Abstract
Free full text

Serotype replacement in disease following pneumococcal vaccination: A discussion of the evidence
Abstract
Vaccination with the protein-polysaccharide conjugate vaccine, PCV7, has significantly reduced the burden of pneumococcal disease in populations where it is in widespread use and has had an important public health benefit. This vaccine targets only 7 of the more than 92 pneumococcal serotypes, and there have been concerns that the non-vaccine serotypes (NVTs) could increase in prevalence and reduce the benefits of vaccination. Indeed, among asymptomatic carriers, the prevalence of NVTs has increased substantially, and as a result, there has been little or no net change in the carriage prevalence of the bacteria. In many populations, there has been an increase in pneumococcal disease caused by NVT, but in most cases this increase in NVT disease has been less than the increase in NVT carriage. In this article, we review the evidence for serotype replacement in carriage and disease and address the surveillance biases that might affect these findings. We then discuss possible reasons for the discrepancy between near-complete replacement in carriage and partial replacement for disease, and address the possibility that differences in invasiveness between vaccine serotypes and those causing replacement could contribute to this difference. We contend that the magnitude of serotype replacement in disease can be attributed, in part, to a combination of lower invasiveness of the replacing serotypes, biases in the pre-vaccine carriage data (unmasking), and biases in the disease surveillance systems that could underestimate the true amount of replacement. We conclude by discussing the potential for serotype replacement in disease in the future and the need for ongoing surveillance.
INTRODUCTION
The pneumococcal conjugate vaccine Prevnar, or PCV7, has dramatically reduced the burden of pneumococcal disease in many populations where it is in widespread use [1–8]. PCV7 targets seven of the 92-plus serotypes (so-called vaccine types, or VTs) of Streptococcus pneumoniae (the newly available PCV13 covers the 7 serotypes in PCV7 as well as serotypes 1, 3, 5, 7F, 6A and 19A). The vaccine has greatly reduced the incidence of disease caused by these serotypes both in vaccinated young children and among non-vaccinated groups due to herd immunity and has led to public health benefits in most places in the developed world where it has been instituted. In light of this record and of clinical trials performed in developing countries showing substantial benefits [9–11], there is a strong movement to introduce conjugate vaccines more widely throughout the world. However, as we argue below, the epidemiological impact of broad-scale use of conjugate vaccines in new settings can be difficult to predict, so careful monitoring of disease burden will be needed to assess the initial and long-term impact of mass vaccination in each area of the world where the vaccine is introduced.
The pneumococcal population has changed following widespread introduction of PCV7. Non-vaccine serotypes (NVTs) have increased among asymptomatic carriers in a process dubbed “serotype replacement”1 [12–13], and to a lesser extent, NVTs have also increased as causes of invasive disease. While the reported magnitude of this increase in disease among NVTs has been relatively modest in most countries, such changes have the potential to dampen the overall public health benefit of the vaccine.
A key question is why there has apparently been complete serotype replacement among asymptomatic carriers but incomplete replacement in disease. In other words, why has the vaccine successfully reduced the burden of pneumococcal disease when the prevalence of bacterial carriage has not changed? Understanding this issue will be critical to predict the impacts of future pneumococcal vaccination programs, particularly in the developing world where pneumococcal epidemiology differs from that in Europe and the United States. To address this question, we here review the evidence for serotype replacement in carriage and disease, evaluate potential biases in the data and reasons for heterogeneity between studies, discuss the biological and epidemiological features of the serotypes, and address the relative contributions of vaccination and other factors to the increases in non-vaccine serotypes. We also discuss the potential impacts of serotype replacement following the introduction of conjugate vaccines in diverse settings.
Literature review and inclusion criteria
We identified relevant studies on serotype replacement in disease with a comprehensive search of PubMed (combination of the search terms pneumococ*, Streptococcus pneumoniae, pcv7, conjugate, serotype) and reference lists. Since we were interested in the question of population-wide serotype dynamics, we included only studies that reported the incidence of VT and NVT disease in the general population such as nationwide or hospital-based surveillance systems or vaccine trials on otherwise healthy individuals and excluded those that focused exclusively on high-risk groups. Additionally, we excluded studies that only reported changes in proportions of serotypes, rather than absolute number of cases or incidence since proportions do not give an indication of the impact of replacement on disease burden. As noted in Table 1, in instances where multiple publications resulted from the same study, we extracted data from the most recent available publication. We note some additional studies in Table 1 that were too small to infer changes in the serotype distribution but which might be of interest to readers. We also discuss the unpublished surveillance data from England and Wales that is publicly available on the internet but do not include it in the summary table because the methods for the collection and analysis of this unpublished data are not yet widely available.
