (17). Crystal structures of AMA1 from Plasmodium spp. and Tg identified a hydrophobic pocket formed by two PAN domains (18–20). Monoclonal antibodies (mAbs) that bind near (mAb 4G2) or in (mAb 1F9) the PfAMA1 hydrophobic pocket interfere with merozoite invasion of RBCs (21–23). Studies in Tg and Pf showed that in parasite detergent extracts, AMA1 coimmunoprecipitated with a complex of proteins that are members of a family found in the rhoptry neck (RONs; refs. 24 and 25). The complex can include RON2, which is predicted to contain three hydrophobic helices; RON5, which contains a single predicted hydrophobic helix; and RON4 and RON8, which appear to be soluble proteins. RON proteins appear to be secreted into and across the RBC membrane and localize at the moving junction (26). Two recent studies identified a region in RON2 located between the second and third hydrophobic helices that binds to AMA1 and blocks invasion (27, 28).
Here we provide evidence that the binding of RON2 to AMA1 between the apically oriented merozoite and RBC is essential for triggering junction formation. We show that PfRON2 localizes to the moving junction along with PfRON4 and also confirm the presence of PfRON4 in the cytoplasmic side of the RBC. We find that the two conserved Cys residues present within the RON2 sequence between the second and third predicted hydrophobic helices, termed RON2L, are essential for AMA1 binding. We also show that mAbs 4G2 and 1F9, which bind near or in the hydrophobic pocket of AMA1, respectively, block binding of RON2 to AMA1. RON2 binding to AMA1 is blocked by a single mutation of Y251A in the hydrophobic pocket. Cytochalasin-treated merozoites bound to RBCs in the presence of mAb 4G2 or RON2L peptide reorient apically and come into close apposition at the apical end, but fail to trigger junction formation. These results identify the interaction of AMA1 and RON2 as essential for committing the merozoite to invasion by triggering junction formation.
Results
Pf AMA1, RON4, and RON2 Mark the Merozoite–RBC Moving Junction.
Using merozoites in the process of invasion, we show by confocal microscopy that PfRON2, PfRON4, and PfAMA1 colocalize at the moving junction (Fig. 1 A and B). The moving junction is demarcated by MSP1-33 (Fig. 1 A and B) that is present on the merozoite surface outside the RBC and is cleaved as the parasite enters the RBC (29). Earlier studies following P. knowlesi merozoite invasion of RBCs showed that the moving junction fuses after merozoite entry and becomes part of the newly formed parasitophorous vacuolar membrane (2). We found that for Pf merozoites, fusion of the junction at the posterior end of the merozoite is marked by PfRON4 (Fig. 1B Bottom).

Localization of Pf AMA1, RON2, and RON4 at the moving junction of invading merozoites. Confocal microscopy images of merozoite invading RBC are shown. (A) Pf AMA1, RON2, and RON4 colocalize during invasion. (B) AMA1 is present at the moving junction (Top) with RON4 colocalizing with AMA1 at the junction (Middle). RON4 marks the sealing of the junction after invasion (Bottom). Scale bars represent 1μM.
We used immuno-transmission electron microscopy to provide high-resolution localization of PfRON4, probing sections with mAb 24C6 specific for RON4. RON4 is initially located within the rhoptry neck in attached (Fig. S1A) and reoriented merozoites that are closely apposed against the RBC (Fig. S1B). Subsequently, RON4 is present in the merozoite and inside the RBC (Fig. S1C), indicating that RON4 is secreted into the RBC during an early step in invasion. In invading parasites, RON4 is located beneath the RBC membrane in the region of the junction (Fig. S1 D–H).
Two Conserved Cysteine Residues Located Within a Region of PfRON2 (RON2L) Are Required for Binding PfAMA1.
