Abstract
Free full text

Mechanistic coupling of protease signaling and initiation of coagulation by tissue factor
Abstract
The crucial role of cell signaling in hemostasis is clearly established by the action of the downstream coagulation protease thrombin that cleaves platelet-expressed G-protein-coupled protease activated receptors (PARs). Certain PARs are cleaved by the upstream coagulation proteases factor Xa (Xa) and the tissue factor (TF)–factor VIIa (VIIa) complex, but these enzymes are required at high nonphysiological concentrations and show limited recognition specificity for the scissile bond of target PARs. However, defining a physiological mechanism of PAR activation by upstream proteases is highly relevant because of the potent anti-inflammatory in vivo effects of inhibitors of the TF initiation complex. Activation of substrate factor X (X) by the TF–VIIa complex is here shown to produce enhanced cell signaling in comparison to the TF–VIIa complex alone, free Xa, or Xa that is generated in situ by the intrinsic activation complex. Macromolecular assembly of X into a ternary complex of TF–VIIa–X is required for proteolytic conversion to Xa, and product Xa remains transiently associated in a TF–VIIa–Xa complex. By trapping this complex with a unique inhibitor that preserves Xa activity, we directly show that Xa in this ternary complex efficiently activates PAR-1 and -2. These experiments support the concept that proinflammatory upstream coagulation protease signaling is mechanistically coupled and thus an integrated part of the TF–VIIa-initiated coagulation pathway, rather than a late event during excessive activation of coagulation and systemic generation of proteolytic activity.
Thrombin is a major mediator of cell-signaling events by specifically recognizing and cleaving three members of a family of closely related G-protein-coupled receptors, namely protease-activated receptor (PAR)-1, -3, and -4 (1). Work from a number of laboratories has provided compelling evidence that the upstream coagulation protease factor VIIa (VIIa) and factor Xa (Xa) can elicit similar PAR-mediated proinflammatory cell signaling events (2–7), and anti-inflammatory in vivo effects of inhibitors of VIIa activity or Xa generation strongly suggest relevance of upstream protease signaling (8). VIIa can activate the thrombin insensitive PAR-2 (9), and Xa has been shown to activate PAR-1 and -2 (7, 9, 10). In contrast to thrombin that binds to a recognition sequence in close proximity to the scissile bond in PAR-1 or -3, the driving force for PAR cleavage by VIIa or Xa may be cell surface localization through cofactor or Gla-domain-mediated membrane recruitment. The requirement for membrane binding may explain why the activation of PAR-1 or -2 by Xa was observed only at relatively high concentrations of ≈10 nM and more (7, 9, 10). This concentration is much higher than levels of ≈1 nM Xa that are sufficient to maintain maximal thrombin generation in simulations of the coagulation pathway in vitro (11). An unresolved issue has thus been how signaling-relevant concentrations of upstream coagulation proteases can be generated under in vivo conditions.
Tissue factor (TF) plays the key role in the cellular initiation of the coagulation protease cascade by serving as the cell surface receptor for the zymogen factor VII (VII) and its activated form VIIa. TF functions as an enzymatic cofactor by stabilizing the active conformation of the VIIa protease domain (12) and, most importantly, by contributing to an extended recognition interface for the substrate X (13). These exosite interactions determine macromolecular substrate specificity and affinity (14, 15). Exosite docking does not interfere with small substrate binding to the active site of VIIa. Thus, X activation is likely a two-step reaction in which exosite docking precedes scissile bond cleavage by VIIa. Product Xa retains significant affinity for TF–VIIa (15), and indirect evidence for a significant biological half-life of this transient ternary complex is the fact that TF pathway inhibitor (TFPI) (16, 17) and nematode-derived proteins (18) have evolved to simultaneously bind Xa and the active site of VIIa to form a higher order complex based on the ternary TF–VIIa–Xa complex. Furthermore, the ternary complex on cell surfaces has sufficient half-life that allows for trafficking of TF between different membrane subdomains (19).
Here, we provide evidence for a role of this ternary complex in cell signaling. Kinetic analysis of the relationship between Xa generation and ensuing cell signaling demonstrates that Xa signaling occurs at low concentrations of Xa that are typical for the early initiation phase of coagulation. With a unique inhibitor that inhibits catalytic activity of free Xa but that maintains an active conformation of Xa when in a ternary TF–VIIa–Xa complex, we further directly demonstrate that Xa signals in the initiation complex through PAR-1 and -2. Proinflammatory effects of upstream coagulation proteases thus do not require excessive generation of free proteolytic activity. Rather, our data support the concept that cell signaling is an integrated part of the biochemical mechanism of substrate activation by TF–VIIa, linking the initiation of coagulation inseparably to inflammatory autocrine cell signaling.
Materials and Methods
Proteins, Antibodies, and Inhibitors.