Table
Selected studies of changes in serotype distribution in disease
Study | Country | Surveillance years | Year vaccine introduced | Study details | Age (years) | Periods compared* | Change in NVT incidence | Net change IPD incidence |
---|---|---|---|---|---|---|---|---|
North America | ||||||||
Kaplan [48] | USA (multicity) | 1994–2008 | 2000 | 8 hospitals nationwide | Pediatric | 1994–99 vs 2007–08 | NA | −53% |
Kaplan [48] | USA (multicity) | 1994–2008 | 2000 | 8 hospitals nationwide | Pediatric | 2001 vs 2007–08 | +100%* | −16%* |
Techasaensiri [85] | USA (Dallas) | 1999–2008 | 2000 | Single hospital | ≤18 | 1999 vs 2005–08 | +177% | −22% (among serotyped cases [−38% among all IPD cases]) |
Bettinger [4] | Canada | 2000–07 | 2001 (regional), 2005 (nationwide) | Active, nationwide | <5 | 2000–01 vs 2006–07 | +90% | −61% |
Jacobs [47] | USA (Cleveland) | 1999–2007 | 2000 | Hospital laboratory (invasive isolates only) | ≤18 and >18 | 1999 vs 2005–07 | ≤18 years: +200%; >18 years: +88% | ≤18 years: −69%; >18 years: −27% |
Pilishvili [2] | USA (Nationwide) | 1998–2007 | 2000 | Active, nationwide | <5 and ≥65 | 1998–99 vs 2006–07 | <5 years: +32% (+102% hospitalized only); ≥65 years: +32% (36% hospitalized only) | <5 years: −77% (−60% hospitalized only); ≥65 years: −36% (−35% hospitalized only) |
Wenger [74] | USA (Alaska) | 1996–2007 | 2000 | Population-based laboratory | <5 | 1996–2000 vs 2005–07 | Alaska native (Yukon): +185%; non-native rural: +321%; non-native urban: +240% | Alaska native (Yukon): −22%; non-native rural: −29%; non-native urban: −48% |
Lacapa [41] | USA (Apache) | 1991–2006 | 2000 | Active surveillance | <1 and ≥ 18 | 1991–97 vs 2004–06 | <1 year: −7%; ≥18 years: +32% | <1 year: −68%; ≥18 years: +4% |
Weatherholtz [42] | USA (Navajo) | 1995–2006 | 2000 | Active surveillance | <5 and >18 | 1995–97 vs 2004–06 | <5 years: −8%; >18 years: +6% | <5 years: −60%; >18 years: −2% |
Black [51] | USA (California) | 1996–2005 | 2000 | Laboratory cases in Northern California Kaiser system | <5 | 1996–2000 vs 2003–05 | −5% | −78% |
Messina [86] | USA (Texas) | 1999–2005 | 2000 | Prospective, hospital | <18 | 1999 vs 2005 | +171%† | −31% |
Steenhoff [87] | USA (Philadelphia) | 1999–2005 | 2000 | Single hospital | <18 | 1999–2000 vs 2001–05 | +75% | −57% |
Byington [88] | USA (West) | 1996–2003 | 2000 | Intermountain healthcare system*** | <2 and <18 | 1997–99 vs 2001–2003 | <18 years: +125%**** | <2 years: −40%; <18 years: +13% |
Europe | ||||||||
Foster [45] | Oxfordshire, UK | 1995–2009 | 2006 | Regional laboratory surveillance | <2 and ≥2 | 1995–2005 vs 2006–08 | <2 years: +56%; ≥2 years: +18% | <2 years: −48%; ≥2 years: −16% |
Maraki [89] | Crete, Greece | 2000–09 | 2006 | Single hospital | ≤14 | 2000–04 vs 2005–09 | +21% | −38% |
Doit [59] | France | 2001–08 | 2002/2003 | Single hospital | <16 | 2001–04 vs 2005–08 | +85% | −8% |
Harboe [1] | Denmark | 2000–08 | 2007 | Nationwide surveillance | <2 and ≥5 | 2000–07 vs 2008 | <2 years: −10%; ≥5 years: 0 | <2 years: −56%; ≥5 years: −9% |
Rodenburg [8] | Netherlands | 2004–08 | 2006 | Hospital sentinel surveillance | <2¶ and ≥65 | 2004–06 vs 2006–08 | <2 years: +71%; ≥65 years: +6% | <2 years: −44%; ≥65 years: +2% |
Rückinger [7] | Germany | 1997–2008 | 2006 | Nationwide hospital and laboratory surveillance | <2 | 1997–2003 vs 2007–08 | +3% | −56% |
Vestrheim [3] | Norway | 2004–08 | 2006 | Nationwide, mandatory reporting | <5 and ≥65 | 2004–05 vs 2008 | <5 years: −5%; ≥65 years: +22% | <5 years: −72%; ≥65 years: −15% |
Adranuy [5] | Barcelona, Spain | 1997–2007 | 2001 (low coverage) | Single hospital (invasive isolates only) | ≥65 | 1997–2001 vs 2005–07 | +66% | +23% |
Fenoll [55] | Spain | 1979–2007 | 2001 (variable coverage) | Nationwide hospitals, passive (adjusted for ascertainment bias) | All ages | 1996–2000 vs 2004–05 | +38% | +2% |
Guevara [90] | Navarre, Spain | 2001–07 | Active surveillance | <5 and ≥65 | 2001–02 vs 2006–07 | <5 years: +47%; ≥65 years: +46% | <5 years: −12%; ≥65 years: +4% | |
Pérez-Trallero [43] | Spain (Basque) | 1996–2007 | Single hospital (adjusted for population size) | <5 and ≥65 | 1996–2000 (<5 years) or 1998–2000 (≥65 years) vs 2005–07 | <5 years: +93%; ≥65 years: +32% | <5 years: −11%; ≥65 years: −3% | |
Muñoz-Almagro [54] | Barcelona, Spain | 1997–2006 | 2001 (low coverage) | Single hospital | <2 | 1997–2001 vs 2002–06 | +531% | +58% |
Bingen [91] | France | 2001–05 | 2002/2003 | Nationwide hospital laboratory (meningitisonly), among serotyped cases | <2|| | 2001–02 vs 2005 | +44% | −21% |
Dias [92] | Portugal | 1999–2004 | 2002 | Population/laboratory surveillance | <1 | 2002–04 | +29% | −18% |
Aristegui [56] | Basque and Navarro, Spain | 1998–2003 | Retrospective (pre-vaccination), prospective (post-vaccination) in several hospitals*** | <2 and 2–5 | 1998–01 vs 2002–03 | <2 years: −3%; 2–5 years: +6% | <2 years: −27%; 2–5 years: +10% | |
Australia | ||||||||
Hanna [53] | Queensland, Australia | 1999–2007 | 2001 | Laboratory-based (indigenous population only) | <5 and ≥15 | 1999– 2001 vs 2005– 07 | <5 years: −3%; ≥15 years: +39% | <5 years: −61%; ≥15 years: +7% |