Recent studies by Tyler & Boothroyd (27) and Lamarque et al. (28) identified a conserved region of RON2, here termed “RON2L,” between two predicted hydrophobic helices that binds TgAMA1 and recombinant PfAMA1 (Fig. S2A). First, we confirm that PfRON2L binds recombinant PfAMA1 (rAMA1; ref. 30; Fig. S2 C and D). As a control, the scrambled peptide with the same amino acid composition failed to bind rAMA1 (Fig. S2 B and D). We also show that RON2L is sufficient to pull down the native parasite AMA1 from extracts of 35S-labeled schizont-infected RBCs (Fig. S2E), binding predominately the 83-kDa form of AMA1. The N-terminal region of RON2L is more conserved than the C-terminal region among Plasmodium spp. as well as between Plasmodium spp. and Tg, including two cysteines (Fig. S2A). We determined that the two completely conserved cysteine residues are critical for binding to AMA1, as replacing the two Cys with Ala in the peptide (RON2L C/A) or blocking the cysteine side chains in the peptide by alkylation (Fig. S2B) abrogated binding to AMA1 (Fig. S2D). This finding indicates that this region of RON2 may adopt a loop structure stabilized by the disulfide bond. A short 16-amino acid peptide consisting only the central region of RON2L that includes the two cysteines (RON2S in Fig. S2B) bound poorly to AMA1 (Fig. S2D). This result suggests that the AMA1 binding region may lie outside the two cysteines and that the disulfide bridge may be required for proper folding. Modeling the in silico folded RON2L peptide in complex with TgAMA1 (using the TgAMA1 crystal structure) suggests that the disulfide bonds may play a structural role for proper orientation of the binding region but may not itself interact with AMA1 (Figs. S3 and S4). Previous data showed failure of PfRON2L to bind recombinant PvAMA1 (28), indicating species specificity of the interaction. Because RON2L is conserved between Plasmodium species (Fig. S2A), we tested a homologous region from mouse Plasmodium yoelli RON2 (PyRON2L) for binding human PfAMA1 but did not observe significant binding (Fig. S2F), indicating evolutionary constraints within Plasmodium spp. for AMA1–RON2 interactions.
PfRON2 Binds the Hydrophobic Pocket of AMA1.
AMA1 contains a conserved hydrophobic pocket formed by two PAN domains (18–20). This pocket appears to be the site for RON2 binding, as the binding of RON2L to AMA1 was blocked by addition of mAbs that bound either in (mAb 1F9; ref. 19) or near (mAb 4G2; ref. 21) the hydrophobic pocket (Fig. 2A). Binding of RON2L to AMA1 was inhibited more effectively by mAb 1F9 than by mAb 4G2 (Fig. 2A). Preincubating AMA1 with mAb 1F9, but not mAb 4G2, further reduced RON2L binding (Fig. 2A). Remarkably, both mAbs 1F9 and 4G2 were able to release already bound RON2L (Fig. 2B). mAbs 1F9 and 4G2 have been shown to inhibit invasion, and this result suggests that invasion is not only blocked by interfering with the binding of AMA1 and RON2 but may also dislodge the bound complex. As the epitope recognized by mAb 4G2 lies adjacent to the hydrophobic pocket in the flexible domain II loop (21, 22), these data also suggest that the inhibitory activity of mAb 4G2 could be mediated by stabilizing the domain II loop in an unfavorable conformation for RON2 binding.
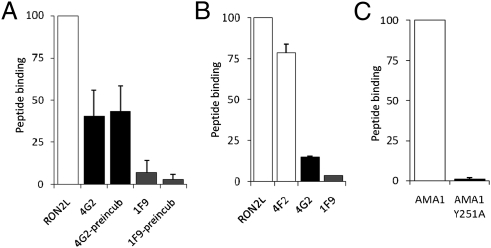
RON2L binds the hydrophobic pocket of AMA1. (A) RON2L binding to AMA1 is inhibited by mAbs 4G2 and 1F9 that bind near or in the hydrophobic pocket, respectively. (B) Prebound RON2L was displaced by AMA1 Abs. RON2L (20 pmol) was preincubated with recombinant AMA1 for 1 h before adding mAbs 4G2 (5 μg/mL), 4F2 (5 μg/mL), and 1F9 (2.5 μg/mL). (C) Mutating Tyr-251 to Ala (Y251A) in the hydrophobic pocket of AMA1 abolishes binding of RON2L. Results in A and B are pooled data from three independent experiments, and C is from two independent experiments. Data are represented as mean ± SEM.
