Abstract
Free full text
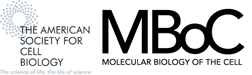
Estradiol and Selective Estrogen Receptor Modulators Differentially Regulate Target Genes with Estrogen Receptors α and β
Abstract
Estrogens and selective estrogen receptor modulators (SERMs) interact with estrogen receptor (ER) α and β to activate or repress gene transcription. To understand how estrogens and SERMs exert tissue-specific effects, we performed microarray analysis to determine whether ERα or ERβ regulate different target genes in response to estrogens and SERMs. We prepared human U2OS osteosarcoma cells that are stably transfected with a tetracycline-inducible vector to express ERα or ERβ. Western blotting, immunohistochemistry, and immunoprecipitation studies confirmed that U2OS-ERα cells synthesized only ERα and that U2OS-ERβ cells expressed exclusively ERβ. U2OS-ERα and U2OS-ERβ cells were treated either with 17β-estradiol (E2), raloxifene, and tamoxifen for 18 h. Labeled cRNAs were hybridized with U95Av2 GeneChips (Affymetrix). A total of 228, 190, and 236 genes were significantly activated or repressed at least 1.74-fold in U2OS-ERα and U2OS-ERβ cells by E2, raloxifene, and tamoxifen, respectively. Most genes regulated in ERα cells in response to E2, raloxifene, and tamoxifen were distinct from those regulated in ERβ cells. Only 38 of the 228 (17%) genes were regulated by E2 in both U2OS-ERα and U2OS-ERβ cells. Raloxifene and tamoxifen regulated only 27% of the same genes in both the ERα and ERβ cells. A subset of genes involved in bone-related activities regulated by E2, raloxifene, and tamoxifen were also distinct. Our results demonstrate that most genes regulated by ERα are distinct from those regulated by ERβ in response to E2 and SERMs. These results indicate that estrogens and SERMs exert tissue-specific effects by regulating unique sets of targets genes through ERα and ERβ
INTRODUCTION
The decline of estrogen levels during menopause is associated with a variety of conditions, including hot flushes, mood swings, vaginal dryness, and accelerated bone loss (Johnson, 1998). In an attempt to prevent these conditions, postmenopausal women are often treated with estrogens in the form of hormone replacement therapy (HRT) (Johnson, 1998). Clinical trials proved that estrogens are effective at relieving menopausal symptoms and preventing osteoporosis (Writing Group for PEPI Trial, 1996; Torgerson, 2000). The randomized, placebo-controlled Women's Health Initiative Trial confirmed that HRT decreases the risk of fractures, but it was terminated early because an increased risk of breast cancer and cardiovascular disease was observed (Writing Group for Women's Health Initiative, 2002).
The adverse effects of estrogens has inspired an intense pursuit to develop selective estrogen receptor modulators (SERMs) for HRT (McDonnell, 2000), which can be taken for many years without eliciting serious side effects. Estrogens and SERMs produce their effects by binding to two estrogen receptors, ERα and ERβ (Green et al., 1986; Kuiper and Gustafsson, 1997). These drugs are classified based on their effects on target tissues. An estrogen acts as an agonist in all tissues, even though it can produce opposite effects. For example, estrogens promote breast cancer but prevent colon cancer (Writing Group for Women's Health Initiative, 2002). SERMs, such as tamoxifen and raloxifene, exhibit both estrogenic and antiestrogenic properties, depending on the tissue type. The antiestrogenic action of tamoxifen on breast cells has been exploited for decades to prevent recurrences of ER-positive breast tumors (Fisher et al., 1996). Tamoxifen is also effective at preventing breast cancer in high-risk women (Fisher et al., 1998), and it elicits beneficial estrogenic activity in the bone to prevent osteoporosis (Love et al., 1992). In contrast, the estrogenic activity of tamoxifen in the uterus can lead to endometrial cancer (Bernstein et al., 1999). Like tamoxifen, raloxifene prevents osteoporosis by acting as an agonist in bone (Delmas et al., 1997; Ettinger et al., 1999) and prevents breast cancer by acting as an antagonist (Cummings et al., 1999). However, raloxifene is not associated with an increased risk of endometrial cancer (Baker et al., 1998). Unlike estrogens, these SERMs do not relieve hot flushes (Cohen and Lu, 2000).
These clinical observations clearly illustrate that SERMs exert common and distinct tissue-specific effects compared with estrogens and that even different SERMs exhibit tissue selectivity. Elucidating the mechanism whereby estrogens and SERMs produce tissue-specific effects is important for designing better drugs to treat conditions associated with estrogen deficiency, such as menopausal symptoms and osteoporosis or excessive estrogen action, such as breast cancer. New paradigms have recently emerged regarding the molecular mechanism of action of estrogens and SERMs based on the discovery of coregulatory proteins (McKenna et al., 1999; McDonnell and Norris, 2002) that interact with ERs and structural studies of the ER ligand binding domain (LBD) (Brzozowski et al., 1997; Shiau et al., 1998; Pike et al., 1999). Estrogen initiates transcriptional activation by inducing a conformational change of the ER LBD (Brzozowski et al., 1997; Shiau et al., 1998). The repositioning of helix 12 by estrogens creates an activation function (AF)-2 surface that permits the binding of coactivators (Feng et al., 1998), which facilitate the recruitment of factors that activate transcription or cause the remodeling of chromatin structure. In contrast, when SERMs bind to ERα the LXXML sequence in helix 12 interacts with the AF-2 surface and occludes the coactivator LXXLL recognition site (Shiau et al., 1998). Thus, unlike estrogens, SERMs do not form a functional AF-2 surface (Brzozowski et al., 1997; Shiau et al., 1998), which prevents the binding of coactivators required for gene activation. The important role of coregulatory proteins in producing tissue-specific effects was demonstrated by the findings that tamoxifen recruits the corepressor N-CoR in breast cells (Shang et al., 2000), where it acts as an antagonist, but recruits the coactivator SRC-1 in endometrial cells, where it acts as an agonist (Shang and Brown, 2002). These observations demonstrate that a major mechanism whereby estrogens and SERMs produce tissue-specific effects is by recruiting different coregulatory proteins to ERs.