Lehmann [52] | Western Australia | 1997–2007 | 2001/2005 | Enhanced | <2 and 15–29 | 1997–2001 vs 2005–07 | Aboriginal, <2 years: +33%; non-aboriginal, <2 years: +53%; aboriginal, 15–29 years: +166% | Aboriginal, <2 years: −46%; non-aboriginal, <2 years: −67%; aboriginal, 15–29 years: +88% |
We have summarized the findings of each study by presenting both net percentage changes in pneumococcal disease before and after vaccination and the percentage change among NVTs. In many instances, these numbers depend on which years were included before and after vaccination and which age-groups are reported. We have reported the years and age-groups that were used for the comparisons and noted other factors that might influence the calculations. Note that this list of studies is not exhaustive and we have excluded publications that repeat data from the same study. Additional studies were excluded for the following reasons: lacked pre-vaccine baseline data; reported relative, rather than absolute, serotype frequencies; lacked a pre-vaccine baseline period; size was too small to infer changes in serotype distribution. Excluded studies came from France: [59, 93–94]; Spain: [6, 95–98]; Canada: [99–102], USA: [103–105] Taiwan: [106]; South Korea: [107]. Additional studies that overlapped those listed were also excluded. NVT=Non-vaccine types.
IPD=Invasive pneumococcal disease.
We attempted to summarize the findings of each study by presenting both net percent changes in pneumococcal disease before and after vaccination and the percent change among non-vaccine serotypes. In many instances, the magnitude of these numbers depends on which years were included pre- and post- vaccination and which age groups are reported. For studies that had such complicating factors, we noted which years and age groups were used for the comparisons and noted other factors that might influence the calculations.
1. Nasopharyngeal carriage: the precursor to invasive disease
The first reports of serotype replacement came from a double-blind, placebo-controlled randomized trial in The Gambia that demonstrated that while carriage of VTs significantly declined in vaccinated infants, carriage of NVTs significantly increased [14]. As a result of this change, the net impact on carriage prevalence in the trial was minimal. A randomized Israeli vaccine trial did not demonstrate a significant impact of serotype replacement in carriage among vaccinated children [15], but subsequent trials in Israel did show evidence of replacement [16]. Additionally, randomized trials from South Africa [17], The Netherlands [18], the US Navajo population [19], and a subsequent trial in The Gambia [20] have demonstrated replacement in carriage. Because these were randomized controlled trials, they implicate vaccination, rather than other factors, as the cause of the increase in NVT carriage.
Since the licensure of PCV7, several observational studies have documented changes in the pneumococcal serotypes present in carriage. A pediatric cross-sectional study from Massachusetts, USA, has demonstrated that in the first seven years of universal vaccination, the PCV7 serotypes have been virtually eliminated from carriage in young children [21]. However, carriage of NVTs increased dramatically, so the overall carriage prevalence was comparable between the pre- and post-vaccine eras [21]. Additionally, serotype replacement has been observed in carriage studies from Norway [22], France [23–24], Portugal [25], Greece [26], Texas [27], and Alaska [28].
The increase in carriage of NVT in these studies could be due, in part, to the artifact of “unmasking” [29], in which the reduction in prevalence of VTs has made it easier to detect NVTs that were present in the population, but undetected, in the absence of vaccination. Since the commonly-used serotyping methods require that only one or two colonies be evaluated, investigators are unlikely to detect co-colonization with multiple serotypes. Reducing the prevalence of VT by vaccination could lead to an increase in the detection of NVT, even in the absence of any real increase in the acquisition of NVT.
If unmasking were playing an important role, then one would not necessarily expect that an increase in the detection of NVTs in carriage would be followed by a concomitant increase in disease. However, while unmasking might partially account for the increase in the detection of NVTs among carriage isolates, it is unlikely to fully explain it, as an analysis of the South African trial suggested [29]. Estimates of multiple carriage rates range from less than 10% to 30% of individuals in unvaccinated populations, with many studies reporting multiple carriage rates on the lower end of that range [22, 30–33]. In general, if the overall prevalence of carriage did not change after vaccination, but the composition shifted from a mix of VTs and NVTs to almost pure NVTs, then implausibly high levels of multiple carriage would have to be invoked to attribute the entire observed effect to unmasking [29].