Previously, Collins et al. (22) showed that a mutation in the hydrophobic pocket (Y251A) of AMA1 prevented the binding of AMA1 to a complex of RON proteins in vitro. However, the specific RON protein that binds this pocket was not identified. We expressed AMA1 with the central tyrosine in the pocket mutated to alanine (Y251A) and tested its ability to bind RON2L. This mutated AMA1 (Y251A) showed no RON2L binding (Fig. 2C). The Y251A mutation did not alter the folding of AMA1, as evidenced by the similar binding of conformation-specific mAbs 4G2 and 1F9 to both wild-type rAMA1 and rAMA1 (Y251A; Fig. S5D). These data indicate that RON2 binds the hydrophobic pocket of PfAMA1.
RON2L and Antibodies (Abs) to RON2L Block Invasion of Merozoites into Erythrocytes.
To determine whether the binding of RON2L to the pocket of AMA1 affects invasion, we monitored invasion of merozoites into RBCs in the presence of RON2L. We found that merozoite invasion of RBCs was blocked by 99% at 1.0 μM RON2L (Fig. S6 A and B). A scrambled peptide of RON2L as a control did not inhibit invasion (Fig. S6B). This result confirms the results of previous studies using recombinantly expressed RON2L fused to GST that showed inhibition of host cell invasion by Tg and Pf (27, 28), albeit at much higher efficiency.
We generated Abs to RON2L in rabbits and affinity purified the rabbit IgG on a RON2L column. The affinity purified RON2L-specific rabbit IgG blocked invasion of RBC by both Pf 3D7 and FVO clones (Fig. 3). The inhibition of invasion required 0.5–2 mg/mL RON2L-specific Ab. Because the Ab was only raised against the small AMA1 binding region of RON2, targeting the entire C-terminal region of RON2 may provide stronger inhibition because the peptide C-terminal to TgRON2L also binds TgAMA1 (27).
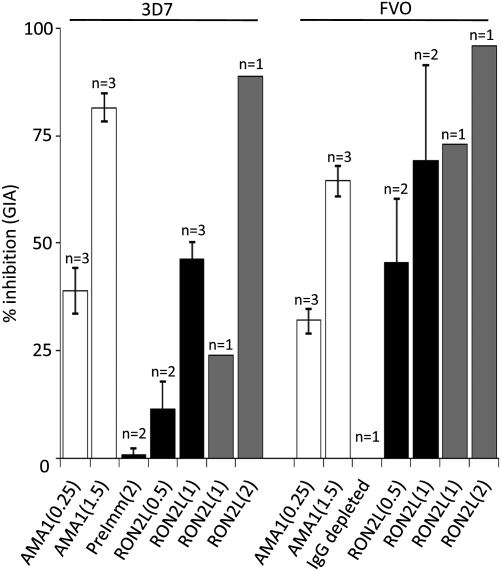
Abs to RON2L inhibit merozoite invasion of RBCs. Affinity purified anti-RON2L IgG from two rabbits (black and gray bars) inhibit merozoite invasion of 3D7 and FVO parasites as determined by standard growth inhibition assay. Anti-AMA1 rabbit IgG to a combination of FVO and 3D7 (white bars) was used as a positive control. Purified IgG from preimmune rabbit sera or sample from which RON2L IgG was depleted (using protein G) was used as negative control. Numbers in parentheses indicate the amount of IgG used (mg/mL) in this assay. Results shown are mean ± SEM from pooled data. Numbers above each bar (n) indicate the number of independent experiments.
Binding of RON2 to AMA1 in Cytochalasin-Treated Merozoites Triggers Junction Formation and Commitment to Invasion.
To dissect the function of AMA1–RON2 interaction during invasion, we determined the effect of mAb 4G2 and RON2L on cytochalasin-treated merozoites. As previously shown, in the presence of cytochalasin D that inhibits actin polymerization, merozoites attach to RBCs and apically reorient (Table S1 and Fig. S7). These merozoites come into close apposition with the RBC, form a junction, and induce vacuole formation within the RBC, indicating rhoptry secretion (Fig. 4A, b and c).