Thrombin, recombinant VII and VIIa, catalytically inactive Ser195 to Ala mutated VIIa (iVIIa), factor IXa, and PAR agonist peptides TFLLRNPNDK and SLIGRL, were as described (7, 14, 20). Monoclonal antibodies 12C7 and 12D10 (21), 5G9, 6B4, and 9C3 (22), ATAP2, and WEDE15 (23) have been characterized previously. Polyclonal anti-TFPI antibodies were raised in rabbits against recombinant TFPI (24). Recombinant nematode anticoagulant proteins 5 (NAP5) (25) and c2 (NAPc2) (18) and the Kunitz type VIIa inhibitor 5L15 (26) were kindly provided by G. Vlasuk (Corvas International, San Diego, CA). Human factor VIIIa (27) was kindly provided by P. Fay (University of Rochester, Rochester, NY). Human Xa was from Hematologic Technologies (Essex Junctions, VT) and hirudin from Calbiochem.
Cell Culture.
Chinese hamster ovary (CHO) cells expressing TF (CHO/TF cells) (28) were transfected with the human PAR-2 cDNA [American Type Culture Collection (ATCC) 99516] (29) in pcDNA3.1/Zeo (Invitrogen), and stable clones were selected for PAR-2 agonist SLIGRL-induced mitogen-activated protein kinase (MAPK) phosphorylation. The human umbilical vein endothelial cell line HUV-EC-C (ATCC CRL 1730) was grown in complete endothelial cell growth medium (Clonetics, Walkersville, MD) containing 5% FCS. Cells were split 1:3 into 2% FCS medium for 48 h and switched to serum-free M199 1× Earle's salt (Irvine Scientific) with 10 mM Hepes/2 mM Ca2+ with or without 5 nM tumor necrosis factor α (TNFα) for 5 h.
Analysis of MAPK Phosphorylation and Northern Blotting.
Cells were serum-deprived overnight. Before agonist stimulation, hirudin (100 nM) was added in all experiments involving cell activation with VIIa or Xa to rule out signaling from downstream-generated thrombin, and HUV-EC-Cs were also preincubated with 15 μg/ml of polyclonal anti-TFPI for 10 min. Cells were stimulated for the indicated times, followed by Western blotting for MAPK phosphorylation or by RNA extraction and Northern blotting, as described (7).
Reporter Gene Assay.
The spontaneously transformed M6–11 fibroblast cell line from PAR-1-deficient mice was kindly provided by Patricia Andrade-Gordon (R. W. Johnson Pharmaceutical Research Institute, Spring House, PA). Cells were grown in low-glucose DMEM/2 mM l-glutamine/10% FCS. Cells were transiently transfected by using GenePORTER reagent (Gene Therapy Systems, San Diego) with expression constructs for human PAR-2 and TF, as well as a reporter construct containing 1.2 kb of mouse egr-1 5′ flanking sequence upstream of the luciferase reporter gene in the promoterless pXP2 (30). Twenty-four hours after transfection, cells were serum-starved for 16 h followed by agonist stimulation and a 5-h incubation to allow for expression of the luciferase protein. Luciferase activity in the cell lysate was determined by using the Luciferase Assay System (Promega) and a Monolight 2010 luminometer (Analytical Luminescence Laboratory, San Diego).
Results
Enhanced PAR Signaling by TF-Initiated Xa Generation.
CHO/TF cells (28) were used to analyze the role of TF in orchestrating protease signaling events. Induction of MAPK phosphorylation in serum-starved cells showed that CHO/TF cells were unreactive to PAR-2 agonist peptides but were responsive to thrombin and the PAR-1 specific agonist peptide TFLLRNPNDK (31), consistent with expression of hamster PAR-1 (Fig. (Fig.11A). CHO/TF cells stably transfected with a human PAR-2 expression construct gained responsiveness to the PAR-2 agonist peptide SLIGRL (Fig. (Fig.11A), and two independent clonal lines behaved indistinguishably in all experiments described below. Only CHO/TF/PAR-2 cells were responsive to VIIa at a high concentration that was used to assure rapid saturation of TF in this short-term assay. In contrast, high concentrations of Xa produced a similar induction of MAPK phosphorylation in CHO/TF and CHO/TF/PAR-2 clones. Thus, PAR-2 expression in CHO/TF cells was required to confer responsiveness to TF-VIIa, without producing synergistic effects in Xa signaling in this or any of the following experiments.
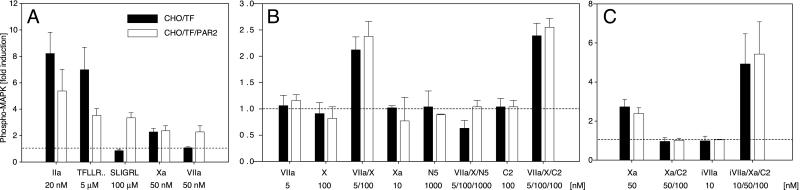
Protease signaling-mediated MAPK phosphorylation in TF-transfected CHO cells. (A–C) Confluent CHO/TF- (solid bars) or PAR-2-transfected CHO/TF/PAR2 (open bars) cells were serum starved and stimulated for 10 min with the indicated concentrations of agonists. Where indicated, specific inhibitors NAP5 (N5) or NAPc2 (C2) and active site-mutated VIIa (iVIIa) were added 2 min before agonist stimulation. MAPK phosphorylation was quantified by Western blotting, and fold induction over unstimulated control was calculated on the basis of densitometric scans. Means and standard deviations are shown (A, n = 4; B and C, n = 3).