In reality, increased acquisition of new serotypes and unmasking both likely contribute to the increase in NVTs in carriage [34]. There may be some contribution from a phenomenon that resembles unmasking but is biologically similar to true replacement: if vaccination reduces the proportion of individuals co-colonized with VT and NVT, and co-colonized individuals have a lower density of colonization with NVTs than singly-colonized hosts, then there could be both an increase in detection probability (the artifact of unmasking) and an increase in the density of NVTs, which could lead to an increased probability of invasive disease or transmission to new hosts (increased acquisition rate). One study has addressed this question, and although the point estimate suggested higher NVT colonization density in vaccinated individuals, the effect was not statistically significant [35].
Theoretically, one would expect that large-scale use of a vaccine would result in a greater increase in NVT carriage than is observed in an individually-randomized clinical trial [36] because mass vaccination changes the exposure of all individuals in the community (due to indirect effects) as well as the susceptibility of vaccinated individuals to NVT carriage. Indeed, complete disappearance of VT carriage, and large increases in NVT carriage, have been observed only in highly-vaccinated populations [21], whereas clinical trials showed only partial reductions in VT and smaller concomitant increases in NVT carriage.
In summary, there is strong evidence that colonization with NVTs increases in vaccinated populations. Randomized trials implicate vaccination as the cause of these increases, and observational evidence is consistent with theoretical predictions that both larger reductions in VT carriage and larger increases in NVT carriage should occur after mass vaccination.
2. Invasive disease
There have been numerous studies that have evaluated serotype-specific IPD incidence before and after the introduction of PCV7 (Table 1), but the measured impact of vaccination on NVT disease has been inconsistent.
In classical, individually-randomized trials, only a small fraction of the population is typically vaccinated. Therefore, such trials typically have a minimal impact on the overall bacterial population compared to what will occur with mass vaccination. For this reason, individually-randomized trials are not designed to detect the long-term impact of replacement [36–38]. Moreover, these trials are not powered to detect replacement disease. The largest randomized trial of a pneumococcal conjugate vaccine, with some 37,000 children, had only 3 cases of NVT invasive disease in the vaccinees and 6 in the controls [39]. The next-largest trial, in The Gambia, was also not powered for the detection of changes in NVT disease, and in this case there was a trend in the other direction, with 15 cases in the vaccinated group and 9 in the controls [9]. For these reasons, data from randomized trials are inconclusive about serotype replacement in disease. Consequently, the bulk of the data regarding replacement in IPD comes from observational studies.
Observational studies of IPD following vaccine introduction are subject to ecological and sampling biases. On the other hand, these post-licensure observational studies more accurately reflect the population-wide impact of mass vaccination. As with carriage, disease caused by NVT is expected to increase more in a well-vaccinated population than among vaccine recipients in a clinical trial [36]. In addition, studies of IPD are not susceptible to unmasking since a case of IPD is thought to be caused by a single clone [40]. As a result, increases in NVT IPD likely represent true serotype replacement if biases in the surveillance systems can be properly controlled. In the following sections, we describe the methodologic issues around these observational studies and then summarize their findings
Overview of IPD surveillance systems and potential sources of bias
With any surveillance system, the possibility exists for bias if either clinical practices or case reporting change over time. Two reports in North America have indicated that the proportion of febrile patients who are blood-cultured has decreased following the introduction of the vaccine [41–42]. As another example, the emergency department at Children’s Hospital Boston discontinued performing routine blood cultures in febrile, well-appearing 6–36 month old children for the detection of pneumococcal occult bacteremia 3 years after the introduction of PCV7. The widespread use of routine blood cultures for febrile children in the United States prior to vaccination and the subsequent reduction of this practice could make it appear that the incidence of disease was artificially high prior to the introduction of PCV7. As a result, the apparent decline in disease in children several years into universal vaccination campaign would be inflated, and the detection of replacement disease caused by NVTs would be reduced. Due to clinical practice, this issue would be most pronounced in studies of young children in emergency departments, and less pronounced, though perhaps still relevant, in studies of hospitalized patients. On the other hand, reports from Spain have indicated that both the rate of blood-culturing and reporting of IPD have increased since the introduction of the vaccine, and if not properly accounted for, they could make replacement appear more significant than it is [43–44].
Active surveillance systems, which perform record reviews and try to identify all cases in participating clinics, should be less prone to the issues of reporting bias. However, such active surveillance systems might be more likely to detect cases of occult bacteremia, and these might be more prone to changes in blood-culturing practices than severe diseases such as invasive pneumonia, sepsis or meningitis.
Passive surveillance systems, which rely on laboratories to report cases, could potentially be prone to reporting biases as well as changes in blood-culturing practices. Finally, a number of studies report data from single hospitals. Such studies are prone to all of the above biases, and they also might be more likely to reflect short-term local fluctuations in serotype patterns.
One way to minimize biases due to changes in surveillance systems would be to focus on well-defined severe diseases such as meningitis, for which the diagnostic criteria is unlikely to change significantly over time. However, reports of meningitis could still be biased by changes in reporting rates in passive surveillance systems.
Estimates of disease incidence in all of the above systems will be influenced by natural fluctuations in overall pneumococcal disease incidence. Depending on the years used to measure the pre- and post- vaccine disease incidence, such fluctuations could either magnify or dampen the effect of the vaccine. For instance, Foster et al. [45] recently demonstrated a multi-year increase in pneumococcal disease incidence that occurred in the late 1990s and early 2000s. Inclusion of these years in the calculation of pre-vaccine incidence makes it appear that there was a larger decline in disease incidence than if only the years in the mid-2000s were used in the calculation.