RON2 binding to AMA1 is required for triggering junction formation. (A) Transmission electron microscopy of merozoites treated with 2 μM cytochalasin D. (a–c) Merozoite attachment (a), reorientation and junction formation (b), and the presence of vacuoles (c), indicative of rhoptry bulb secretion. (d–i) The processes affected by the presence of invasion inhibitory mAb 4G2 (d–f) and RON2L peptide (g–i). Black arrows show the junction, and white arrows show the secreted vacuoles. R, rhoptry; M, microneme. (B) The percentage of merozoites in transmission electron microscopy that are attached to RBCs in each category and that are apical, not apical, and indeterminate. (C) The percentage of apically oriented merozoites in each category that form a junction and RBCs with vacuoles (indicative of rhoptry bulb secretion). Data shown for mAb 4G2 and RON2L peptide are pooled from two and three independent experiments, respectively. Numbers within each bar represent the number of merozoite-attached RBCs in each category. The number 0 indicates that no merozoites were found in that category. Scale bars represent 250 nm.
In the presence of mAb 4G2, the apical end of cytochalasin-treated merozoites came into close apposition with the RBC, indicating that the AMA1 interaction is not required for parasite attachment or reorientation (Table S1, Fig. 4B, and Fig. S7). However, the RBC membrane did not indent, no junction formed, and no vacuoles were observed in the RBC (Fig. 4A, e and f, and C). Thus, even merozoites in close apposition with the RBC could not form a junction without engagement of AMA1 with RON2. Tg in which AMA1 was depleted was defective in rhoptry bulb secretion, consistent with a role for AMA1 in this process (16). Close apical attachment to host cells in AMA1-depleted Tg was affected (16) and may reflect differences between Plasmodium and Toxoplasma.
Live video microscopy in the presence of mAb 4G2 showed that merozoites bind to RBCs and apically reorient but fall off the RBC without invading (Compare Movie S1 to Movie S2). The failure of merozoites to remain bound in the presence of mAb 4G2 is consistent with the failure to form a junction in the presence of the mAb (Fig. 4A). Earlier experiments using P. knowlesi merozoites and mAb specific for AMA1 (R31C2) in the absence of cytochalasin D suggested a role for AMA1 in mediating apical attachment (31). However, in the absence of cytochalasin D, the merozoite motor was continuously active, and as a result, merozoites detached from the RBCs because the junction was not formed (Movie S2). Contrary to the P. knowlesi study, our study shows normal apical reorientation in the presence of cytochalasin D and invasion inhibitory mAb 4G2.
In the presence of RON2L, merozoites reoriented apically (Table S1), there was no indentation of the RBC at the apical end of the merozoite, and no junction was formed (Fig. 4A, h and i). However, in the presence of RON2L, vacuoles formed in the RBC (Fig. 4A, i, and C). Together, these findings indicate that the AMA1–RON2 interaction is essential to trigger junction formation. These data also suggest that rhoptry secretion into the RBC occurs independent of junction formation.
Cytoplasmic Tail of AMA1 Binds Aldolase.
In the presence of cytochalasin, which disrupts actin polymerization, a junction formed, but the parasite could not invade (Fig. 4A, b and c; ref. 3). Similarly, in the absence of the junction (by preventing AMA1 binding to RON2), the merozoite could not invade despite a functional actin–myosin motor (Movie S2). Hence, merozoite entry into the RBC requires concurrent formation of the junction and linking the junction to the merozoite actin–myosin motor. Therefore, we tested whether the cytoplasmic tail of PfAMA1 could interact with aldolase, the bridge that connects the parasite surface ligands with the actin–myosin motor. We found that the cytoplasmic tail of AMA1 Fig. 5A, Wt) could bind rabbit muscle aldolase (Fig. 5B) and that this binding was competitively inhibited by the aldolase substrate, fructose-1,6-biphosphate (Fig. 5C). This finding suggests that the cytoplasmic tail of AMA1 interacts with the substrate binding pocket of aldolase, similar to TRAP (32). Phosphorylation of the Ser-610 in the AMA1 cytoplasmic tail has been shown to be required for merozoite invasion (33, 34). We found that the phosphorylation of S610 is not required for aldolase binding (Fig. 5A, Wt) and that mutating S610A does not affect binding (Fig. 5 B and C). This finding indicates that the phosphorylation of Ser-610 may have other roles in vivo such as signaling. However, mutating other potential aldolase binding residues to alanine (“All A” in Fig. 5A) abolished aldolase binding. To identify specific residues that are involved in binding, we mutated the two central Phe, Trp (F602A, W603A), or the two terminal Tyr (Y621A, Y622A) residues and found that they independently abolished binding, suggesting that both of these regions are required for efficient binding to aldolase (Fig. 5B). Mutating W603A did not completely inhibit aldolase binding (Fig. 5B). This result suggests that, unlike in TRAP (7), the Trp residue in PfAMA1 may not be the major aldolase binding site, but both FW and YY are required. Recent studies in Tg showed that the cytoplasmic tail of TgAMA1 (Fig. S8) bound rabbit muscle aldolase (35). The requirement of residues F519W520 (Fig. S8) for binding aldolase and for invasion in Tg suggests that this interaction also occurs in Pf (35). However, at least in Plasmodium, both FW and YY are equally important motifs for binding aldolase. Our data suggest that the cytoplasmic tail of PfAMA1 can bind aldolase and may provide a link to the merozoite motor for invasion.