Signaling incompetent concentrations of VIIa are known to elicit PAR-2 signaling by rapidly converting a significant portion of X added at plasma concentrations (9). Addition of low concentrations of VIIa and near plasma concentrations of zymogen X also triggered MAPK phosphorylation in the CHO-cell model (Fig. (Fig.11B). During the 10-min assay period, 8 ± 2 nM Xa (mean ± SD, n = 3) was generated, but stimulation of cells with 10 nM Xa for the entire 10 min failed to induce MAPK phosphorylation. Simultaneous addition of VIIa and X may enhance signaling of VIIa or the product Xa. Because substrate increases the affinity of VIIa for TF, PAR cleavage by VIIa may be increased in the presence of X. However, that CHO/TF and CHO/TF/PAR2 cells were similarly responsive to the combination of VIIa and X and that the specific Xa active site inhibitor NAP5 abolished signaling by VIIa–X argue against this possibility. One possible mechanism for enhanced signaling of Xa generated by TF–VIIa is transient association of product with the membrane surface on which Xa is formed. If this were the case, Xa generation by the intrinsic factor VIIIa–IXa complex should produce a comparable signaling response. Generation of 2.9 ± 0.9 nM Xa by 5 nM VIIIa–IXa resulted in 0.9 ± 0.3-fold induction of MAPK phosphorylation, as compared with a 2.0 ± 0.6-fold induction by TF–VIIa that generated 3.4 ± 0.8 nM Xa in parallel reactions (n = 3). Thus local generation of Xa is not sufficient to increase the efficiency of Xa signaling. Rather, these data suggest that the biochemical mechanism of Xa generation by TF–VIIa promotes efficient PAR signaling by Xa.
Signaling of the NAPc2 Stabilized TF–VIIa–Xa Complex.
To manipulate TF–VIIa-dependent X activation, we used another nematode-derived inhibitor, NAPc2, that has a complex inhibitory mechanism. NAPc2 binds to both X and Xa at a high-affinity exosite that is outside of the catalytic cleft and utilizes X or Xa as a scaffold to associate with the TF–VIIa complex. The inhibitor domain of NAPc2 then binds to the active site of VIIa, resulting in a tight TF–VIIa–Xa–NAPc2 complex in which VIIa is inactive, but the catalytic cleft of Xa remains accessible for binding of other protease inhibitors (18). NAPc2 inhibited TF–VIIa-dependent cell surface Xa generation by 90% under our experimental conditions. However, the 10-fold reduction in the amount of Xa generation did not reduce MAPK phosphorylation in response to VIIa–X (Fig. (Fig.11B).
A possible explanation for this paradoxical effect of NAPc2 is stabilization of a signaling competent ternary TF–VIIa–Xa complex. In addition, NAPc2 may have allosteric effects on Xa's catalytic activity, and this possibility was addressed by analyzing the cleavage of the chromogenic substrate Spectrozyme FXa (American Diagnostica, Greenwich, CT). Xa (2 nM) amidolytic activity was analyzed in the presence of relipidated TF (5 nM) to mimic the TF-expressing cell surface. Addition of NAPc2 (10 nM) inhibited Xa amidolytic activity to a level of 16 ± 1% (mean ± SD, n = 3) of control. To analyze the activity of Xa when localized by NAPc2 to TF–VIIa, we used catalytic triad mutated iVIIa, which lacks catalytic activity but has the active site accessible for NAPc2 interaction. Biacore analysis showed that this mutant indeed formed a high-affinity stable complex with NAPc2–Xa in the presence of full-length TF (data not shown). Addition of iVIIa (5 nM) to TF (5 nM), NAPc2 (10 nM), and Xa (2 nM) resulted in Xa amidolytic activity of 114 ± 7% of Xa's activity in the absence of NAPc2. Thus, NAPc2 inhibits catalytic activity of free Xa, but the allosteric inhibition is reversed on formation of the TF–VIIa–Xa–NAPc2 complex. As predicted from these data, NAPc2 efficiently blocked PAR signaling by high concentrations of free Xa (Fig. (Fig.11C). Addition of the signaling incompetent iVIIa significantly enhanced signaling of Xa–NAPc2 to levels higher than those achieved with high concentrations of free Xa in the absence of NAPc2 (Fig. (Fig.11C). Thus, NAPc2 indeed stabilized the TF–VIIa–Xa complex in a conformation that preserved the activity of Xa for PAR activation.