Likewise, the length of the post-vaccine follow-up period can influence the reported magnitude of changes in disease incidence. Carriage studies suggest that the relative prevalence of serotypes in the nasopharynx is in flux for the first few years of vaccination and then reaches a steady state [46]. As a result, there appears to be a lag between vaccine introduction and the increase in IPD caused by non-vaccine serotypes in some populations [47–48]. This could be attributed to the amount of time required to reach full vaccine coverage and for vaccine serotypes to be eliminated, especially in the general population through herd effects. Additionally, changes in the bacterial population, such as serotype switching, could have a delayed effect on disease incidence. Studies reporting less than a few years of post-vaccine incidence data may thus underestimate replacement.
Evidence of serotype replacement in disease
PCV7 was first introduced in the United States in 2000. The ABCs system in the United States has demonstrated a clear drop in VT disease and a modest increase in NVT disease, most notably caused by serotype 19A, though other serotypes have increased as well [2]. As reported by Pilishvili et al [2, 49], the increase in non-vaccine serotypes in this population is most apparent among hospitalized cases. This could be explained if blood culturing has remained routine for patients ill enough to be hospitalized but has declined in frequency for less severe cases. Indeed, the incidence rates for bacteremias without foci caused by NVTs decreased in all age groups between the pre- and post-PCV7 eras, while invasive pneumonia and meningitis caused by NVTs showed increased incidence rates in all age groups [2]. It is important to note, however, that despite the increases in non-vaccine serotypes, the overall rate of IPD in the pediatric population is still significantly lower than in the pre-vaccine era even when considering only hospitalized cases [2]. Additionally, the same study reported a 64% decline in pediatric meningitis cases, which is unlikely to be biased by changes in clinical practice. This decline in IPD is further confirmed by a drop in hospitalized all-cause pneumonia cases in children <2 [50].
Other studies from North America show varied results (Table 1). These data include both hospital-based laboratory surveillance and active surveillance systems. Most studies from North America report some degree of serotype replacement (Table 1), although one study reported no detectable increase in NVTs [51].
A study of Aboriginals in Western Australia [52] found that PCV7 reduced the incidence of IPD in young children and the elderly, but there was a significant net increase in IPD in young adults driven by an increase in NVTs. In another population, there was no evidence of replacement in vaccinated children, but there was a significant increase in NVTs in adults, which offset the herd immunity benefits of the vaccine [53].
In Europe, reports on serotype replacement also give varied results. Several studies from Spain have examined the issue, with some areas reporting complete serotype replacement with no net effect of the vaccine on IPD incidence [5, 54–55] while another reported no increase in NVTs [56]. The Spanish data are further complicated by regional differences in blood culturing practices and by increases in blood culturing and reporting rates since the introduction of PCV7 [43–44, 57]. In France, the vaccine had no overall effect in the most recent national study of pediatric meningitis in children under 5, though it showed a benefit in those under 2 [58]. The effect on bacteremia in France was modest [59]. Interpretation of the data from Spain and France is also difficult because of the relatively low initial vaccine coverage in these countries, the gradual introduction of the vaccine, and regional differences in vaccine uptake. Elsewhere in Europe, The Netherlands [8] demonstrated a 44% increase in NVT IPD in children <2. Denmark [60] and Norway [3] found modest increases in specific serotypes, but this did not result in a net increase in NVT. Germany has not seen any evidence of serotype replacement as of 2008 [61]. In England, the incidence of NVTs more than doubled in children <2 after vaccine introduction, but there was still a 48% net decline in disease [45, 49, 62]. Data from the HPA in England and Wales also demonstrate a net decline in disease incidence in children despite significant serotype replacement, but in the elderly, the increase in NVTs completely offsets the decline in VTs, so there was no “herd immunity” effect in this population [49, 62].
In summary, most locations have reported a net decline in the incidence of invasive pneumococcal disease in young children, especially those under 2. Many, but not all, studies also report a substantial increase in NVT in young children. In contrast to the overall decline in IPD reported elsewhere, data from France, Spain, and Alaska Natives suggest little net benefit of vaccination in children, especially in those 2 and older. Among non-vaccinated age groups, there is substantial heterogeneity in the reported magnitude of the indirect benefits of vaccination, with some studies reporting complete replacement resulting in no net indirect benefit, while others report relatively little replacement. Further consideration of all of these data will be required to determine how much of the heterogeneity is due to true differences in serotype replacement and how much is due to surveillance artifacts.
3. Causes of observed replacement in disease
Now that we have reviewed the evidence for serotype replacement in carriage and disease, we return to the questions of why serotype replacement has been complete in carriage but not in disease, how much the incidence of invasive disease has truly been affected by vaccination and replacement, and what factors are likely to be responsible for the increase in non-vaccine serotypes.
Why is there a discrepancy in the magnitude of replacement between the carriage and disease data?
The incidence of a serotype causing invasive pneumococcal disease is determined both by the prevalence of that serotype among carriers and the proportion of carriage episodes that results in a case of invasive disease—frequently referred to as “invasiveness” or the “case-carrier ratio”.