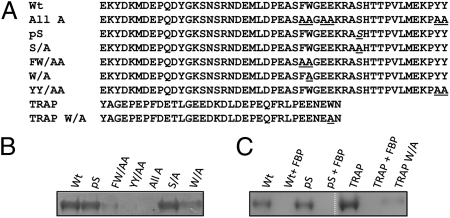
AMA1 cytoplasmic tail binds aldolase. (A) Sequence of the native cytoplasmic tail (Wt) of AMA1. Mutations analyzed in the current study are underlined. pS, phosphorylated Ser (S610). The sequence of Plasmodium TRAP and the subterminal Trp residue in TRAP mutated to Ala is also shown. (B) Identifying the critical residues in AMA1 cytoplasmic tail for aldolase binding. Wt AMA1 cytoplasmic tail binds recombinant rabbit muscle aldolase (lane 1). Ser-610 in the AMA1 cytoplasmic tail (lane 6) or its phosphorylation (lane 2) is not critical for binding, whereas the central Phe, Trp (F602W603), and the terminal Tyr (Y621 and Y622) are critical for aldolase binding (lanes 3 and 4). (C) Cytoplasmic tail of AMA1 interacts with the aldolase substrate binding pocket. Binding of aldolase to Wt and pS AMA1 cytoplasmic tails was inhibited by the aldolase substrate fructose-1,6-biphosphate (FBP; lanes 2 and 4). Binding of TRAP (lane 5) was also inhibited by FBP (lane 6) as well as by mutation of the subterminal Trp (lane 7). All of the lanes were from the same gel with the empty lane removed (white dotted line) for showing lanes side by side.
Discussion
The data presented here describe the molecular basis of junction formation during Pf invasion of RBCs in the blood stage of malaria infections. Plasmodium merozoites invade RBCs by a complex series of events that involve multiple, often redundant parasite–RBC interactions that initiate the cellular responses necessary for invasion. Our current understanding of the early events in the invasion process is depicted in Fig. 6. First, the Pf merozoite attaches to the target RBC through weak, poorly understood interactions between the parasite and the RBC. Second, the merozoite quickly reorients, and then, primarily through the function of two parasite ligand families, DBL and RH, the parasite is brought into close apposition with the RBC membrane (3). EBA-175, a parasite ligand, has been shown to be released by exposure to merozoites to low K+ environment (5 mM) or to the calcium ionophore A23187 (36). Third, parasite proteins from the neck of the rhoptries, RONs, are presumably inserted into and across the RBC membrane by unknown signals. Fourth, a junction forms between the merozoite and the RBC, defined by the electron-dense area below the RBC membrane. The junction is a critical structure that provides a firm anchor for the merozoite to pull its way into the RBC. Fifth, rhoptry bulb proteins that are presumably involved in the next stage of invasion, in particular, in the induction of the parasitophorous membrane as the parasite moves into the RBC, are injected into the RBC and are concentrated in vesicular-like structures observed in the RBC cytoplasm during cytochalasin-blocked invasion (present work and refs. 3 and 11). The parasitophorous membrane derives from the RBC membrane that flows past the junction (8–10, 37). Here we asked what merozoite–RBC interactions trigger formation of the junction. We focused on interactions between AMA1 and RON2 that were recently shown to play a role in invasion (27, 28). We show that a critical step in invasion—namely, junction formation—requires the interaction of AMA1 and RON2 (Fig. 6).