To further characterize signaling by the NAPc2-stabilized TF–VIIa–Xa complex, the dose requirement for Xa was established (Fig. (Fig.22A). In this short-term assay, up to 30 nM of free Xa produced little response, whereas NAPc2-mediated recruitment of 3–10 nM Xa to the TF–VIIa complex induced MAPK phosphorylation that approached the responses of agonist peptides. Signaling at these concentrations of Xa is consistent with the ≈6 nM dissociation constant of TF–VIIa–Xa–NAPc2 complex formation on neutral phospholipid (18). Antibody inhibition experiments were performed to confirm that the NAPc2-stabilized complex has the characteristics of the ternary TF–VIIa–Xa complex that exists transiently before product dissociation. The noninhibitory monoclonal anti-VIIa 12C7 was without effect on complex signaling, but TF–iVIIa–Xa–NAPc2 signaling was inhibited with antibodies that block X substrate interaction with TF–VIIa either by binding to the VIIa protease domain (anti-VIIa 12D10) (21) or by binding to the X-binding exosite site on TF (anti-TF 5G9) (32). Similarly, blocking the VIIa-binding site on TF by a combination of anti-TF 6B4 and 9C3 also prevented signaling, emphasizing the importance of iVIIa ligand binding to TF. Thus, the NAPc2-stabilized complex has the characteristics of the coagulation initiation complex, and Xa in this complex produces more efficient signaling than free Xa or the TF–VIIa complex.
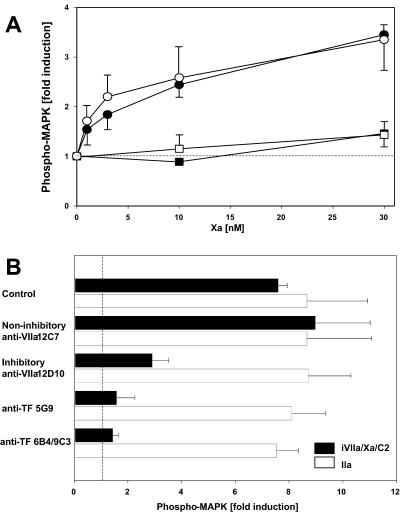
(A) Dose response of Xa-induced MAPK phosphorylation. Serum-starved CHO/TF cells (solid symbols) or CHO/TF/PAR2 cells (open symbols) were stimulated (10 min) with the indicated concentrations of Xa alone (squares) or Xa in the presence of iVIIa (10 nM) and NAPc2 (100 nM) (circles). (B) Effect of inhibitors of ternary TF–VIIa–Xa complex formation on iVIIa/Xa/NAPc2-induced signaling in CHO/TF/PAR2 cells. Serum-starved cells were preincubated (10 min) with no antibody (control) or monoclonal anti-VIIa 12C7 (50 μg/ml), anti-VIIa 12D10-Fab (25 μg/ml), anti-TF 5G9 (50 μg/ml), or anti-TF 6B4 (25 μg/ml)/9C3 (25 μg/ml). Cells were stimulated (10 min) with iVIIa/Xa/NAPc2 (10/50/100 nM) (solid bars) or 10 nM thrombin (open bars). Induction of MAPK phosphorylation is shown in A and B as fold induction over control (mean ± SD, n = 3).
PAR-2 Activation by the Ternary TF–VIIa–Xa Complex.
Because of lack of suitable reagents to block PAR-1 signaling in the CHO cell model, it remained unclear whether complexed Xa was capable of signaling through PAR-2. PAR-1 and -2 agonist peptide unresponsive spontaneously transformed fibroblasts from PAR-1 knockout mice were used for a gene transcription reporter assay that measured the induction of the promoter of the zinc-finger transcription factor EGR-1, known to be downstream of protease signaling (4). Cells were cotransfected with TF and PAR-2 cDNA constructs and a construct containing the luciferase gene under the control of the mouse EGR-1 promoter. Overnight serum-starved cells were activated for 5 h. Responsiveness to PAR-2 agonist peptide and to 10 nM VII or VIIa demonstrates that expression of PAR-2 and TF was achieved under these conditions (Fig. (Fig.33A). VIIa signaling was not blocked by the Xa inhibitor NAP5, but NAP5 inhibited Xa (20 nM) and also iVIIa/Xa/NAPc2 (10/5/50 nM) responses (Fig. (Fig.33A). As predicted, low concentrations of Xa (0.6–2.5 nM) produced only a signal when recruited by NAPc2 to the TF–iVIIa complex (Fig. (Fig.33B). The 5-h stimulation period clearly excluded that the failure of low concentrations of free Xa to stimulate cells is because of a slow association of the enzyme with cell surface-binding sites. Thus, Xa can efficiently induce gene expression only in the coagulation initiation complex with TF–VIIa. Addition of iVIIa together with Xa in the absence of NAPc2 enhanced signaling marginally at the higher Xa concentration (Fig. (Fig.33B), as expected from the ≈5-fold lower affinity of Xa for TF–VIIa as compared with substrate X (15). Under physiological conditions, the plasma concentration of X governs the assembly with TF–VIIa, and the dissociation rate of product, rather than overall affinity, determines whether a ternary TF–VIIa–Xa complex is present.