The carriage prevalence and the invasiveness of a serotype are likely influenced by the microbiological properties of the strains. The capsular polysaccharides play an important role in evading host immune responses. More heavily encapsulated serotypes tend to be carried more frequently [63], and capsular polysaccharides vary in their ability to prevent complement deposition [64], which might influence the invasiveness of a serotype [65]. Additionally, other microbial factors such as adhesins, toxins, and proteins that allow the bacterium to avoid host immune effectors likely influence the carriage prevalence of a serotype and its invasiveness (reviewed in [66]). Interestingly, there is an inverse correlation between the carriage prevalence of a serotype and its invasiveness [67].
Invasiveness in children varies between serotypes, but the invasiveness of a given serotype is comparable in different populations [68–71]. One would expect, then, that if a serotype increases in carriage, and invasiveness does not change, then there should be a proportional increase in disease. Therefore, if the non-vaccine serotypes that increase in carriage are less invasive than the vaccine serotypes that they replace, there should be a decrease in disease burden. Conversely, if the replacement serotypes are more invasive, there should be a net increase in disease. The NVTs that have increased in carriage do tend to be less invasive than the serotypes targeted by PCV7 [72]. This is particularly true if the highly invasive, yet rarely carried, serotypes 1 and 5 are excluded. Recent estimates from our group suggest that due to invasiveness differences between serotypes, we would have expected approximately a 30% decline in invasive disease after the introduction of PCV7 [73].
Given these points, the apparent discrepancies in serotype replacement between carriage and disease might be attributable to several factors. First, the lower invasiveness of the replacement serotypes compared to the vaccine serotypes could contribute to the net decline in disease incidence. Second, biases in the detection of vaccine serotypes in pre-vaccine studies of pneumococcal carriage (so-called “masking”) might have resulted in NVTs appearing to increase in carriage prevalence more than they did. Finally, biases in the pre- and post-vaccine disease incidence measurements caused by changes in surveillance methodologies or secular trends could have resulted in inaccurate comparisons between these two periods.
What is the true magnitude of the increase of NVTs in disease?
As discussed above, some studies likely under-report serotype replacement while other studies likely over-report it—in both cases due to changes in blood culturing, clinical practices, or reporting over the course of data collection. Despite these issues, we believe two conclusions can be reached: 1) nearly all studies indicate that there is a net positive benefit of vaccination with PCV7 apparent at least during the first three years of institution and 2) evidence for serotype replacement in disease is apparent in most populations. Most studies report a net decline in disease of 40–60% in children even after accounting for replacement, though many of these studies have a short post-vaccine follow-up period. As noted in the previous section, however, considerable heterogeneity exists between studies, and it is not yet clear how much of this variation can be attributed to true differences or to surveillance artifacts. The data from Alaska, in which the benefits of vaccination were smaller among rural Alaska natives, suggests that there might be real differences in the magnitude of replacement between groups of people. These differences could depend on differences in the proportions of serotypes present prior to vaccination, the coverage of the vaccines, and socioeconomic differences between the groups [74]. As such, care must be taken when predicting the benefits of mass vaccination in new settings.
Finally, while this review has focused on reported changes in serotype incidence and distributions in the general population, the patterns and magnitude of replacement could be different among those with immunodeficiencies and other co-morbidities [75]. In particular, the greater susceptibility of these high-risk groups to pneumococcal disease, could lead to a higher level of replacement disease than what is seen in the general population. Thus, the prevalence of such co-morbidities could influence the overall magnitude of serotype replacement in a population.
What has been the relative contribution of vaccination to the increase in NVTs in carriage and disease?
This question has implications for the introduction of future pneumococcal vaccines. If the increase in NVTs is caused simply by factors not related to vaccination, then one would not necessarily expect additional serotype replacement when the new vaccine is introduced. However, if serotype replacement in carriage or disease is attributable to vaccination, then we might expect that other serotypes will increase as the strains targeted by a new vaccine are eliminated. It has been suggested that antibiotic usage patterns or long term “secular trends” could influence changes in serotypes [76–77]. Indeed, serotype 19A has increased in some areas where the vaccine has not been introduced [78–79], and certain serotypes, such as serotype 1, do exhibit long-term fluctuations in prevalence [55, 60]. Such patterns unquestionably occur, but as the randomized trials demonstrate for carriage, the vaccine changes serotype prevalence more rapidly and to a greater extent than occurs from natural fluctuations.
Multiple mechanisms may contribute to the overall serotype patterns. The vaccine could create an open niche that could be filled by NVTs. Which NVTs increase, however, could be influenced by antibiotic use and resistance and other general biological properties of the strains [21, 63]. The open niche created by vaccination could amplify these “secular trends” of the serotypes. It is unlikely, however, that antibiotic use and resistance are the sole cause of increasing IPD incidence from NVTs. Prior to vaccination in the United States, selection pressure for antibiotic resistance resulted in regional differences in the prevalence of resistance within serotypes but not in regional differences in the serotype composition of IPD isolates [80]. This argues that antimicrobial use may not be capable of changing serotype patterns appreciably. Most compelling are the data from Norway, where there was a sharp rise in serotype 19A IPD approximately 1 year after the introduction of the vaccine [3]. This increase comes despite low antibiotic pressure in Norway, and indeed the serotype 19A clone that emerged was susceptible to penicillin.