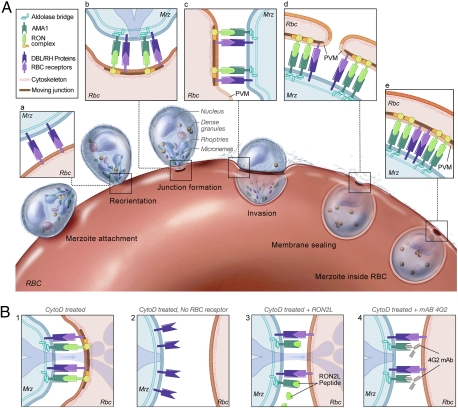
Schematic model of the steps involved in Pf merozoite (Mrz) invasion. (A) See text for details. (B) Cytochalasin-treated merozoites are able to reorient and form the junction as well as induce membrane flow (1). In the absence of the RBC receptor (e.g., GlycophorinA) the merozoite does not come into close apposition with the RBC membrane and cannot form a junction (2). In the presence of RON2L peptide (3) or AMA1-specific mAb 4G2 that competes with parasite secreted RON2 for binding AMA1 (4), junction formation is blocked. In the presence of mAb 4G2 no vacuoles are seen inside the RBC (4). However, binding of RON2L peptide to parasite AMA1 does not block rhoptry bulb secretion into the RBC (3).
To identify the role of AMA1–RON2 interactions in invasion, we took advantage of earlier studies that defined discrete steps in the invasion process. Cytochalasin-treated Pf merozoites reorient and form the typical junction that includes the electron-dense area below the RBC membrane that follows the area of close apposition with the merozoite. In addition, the RBC invaginates at this point, but the cytochalasin-treated merozoite cannot enter the vacuole, an actin-dependent event (3). In part, the junction consists of a complex of RON proteins as shown by immuno-transmission electron microscopy (present work and ref. 11). We show that blocking the binding of RON2 to the hydrophobic pocket of AMA1 by mAb 4G2 or by the RON2L peptide blocks junction formation. In the presence of mAb 4G2, the merozoite comes into close contact with the RBC at the tip of the apex, but no junction forms. We show that vacuoles within the RBC do not appear if the attachment of AMA1 to RON2 is blocked by mAb 4G2. The close apposition alone in the absence of engagement of AMA1 with RON2 does not trigger release of rhoptry bulb material that is normally observed as membrane flows into the RBC. Similarly, in the presence of RON2L peptide, no junction is formed. However, vacuoles are observed in the RBC cytosol, indicating the release of rhoptry bulb contents into the RBC. Why mAb 4G2 blocks rhoptry bulb release, but RON2L does not, remains a mystery. In addition, other receptor–ligand interactions that occur during the highly coordinated invasion process may play a role alongside AMA1–RON2 in inducing rhoptry bulb release (36). Our results clarify the molecular basis of junction formation but do not address when the AMA1–RON2 interaction occurs, either after RON2 is introduced into the RBC membrane or during the secretion.
In silico modeling of the RON2L peptide–AMA1 complex using the TgAMA1 structure predicts interesting features of this interaction that are consistent with experimental data presented in this paper and suggests explanations for the observed binding properties of the RON2L peptide. For example, in the model, it is observed that the disulfide-bonded loop plays a structural role by orienting the N- and C-terminal helices for binding AMA1 (Figs. S3 and S4). This finding is consistent with our experimental data that the central region encompassing the two cysteines (RON2S) is not sufficient for binding AMA1; yet both cysteines are required for binding of the full-length peptide (RON2L). Intriguingly, in the model, the C terminus of the peptide is immediately adjacent to a solvent exposed, hydrophobic patch on AMA1 (Fig. S4C). In the native RON2, the stretch of amino acids that would continue from the C terminus of the peptide is predominantly hydrophobic. A high degree of conservation in this region between RON2 orthologs coupled to the observation that the C-terminal region following this hydrophobic stretch can interact with TgAMA1 (27) indicates that the third predicted hydrophobic stretch may not be a transmembrane helix. There are, however, some aspects of the interaction that are not explained by the model. In particular, the model does not identify a clear role for the conserved tyrosine (Y251 in Pf and Y230 in Tg) in the hydrophobic cleft that is required for binding RON2. Clearly, only a crystal structure of the AMA1–RON2 complex can define its structure and verify or reject this in silico prediction.