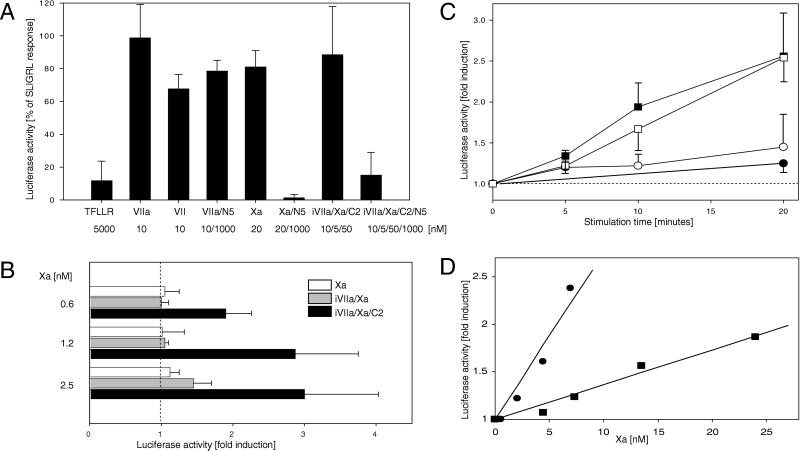
PAR-2-dependent induction of EGR-1 promoter activity. (A) Serum-starved PAR-1-deficient fibroblasts, transfected with human PAR-2 and TF, as well as an EGR-1 promoter luciferase reporter construct, were incubated for 5 h in the presence of agonists. PAR-2-activating peptide SLIGRL (100 μM) induced luciferase activity 3.9 ± 0.6-fold (mean ± SD, n = 3) over control. Gene induction by the indicated agonist and in the presence of inhibitors NAP5 (N5) or NAPc2 (C2) is shown as percent of the SLIGRL response (mean ± SD, n = 3). (B) Dose response of Xa in transfected fibroblasts. Fold induction of luciferase activity is shown for cells stimulated (5 h) with the indicated concentrations of Xa alone (open bars), Xa in the presence of 10 nM iVIIa (gray bars), or Xa with 10 nM iVIIa/50 nM NAPc2 (solid bars) (mean ± SD, n = 3). (C) Time requirement for protease signaling input in transfected cells stimulated with 10 nM VII (●), 10 nM VII/100 nM X (□), 10 nM VII/100 nM X in the presence of 1 μM NAP5 (○), or 10 nM iVIIa/2 nM Xa/5 nM NAPc2 (■). Proteases were inhibited at the indicated times with 1 μM 5L15 (added to all reactions with VII) and 1 μM NAP5 (added to all reactions with X/Xa), followed by determination of luciferase activity after 5 h (mean ± SD, n = 3). Fold induction by SLIGRL was 3.1 ± 0.6 in these experiments. (D) Dose response of EGR-1 promoter induction by in situ-generated Xa versus exogenously added Xa. On cells incubated with 10 nM VII and 100 nM X, the amount of Xa generated in situ was manipulated by blocking VIIa with 1 μM 5L15 after 0, 2, 5, 10, 20, or 30 min. Xa concentrations were determined by amidolytic assay for all reactions at 30 min, followed by termination of signaling input with 1 μM NAP5 at 30 min. The plots show gene induction in dependence of the concentration of exogenously added Xa (■) or in situ-generated Xa (●). A typical experiment of three is shown. Control experiments with Xa generated by the intrinsic activation complex did not show enhanced signaling. Xa (12 ± 1 nM) generated by 2 nM VIIIa–IXa in 20 min produced a 1.3 ± 0.2-fold induction of luciferase activity, as compared with a 1.6 ± 0.7-fold induction by 12 ± 2 nM free Xa (n = 3).
Signaling in the Initiation Phase of Coagulation.
The signaling that is produced by the initiation phase of coagulation was further explored in the EGR-1 reporter assay system. Because the accumulation of the luciferase protein is necessary for the assay readout, we used specific inhibitors of VIIa and Xa to interfere with protease-mediated cell signaling at early times. Signaling of the NAPc2-stabilized TF–iVIIa–Xa complex was stopped after 5, 10, or 20 min with NAP5, and luciferase activity was determined after 5 h (Fig. (Fig.33C, solid squares). Stimulation for 20 min was as effective as a 5-h stimulation, demonstrating that the stabilized complex produces an early and efficient signaling input. VII (10 nM) did not induce significant luciferase activity when VIIa activity was blocked with 5L15 after 20 min (Fig. (Fig.33C, solid circle), indicating that response to VII/VIIa seen in Fig. Fig.33A is the result of a slow and accumulative cell signaling input. Lack of signaling on incubation with VII and X in the presence of NAP5 (Fig. (Fig.33C, open circles) ruled out that VII may require the presence of near physiological concentrations of substrate X for efficient recruitment to TF and resulting TF–VIIa cell signaling. In contrast, when cells were stimulated by VII and X followed by simultaneous inhibition of VIIa and Xa after 10 or 20 min, signaling occurred with essentially the same magnitude as observed for the NAPc2-stabilized ternary complex (Fig. (Fig.33C, open squares). Under these conditions, <5% of substrate X was activated, and free Xa did not reach a signaling threshold in this assay. Activation with near physiological concentrations of VII–X produced a signal that was similar to the NAPc2-stabilized TF–VIIa–Xa, indicating that during initiation of coagulation the cell surface half-life of the product TF–VIIa–Xa complex is long relative to the half life of the substrate TF–VIIa–X complex.