Taken together, the evidence from randomized control trials, cross-sectional carriage studies and population-based disease surveillance make it almost certain that a substantial proportion of observed replacement in disease has been caused by the vaccine. Since invasive disease typically follows colonization, one of the following, unlikely scenarios would have to be true for the vaccine not to have caused replacement in disease: (a) the observed increase in NVT carriage was entirely attributable to unmasking, hence there was no true serotype replacement in carriage, or (b) the invasiveness (ratio of IPD cases to carriers) of NVT declined since vaccination, fully compensating for any increase in carriage. Scenario (a) is unlikely because it would imply that every VT carrier before vaccination had both VT and NVT strains, and the NVT strains have just been unmasked. It would also imply that the unmasking is not followed by an increase in the density of NVT strains, a claim that seems biologically unlikely and contradicts animal studies of strain competition [81]. Scenario (b) is implausible because we know of no mechanism by which vaccination should reduce the invasiveness of NVTs, and the data on invasiveness from many different populations have been strongly consistent [68–71]. Finally, the temporal relationship between the introduction of PCV7 in various countries and the rise of non-vaccine serotypes is unlikely due to coincidence given that the vaccine was introduced at different times in different countries (Figure). A recent study from England demonstrates that while NVTs were increasing in disease prior to the introduction of PCV7 in that country, they increased significantly more rapidly after vaccination [45].
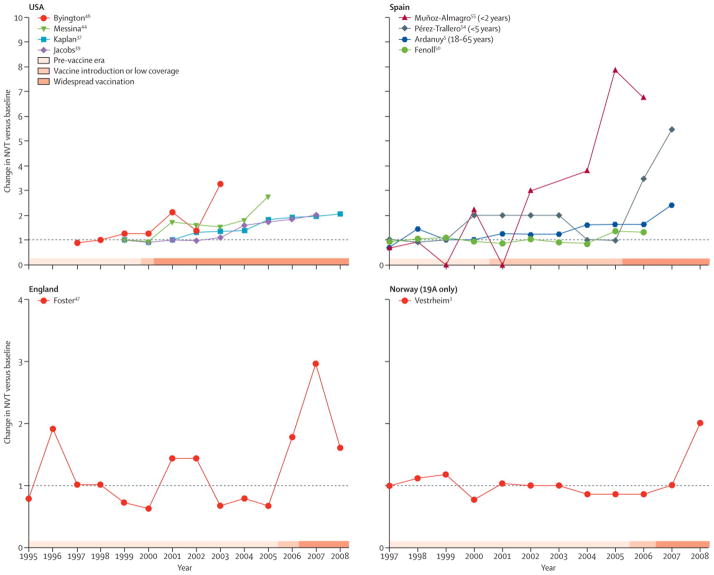
Timing of the increase in the incidence of non-vaccine serotypes relative to vaccine introduction in select studies that report serotype replacement. Light grey bars indicate pre-vaccine era, medium-grey bars indicate the period of vaccine introduction or low coverage, and the dark grey bars indicate widespread vaccination. The values represent the incidence in each year divided by the mean pre-PCV7 incidence, as indicated by the shaded bars and table 1. The data from the U.S., Spain, and England represent changes in all non-vaccine serotypes, and the data from the Norway study is just serotype 19A. There was no net increase among NVTs in Norway as of 2008.
5. Vaccination in developing countries
It is clear from the studies reviewed here that despite the increased incidence of NVTs causing IPD in many populations, PCV7 has had a significant positive benefit for pediatric disease, especially in children under 2, where it is in widespread use. It is also clear that the measured benefit varies across populations, and that some of this variation is likely real, reflecting host and pathogen population characteristics. As reviewed previously [82], the epidemiology of IPD in developing countries differs from that in other parts of the world, and the high carriage prevalence, co-infections, and different serotype distributions in these populations add uncertainty to efforts to extrapolate from existing data. It is possible that the extent of serotype replacement could be different—either larger or smaller—in a setting where the vaccine covers fewer of the serotypes responsible for IPD and the composition of serotypes is different. The clinical trials from The Gambia and South Africa suggest that the vaccines could provide a significant benefit in these areas, but the net reductions in IPD observed in a randomized trial might differ in magnitude when mass vaccination is introduced, magnifying both the herd effects and the impact of serotype replacement compared to what one observes in a randomized trial.
As noted above, there is support for the rapid introduction of pneumococcal conjugate vaccines in developing countries. We believe that the incidence of disease in these new areas should be monitored following the introduction of the vaccine to confirm its effectiveness. Due to resource limitations, it is unlikely that comprehensive, laboratory-based surveillance systems, such as those used in the United States and parts of Europe, could be used in many developing countries. However, existing pneumococcal research sites, such as those in South Africa, The Gambia, and eastern Africa (netSPEAR) could serve as sentinel sites for the rest of Africa. Since pneumonia, both bacteremic and non-bacteremic, constitutes the largest burden of pneumococcal disease, changes in pneumonia incidence could be the focus of surveillance. A significant and sustained drop in all-cause pneumonia incidence, as has been seen in the United States [50], could be used as an indicator of vaccine effectiveness. Finally, the serotype composition among carriers should be monitored where possible both to ensure that vaccine serotypes are being eliminated as expected and to detect if there is an increase in any especially invasive serotypes. Carriage surveys are relatively inexpensive and feasible compared to population-based IPD surveillance. Using carriage data along with known invasiveness data could serve as an indirect way to monitor serotype replacement [83].