Our results also allow us to comment on what determines the boundaries of the junction over the apical end of the merozoite that creates the moving junction as the parasite enters the RBC. Is the extent of junction the result of the extent of AMA1 on the merozoite apical end? Alternatively, could the RON proteins be limited in their distribution that determines the extent of binding? The RON proteins, if attached to the RBC skeleton, may serve with other proteins the function of disrupting the skeleton so that the merozoite can enter the vacuole. The binding of aldolase to the cytoplasmic tail of AMA1 also suggests that AMA1 may be the link to the merozoite motor. Supporting our data is the recent finding that the cytoplasmic tail of TgAMA1 binds rabbit muscle aldolase and that residues F519W520 in the cytoplasmic tail were shown to be important for invasion (35). Our data do not rule out the possibility that the cytoplasmic tails of the DBL and RH ligands may also bind aldolase.
The other important finding was that invasion can be blocked by Abs to the region of RON2 (RON2L) that binds AMA1. This sequence is conserved, constrained by function, and opens the way for another approach to blood-stage vaccine development. The high level of RON2L-specific IgG required to block invasion indicates that this Ab alone is probably not an effective block but must be combined with another vaccine target such as AMA1 itself. Collectively, our data point to a crucial role for AMA1–RON2 interaction in committing the merozoite for invasion. Developing small molecule inhibitors of this interaction may provide alternative strategies to block invasion.
Methods
Parasite Culture and Isolation of Invasive Merozoites.
A line of Pf FVO selected for prolonged survival of purified, free merozoites by J.D.H. was used in this study. Schizont-infected RBCs were cultured as described (38, 39) and synchronized by temperature (40). Invasive merozoites from this line were purified as follows. Mature schizont-infected RBCs (>90% at more than eight nuclei) were purified on a percoll/sorbitol gradient at 10,000 × g for 10 min at room temperature, and the parasites were returned to culture at 37 °C. The culture was observed every 30 min for the start of rupture of infected RBCs and the release of merozoites. Merozoites released into the culture medium and those in fully mature schizont-infected RBCs ready to rupture were collected by passing the parasites through a 1.2-μm filter. They were passed a second time through the filter to remove any contaminating schizont-infected RBCs. The efficiency of invasion of merozoites isolated by using this method ranged between 3% and 22%.
Peptide Synthesis and Ab Generation.
Details on sequences of peptides used and Ab generation are provided in SI Methods.
Confocal Microscopy.
Purified merozoites were mixed with prewarmed RBCs to 37 °C at 1% hematocrit, and invasion was allowed to proceed at 37 °C and fixed after 20 s to 2 min with an equal volume of ice-cold fixative (5% paraformaldehyde and 0.2% glutaraldehyde in PBS) for 45 min at 4 °C. For Ab labeling details, see SI Methods.
Transmission Electron Microscopy and Immuno-Transmission Electron Microscopy.
For ultrastructural analysis, merozoites in the presence of 2 μM cytochalasin D were added to prewarmed RBCs with or without mAb4G2 (2 mg/mL) and RON2L peptide (5 μM). Cells were fixed with 2.5% glutaraldehyde, 3% paraformaldehyde, 0.05 M phosphate buffer, and 4% sucrose at room temperature for 2 h. For immunolocalization, merozoites were added to 37 °C RBCs for 20 s to 2 min, and cells were fixed in 2.5% paraformaldehyde, 0.1% glutaraldehyde, and 20 mM ethylacetimidate in Hepes-buffered saline (pH 7.3) for 1 h at 4 °C. See SI Methods for details.
Invasion Inhibition Assay.