To characterize the dose-response for Xa signaling during the initiation phase, we used the VIIa active site inhibitor 5L15 to manipulate the amount of in situ-generated Xa. Xa generation was blocked by adding 5L15 after 2, 5, 10, 20, or 30 min. At the 30-min time point, aliquots were removed to determine the amount of Xa in the cell supernatant by amidolytic assay, and NAP5 was added to block any further cell signaling input by Xa. Cells were also incubated with increasing concentrations of Xa for a 30-min stimulation, and the supernatant from these wells was similarly assayed for Xa by amidolytic assay. Fig. Fig.33D shows gene induction in dependence of the amount of Xa present at 30 min for exogenously added Xa or in situ generated Xa. Very low concentrations of in situ-generated Xa produced agonist responses that were higher than the ones obtained with the tested concentrations of exogenously added free Xa. The slopes of the presented dose-response curves indicate a 5.1 ± 0.5 (mean ± SD, n = 3) increase in signaling efficiency for Xa in the initiation phase reaction, as compared with free Xa.
Xa Initiation Phase Signaling Activates TNFα-Stimulated Endothelial Cells.
Endothelial cells are possible in vivo targets of proinflammatory effects of upstream coagulation protease signaling. We analyzed signaling of the NAPc2-stabilized TF–iVIIa–Xa complex in HUV-EC-Cs that are known to express TF in response to TNFα. All experiments were performed in the presence of an inhibitory antibody to TFPI, because TFPI was found to variably interfere with protease-mediated endothelial cell activation. Both noninduced and TNFα-induced HUV-EC-Cs phosphorylated MAPK in response to PAR-1 and -2 agonist peptides (Fig. (Fig.44A). The SLIGRL response was slightly higher in the TNFα-treated cells, consistent with the known up-regulation of PAR-2 by TNFα in endothelial cells (33). Xa (50 nM) showed robust induction of phosphorylated MAPK in both unstimulated and stimulated cells, whereas VIIa (50 nM) was without major effects under our experimental conditions. Cell activation on incubation with iVIIa/Xa/NAPc2 was detected only in TNFα-stimulated cells, consistent with the requirement of TF for assembly of the signaling complex (Fig. (Fig.44A). Addition of iVIIa or Xa–NAPc2 alone was without effect, and signaling of the NAPc2-stabilized ternary complex in endothelial cells was inhibited by NAP5 (Fig. (Fig.44B), as predicted from the overexpression systems described above.
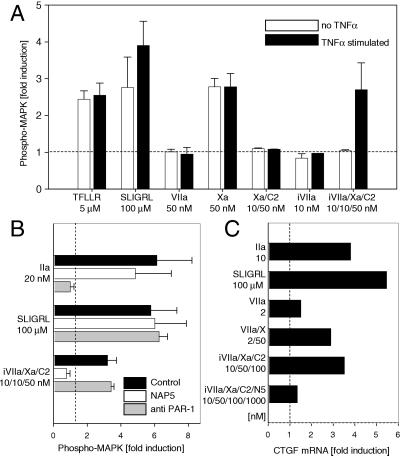
Upstream coagulation protease signaling in endothelial cells. (A) MAPK phosphorylation in endothelial cells on ternary TF–VIIa–Xa complex signaling. HUV-EC-Cs were serum starved for 5 h in the absence (open bars) or presence (solid bars) of 5 nM TNFα followed by 10-min stimulation with the indicated agonists. Fold induction of phosphorylated MAPK is shown (mean ± SD, n = 3). (B) Role of PAR-1 in TF–VIIa–Xa complex signaling in TNFα-stimulated endothelial cells. MAPK phosphorylation on thrombin, SLIGRL, or TF–iVIIa–Xa–NAPc2 activation for 10 min is shown in the absence of inhibitors (control; black bars), the presence of 1 μM NAP5 (open bars), or with 10 μg/ml of ATAP2 and 25 μg/ml of WEDE15 (anti-PAR-1; gray bars). (C) Gene induction by the coagulation initiation complex in endothelial cells. HUV-EC-C cells were serum starved for 12 h in the presence of 5 nM TNFα and stimulated for 60 min with the indicated agonists. Induction of connective tissue growth factor mRNA expression by Northern blotting was normalized to the housekeeping gene GAPDH (7).