6. Future prospects for serotype replacement in disease
The evidence presented here strongly support the notion that serotype replacement has occurred in IPD in most populations and is caused by the vaccine. We should assume that the introduction of new conjugate vaccine formulations in the future will again be met with complete serotype replacement among carriers and some amount of replacement in disease that will depend, in part, on the invasiveness of the colonizing serotypes. The 13-valent vaccine that was recently introduced has the potential to have significant and sustained impacts on disease, particularly in developing countries. Serotypes 1 and 5, which are covered by this vaccine, are rarely carried but cause a lot of disease in many areas. As a result, the elimination of these two specific serotypes is unlikely to be followed by substantial replacement [12, 36]. While it is difficult to predict how the composition of non-vaccine serotypes will change following PCV13 or whether the characteristics of the serotypes will change, projections based on the invasiveness of the serotypes suggest that this new vaccine will result in additional reductions in disease incidence [73]. Such projections are valuable for defining likely scenarios, but limitations of our biological knowledge and of the formalization of that knowledge in theoretical models mean that model predictions are no substitute for careful monitoring [84]. Long-term surveillance of both invasive pneumococcal disease, carriage, and non-bacteremic pneumonia, which constitutes a major portion of disease burden, will be critical to ascertain whether the vaccines are having the desired effect of reducing the incidence of disease over the long term.
Acknowledgments
We thank Anthony Scott and Elizabeth Miller for helpful discussions, particularly on the topics of surveillance artifacts (EM) and design of surveillance in low-resource areas (AS), and for critical feedback on the manuscript.
Funding: This study was supported by NIH research grant R01 AI048935 to ML and R01 AI066013 to RM.
Footnotes
1In nasopharyngeal carriage, we define serotype replacement as an increase in the proportion of individuals in a population who harbor NVTs in their nasopharynx following vaccine introduction. For invasive disease, serotype replacement is defined as an increase in the incidence of invasive disease caused by NVTs following vaccine introduction.
Conflicts of Interest: DMW declares no conflicts. RM is a member of the Scientific Advisory Board of Genocea Biosciences. ML has accepted honoraria or consulting fees from Pfizer and Novartis.
Author contributions: DMW reviewed the literature and DMW, RM, and ML wrote the manuscript.
References
Full text links
Read article at publisher's site: https://doi.org/10.1016/s0140-6736(10)62225-8
Read article for free, from open access legal sources, via Unpaywall:
https://europepmc.org/articles/pmc3256741?pdf=render
Citations & impact
Impact metrics
Citations of article over time
Alternative metrics
Smart citations by scite.ai
Explore citation contexts and check if this article has been
supported or disputed.
https://scite.ai/reports/10.1016/s0140-6736(10)62225-8
Article citations
Pneumococci Isolated From Children in Community-Based Practice Differ From Isolates Identified by Population- and Laboratory-Based Invasive Disease Surveillance.
J Infect Dis, 230(5):1243-1252, 01 Nov 2024
Cited by: 1 article | PMID: 38591247
A genome-based survey of invasive pneumococci in Norway over four decades reveals lineage-specific responses to vaccination.
Genome Med, 16(1):123, 25 Oct 2024
Cited by: 0 articles | PMID: 39456053 | PMCID: PMC11515192
Molecular epidemiology of Streptococcus pneumoniae isolates causing invasive and noninvasive infection in Ethiopia.
Sci Rep, 14(1):21409, 13 Sep 2024
Cited by: 0 articles | PMID: 39271789 | PMCID: PMC11399344
A microbiological and genomic perspective of globally collected Escherichia coli from adults hospitalized with invasive E. coli disease.
J Antimicrob Chemother, 79(9):2142-2151, 01 Sep 2024
Cited by: 0 articles | PMID: 39001716 | PMCID: PMC11368426
Prediction of post-PCV13 pneumococcal evolution using invasive disease data enhanced by inverse-invasiveness weighting.
mBio, 15(10):e0335523, 29 Aug 2024
Cited by: 0 articles | PMID: 39207103 | PMCID: PMC11481909
Go to all (537) article citations
Other citations
Similar Articles
To arrive at the top five similar articles we use a word-weighted algorithm to compare words from the Title and Abstract of each citation.
The rise and fall of pneumococcal serotypes carried in the PCV era.
Vaccine, 35(9):1293-1298, 01 Feb 2017
Cited by: 39 articles | PMID: 28161425
Five winters of pneumococcal serotype replacement in UK carriage following PCV introduction.
Vaccine, 33(17):2015-2021, 14 Mar 2015
Cited by: 78 articles | PMID: 25776920 | PMCID: PMC4392391
Effect of pneumococcal conjugate vaccination on serotype-specific carriage and invasive disease in England: a cross-sectional study.
PLoS Med, 8(4):e1001017, 05 Apr 2011
Cited by: 184 articles | PMID: 21483718 | PMCID: PMC3071372
Interim results of an ecological experiment - Conjugate vaccination against the pneumococcus and serotype replacement.
Hum Vaccin Immunother, 12(2):358-374, 01 Jan 2016
Cited by: 66 articles | PMID: 26905681 | PMCID: PMC5049718
Review Free full text in Europe PMC
Funding
Funders who supported this work.
NIAID NIH HHS (4)
Grant ID: R01 AI066013-05
Grant ID: R01 AI048935
Grant ID: R01 AI048935-10
Grant ID: R01 AI066013