Invasion efficiency was measured by mixing purified merozoites (~5 × 107/500 μL) with 2.5 × 107 prewarmed RBCs in 1-mL Nunc tubes in the presence of different concentrations of the RON2L peptide, gassed, and incubated at 37 °C. The number of trophozoites was counted 12–15 h later in Geimsa-stained smears. Growth inhibition assay was performed as described (41).
ELISA, 35S-Labeling of Parasite Proteins, and Aldolase Pull-Down Assays.
Details are provided in SI Methods.
Acknowledgments
We thank Drs. Robin F. Anders, Jean-François Dubremetz, and Takafumi Tsuboi for antibodies 1F9 (AMA1), 24C6 (RON4), and RON2, respectively; Lydia Kibiuk for help with art illustration; and Dr. Susham Ingavale for critical reading of the manuscript. This work was supported by the National Institutes of Health Intramural Research Program.
Footnotes
The authors declare no conflict of interest.
This article contains supporting information online at www.pnas.org/lookup/suppl/10.1073/pnas.1110303108/-/DCSupplemental.
References
Articles from Proceedings of the National Academy of Sciences of the United States of America are provided here courtesy of National Academy of Sciences
Full text links
Read article at publisher's site: https://doi.org/10.1073/pnas.1110303108
Read article for free, from open access legal sources, via Unpaywall:
https://europepmc.org/articles/pmc3156155?pdf=render
Citations & impact
Impact metrics
Citations of article over time
Alternative metrics
Smart citations by scite.ai
Explore citation contexts and check if this article has been
supported or disputed.
https://scite.ai/reports/10.1073/pnas.1110303108
Article citations
Plasmodium RON11 triggers biogenesis of the merozoite rhoptry pair and is essential for erythrocyte invasion.
PLoS Biol, 22(9):e3002801, 18 Sep 2024
Cited by: 0 articles | PMID: 39292724 | PMCID: PMC11441699
Conformational variability in the D2 loop of Plasmodium Apical Membrane antigen 1.
J Struct Biol X, 10:100110, 10 Sep 2024
Cited by: 0 articles | PMID: 39324028 | PMCID: PMC11422552
A broadly cross-reactive i-body to AMA1 potently inhibits blood and liver stages of Plasmodium parasites.
Nat Commun, 15(1):7206, 22 Aug 2024
Cited by: 0 articles | PMID: 39174515 | PMCID: PMC11341838
Oral vaccination with a recombinant Lactobacillus plantarum expressing the Eimeria tenella rhoptry neck 2 protein elicits protective immunity in broiler chickens infected with Eimeria tenella.
Parasit Vectors, 17(1):277, 28 Jun 2024
Cited by: 1 article | PMID: 38943202
Plasmodium falciparum AMA1 and CSP antigen diversity in parasite isolates from southern Ghana.
Front Cell Infect Microbiol, 14:1375249, 13 May 2024
Cited by: 0 articles | PMID: 38808064 | PMCID: PMC11132687
Go to all (190) article citations
Data
Data behind the article
This data has been text mined from the article, or deposited into data resources.
BioStudies: supplemental material and supporting data
Similar Articles
To arrive at the top five similar articles we use a word-weighted algorithm to compare words from the Title and Abstract of each citation.
Disrupting malaria parasite AMA1-RON2 interaction with a small molecule prevents erythrocyte invasion.
Nat Commun, 4:2261, 01 Jan 2013
Cited by: 62 articles | PMID: 23907321 | PMCID: PMC3755449
Plasmodium falciparum rhoptry neck protein 5 peptides bind to human red blood cells and inhibit parasite invasion.
Peptides, 53:210-217, 08 Aug 2013
Cited by: 5 articles | PMID: 23932940
Rhoptry neck protein RON2 forms a complex with microneme protein AMA1 in Plasmodium falciparum merozoites.
Parasitol Int, 58(1):29-35, 07 Oct 2008
Cited by: 109 articles | PMID: 18952195
Apical membrane antigen 1 as an anti-malarial drug target.
Curr Top Med Chem, 11(16):2039-2047, 01 Jan 2011
Cited by: 24 articles | PMID: 21619512
Review
Funding
Funders who supported this work.