Because endothelial cells express both PAR-1 and -2, it was of interest to analyze the role of PAR-2 in ternary complex signaling under conditions where PAR-2 is expressed endogenously and not by forced overexpression, as in the fibroblast model. Human PAR-1 activation can be inhibited by cleavage-blocking antibodies (7, 23), which efficiently abolished thrombin-mediated MAPK phosphorylation in endothelial cells (Fig. (Fig.44B). Anti-PAR-1 antibodies had no effect on iVIIa/Xa/NAPc2 signaling in TNFα-stimulated HUV-EC-Cs, demonstrating that PAR-2 is sufficient to mediate Xa signaling in the TF–iVIIa complex on a cell type of physiological relevance. Connective tissue growth factor (CTGF) is an endothelial cell-expressed gene, which has been identified as a target for upstream coagulation protease signaling (5, 7). Northern blotting demonstrated that CTGF is induced by Xa in the NAPc2-stabilized TF–iVIIa–Xa complex, as well as by initiation complex signaling (Fig. (Fig.44C), providing evidence that relevant downstream signaling events occur during initiation of coagulation on endothelial cells.
Discussion
Using three different downstream signaling events, we demonstrate that Xa at low nanomolar concentrations signals when associated with TF–VIIa, either through stable recruitment by the inhibitor NAPc2 or in the course of substrate conversion by the cell surface initiation complex. At physiological concentrations, VIIa and X rapidly assemble with TF into the ternary substrate complex TF–VIIa–X. The rate-limiting step in small substrate hydrolysis by TF–VIIa is deacylation (34), and cleavage of the scissile bond of X in the ternary substrate complex is expected to be fast (Fig. (Fig.5).5). Notably, in the intermediate acylenzyme TF–VIIa–Xa complex, Xa is already active for inflammatory cell signaling, whereas the acylenzyme VIIa cannot cleave PARs. NAPc2 inactivates VIIa, but not Xa, and the NAPc2-stabilized ternary complex thus mimics aspects of the acylenzyme intermediate complex. The NAPc2-stabilized ternary complex and the initiation reaction produced equivalent cell signaling. We conclude from these data that the ternary complex during initiation of coagulation on the cell surface has sufficient half-life to activate PARs. Although product Xa release disrupts ternary TF–VIIa–Xa complex cell signaling, rapid rebinding of macromolecular substrate followed by scissile bond cleavage can restore a signaling competent TF–VIIa–Xa complex. Because the stabilized ternary complex was localized in a signaling permissive cell surface microenvironment, we infer that the transient ternary complex localizes to the same compartment during the initiation phase of coagulation.
Activation of the coagulation cascade in Gram-negative septicemia triggers diverse thrombotic and inflammatory events. A proinflammatory signaling function of the upstream coagulation cascade has long been suspected from the effect of coagulation inhibitors in animal models of septicemia. In contrast to inhibition of thrombin generation by the prothrombinase inhibitor active site-blocked Xa, which essentially cured thrombotic complications of sepsis but failed to reduce mortality (35), inhibitors of the TF initiation complex, i.e., antibodies to TF (36), TFPI (37), or active site-blocked VIIa (38) all reduced inflammatory responses in addition to their antithrombotic effects. Moreover, it appears that therapeutic efficacy toward thrombotic complications is much more readily achieved than the anti-inflammatory benefits (39). These data indicate that the excessive activation of free proteolytic activity is not the crucial pathophysiological mechanism that drives inflammation. Signaling of the ternary TF–VIIa–Xa initiation complex provides a mechanism to activate PAR-1 and -2 without requiring massive activation of fluid-phase proteases that drive disseminated intravascular coagulation. In addition, fluid-phase inhibition by the plasma serine protease inhibitors, e.g., antithrombin III, is at a kinetic disadvantage if PAR signaling can occur concomitantly with product generation at the TF–VIIa complex. Inhibition of ternary complex cell signaling must be most efficient by the inhibitor that targets the initiation complex, i.e., TFPI. Breakdown of inhibitory control by TFPI, e.g., by leukocyte elastase (40) or metalloproteinases (41), may thus represent a crucial early event that determines the progression of septic syndromes through upstream coagulation protease signaling.
With limited sequence complementarity of PAR-1 and -2 with the catalytic cleft of upstream coagulation proteases, the distance of the PAR scissile bond from the membrane may be a crucial factor for the efficiency of cleavage. The specific interactions of TF with enzyme VIIa versus product Xa may position the active site of Xa in a more favorable orientation for the activation of PARs, as compared with VIIa. This may explain the apparently less efficient activation of PAR-2 by TF–VIIa. The transient ternary TF–VIIa–Xa complex is a unique example of a self-limiting signaling input through PARs. Because its half-life is determined by the dissociation rate of product from the complex once substrate delivery ceases, the signaling event is closely linked to the ongoing activation of the coagulation cascade. Whereas protease signaling has long been appreciated as part of the hemostatic response by providing the crucial activation of platelets by thrombin, a different concept emerges for the upstream coagulation reaction. On TF-expressing cells, autocrine or, in specific settings of cell–cell interaction, possibly paracrine, cell signaling is not a secondary event resulting from the excessive activation of proteases. Rather activation of inflammatory signaling pathways is an integrated part of and inseparably tied to the cellular initiation of coagulation by TF. Intriguingly, the protein C pathway, which is a major anticoagulant regulator of the downstream coagulation reaction, has recently been recognized as an important anti-inflammatory mediator through upstream receptor-mediated cell signaling effects (8) that have successfully been exploited to treat sepsis (42). In its evolution from a structural member of the cytokine receptor family, TF has thus not parted from its heritage as an inflammatory receptor by generating a mechanism to localize at least one of its ligands for efficient cell signaling functions, without interfering with its acquired function of initiation of the downstream coagulation cascade.
Acknowledgments
We thank Jennifer Royce, Pablito Tejada, and Aaron Donner for excellent technical assistance, and Drs. G. Vlasuk, P. Andrade-Gordon, L. Brass, and P. Fay for invaluable reagents. These studies were supported by National Institutes of Health Grants HL 16411 and HL 48752, by a fellowship from International Society for Thrombosis and Hemostasis (M.R.), and by an Established Investigator Award from the American Heart Association (W.R.).
Abbreviations
CHO | Chinese hamster ovary |
CHO/TF | TF-expressing CHO cells |
NAPc2 | nematode anticoagulant protein C2 |
NAP5 | nematode anticoagulant protein 5 |
PAR | protease-activated receptor |
TF | tissue factor |
TFPI | TF pathway inhibitor |
TNFα | tumor necrosis factor α |
VII/VIIa | factor VII/VIIa |
iVIIa | Ser195 to Ala mutated VIIa |
X/Xa | factor X/Xa |
MAPK | mitogen-activated protein kinase |
Footnotes
This paper was submitted directly (Track II) to the PNAS office.
References
Articles from Proceedings of the National Academy of Sciences of the United States of America are provided here courtesy of National Academy of Sciences
Full text links
Read article at publisher's site: https://doi.org/10.1073/pnas.141126698
Read article for free, from open access legal sources, via Unpaywall:
https://europepmc.org/articles/pmc35412?pdf=render
Citations & impact
Impact metrics
Citations of article over time
Alternative metrics
Smart citations by scite.ai
Explore citation contexts and check if this article has been
supported or disputed.
https://scite.ai/reports/10.1073/pnas.141126698
Article citations
Targeting the tissue factor coagulation initiation complex prevents antiphospholipid antibody development.
Blood, 143(12):1167-1180, 01 Mar 2024
Cited by: 1 article | PMID: 38142429
Coagulation Factor Xa Has No Effects on the Expression of PAR1, PAR2, and PAR4 and No Proinflammatory Effects on HL-1 Cells.
Cells, 12(24):2849, 15 Dec 2023
Cited by: 3 articles | PMID: 38132169 | PMCID: PMC10741780
Peripheral blood mononuclear cell tissue factor (F3 gene) transcript levels and circulating extracellular vesicles are elevated in severe coronavirus 2019 (COVID-19) disease.
J Thromb Haemost, 21(3):629-638, 22 Dec 2022
Cited by: 15 articles | PMID: 36696180 | PMCID: PMC9773443
Manipulation of tissue factor-mediated basal PAR-2 signalling on macrophages determines sensitivity for IFNγ responsiveness and significantly modifies the phenotype of murine DTH.
Front Immunol, 13:999871, 12 Sep 2022
Cited by: 3 articles | PMID: 36172348 | PMCID: PMC9510775
Cancer-related stroke: Exploring personalized therapy strategies.
Brain Behav, 12(9):e2738, 08 Aug 2022
Cited by: 4 articles | PMID: 35938982 | PMCID: PMC9480895
Review Free full text in Europe PMC
Go to all (187) article citations
Similar Articles
To arrive at the top five similar articles we use a word-weighted algorithm to compare words from the Title and Abstract of each citation.
Orchestration of coagulation protease signaling by tissue factor.
Trends Cardiovasc Med, 12(4):149-154, 01 May 2002
Cited by: 51 articles | PMID: 12069753
Review
Regulation of tissue factor-induced signaling by endogenous and recombinant tissue factor pathway inhibitor 1.
Blood, 105(6):2384-2391, 18 Nov 2004
Cited by: 58 articles | PMID: 15550483
Ligand-induced protease receptor translocation into caveolae: a mechanism for regulating cell surface proteolysis of the tissue factor-dependent coagulation pathway.
J Cell Biol, 133(2):293-304, 01 Apr 1996
Cited by: 117 articles | PMID: 8609163 | PMCID: PMC2120798
Tissue factor residues Lys165 and Lys166 are essential for rapid formation of the quaternary complex of tissue factor.VIIa with Xa.tissue factor pathway inhibitor.
Biochemistry, 34(34):10867-10871, 01 Aug 1995
Cited by: 12 articles | PMID: 7662667
Funding
Funders who supported this work.
NHLBI NIH HHS (4)
Grant ID: HL 16411
Grant ID: R01 HL048752
Grant ID: HL 48752
Grant ID: P01 HL016411