Evidence derived from transient transfection experiments indicates that estrogens and SERMs also produce tissue-specific effects by differentially regulating response elements in target genes with ERα and ERβ. In response to estradiol (E2), ERα is more effective than ERβ at activating a classical estrogen response element (ERE) (An et al., 1999). In contrast, ERβ is more effective at activating an AP-1 element with SERMs compared with ERα (Paech et al., 1997). In fact, E2 is an antagonist of SERM-mediated activation of AP-1 elements (Paech et al., 1997). Compared with simple response elements used in reporter plasmids, it is not known whether E2 and SERMs also exert distinct regulatory effects on native target genes of ERα and ERβ. Identifying target genes regulated by estrogens and SERMs is a critical first step required for subsequent characterization of the types of response elements present in ERα and ERβ target genes and elucidation of the mechanisms whereby ERα and ERβ regulate distinct genes in response to different ligands. In this study, we used microarray technology to compare the effects of E2 and SERMs on global patterns of gene expression in a bone cell line stably transfected with ERα or ERβ. Our study indicates that estrogens and SERMs can produce tissue-specific effects by regulating different targets genes with ERα and ERβ.
MATERIALS AND METHODS
Materials
The U2OS (human osteosarcoma) cells stably transfected with the tet repressor, zeocin, hygromycin, TRIzol Reagent, pcDNA 6/V5-His, NuPAGE gels, SuperScript Choice System Platinum TaqDNA polymerase, and SuperScript II were purchased from Invitrogen (Carlsbad, CA). Human ERα and ERβ cDNAs were obtained from P. Chambon, and J.-A. Gustafsson, respectively. Monoclonal ERα (ID5) antibody was obtained from DAKO (Carpinteria, CA), and monoclonal ERβ antibodies (6A12, 14C8, and 7B10.7) were from GeneTex (San Antonio, TX). The Elite ABC kit was purchased from Vector Laboratories (Burlingame, CA). Enhanced chemiluminescence kits were obtained from Amersham Biosciences (Piscataway, NJ). RNeasy columns were manufactured by QIAGEN (Valencia, CA). The pGEM T-easy kit was obtained from Promega (Madison, WI). Human U95Av2 GeneChips, Test3 Arrays, BioArray High-Yield RNA Transcript Labeling kit, and the Microarray Suite version 5.0 software were obtained from Affymetrix (Santa Clara, CA). Oligonucleotides were synthesized by IDT Technologies (Coralville, IA). All other reagents were purchased from Sigma-Aldrich (St. Louis, MO) or as described previously (An et al., 1999, 2001).
Cell Culture and Preparation of U2OS-ERα and ERβ Stable Cell Lines
The MCF-7 breast cancer cell line was cultured in phenol-free DMEM/F-12 media containing 5% fetal bovine serum, 2 mM glutamine, 50 U/ml penicillin, and 50 μg/ml streptomycin. The U2OS cells were maintained in phenol-free DMEM/F-12 containing 5% stripped fetal bovine serum, 2 mM glutamine, 50 U/ml penicillin, 50 μg/ml streptomycin, 50 μg/ml hygromycin B, and 500 μg/ml zeocin. The U2OS cells stably expressing the tet repressor were transfected with pcDNA 6/V5-His vector containing ERα or ERβ cDNA.
Immunohistochemistry for ERα and ERβ
The U2OS-ERα and ERβ cell lines were plated on chamber slides and treated with 1 μg/ml doxycycline for 18 h to induce ER expression. The slides were fixed in neutral-buffered formalin and incubated in a microwave oven at full power for 20 min in 10 mM citrate buffer, pH 6, for antigen retrieval. After cooling, the slides were treated for 20 min with hydrogen peroxide/methanol to quench endogenous peroxidase activity. The slides were washed in phosphate-buffered saline (PBS) for 5 min, followed by a 30-min incubation at room temperature with 3% horse serum/PBS/0.3% Triton X-100. The slides were incubated overnight at 4°C with either anti-ERα (1:200), two mouse monoclonal ERβs (14C8 and 7B10.7, 1:600 each), or without antibody to serve as a negative control. After washing in buffer, cells were stained with the avidin-biotin-peroxidase method (Elite ABC kit; Vector Laboratories), with diaminobenzidine as the Chromagen, followed by counterstaining with hematoxylin to visualize the nuclei.
Western Blot Analysis
Ten micrograms of total proteins from the U2OS-ERα and ERβ cells were used for Western blot. The membranes were probed with anti-ERα (DAKO antibody, diluted 1:1500 in blocking buffer) or three monoclonal ERβ antibodies (GeneTex) in 1:3000 in blocking buffer overnight at 4°C. Proteins were visualized using the enhanced chemiluminescence detection system.
Estrogen Receptor Binding Assay
U2OS-ERα stable cells grown in six-well dishes were treated for 18 h with 1 μg/ml doxycycline. After the treatment, cells were incubated [37°C, 2 h] with 0.1-20 nM [3H]E2 [specific activity 87.6 Ci/mmol; PerkinElmer Life Science, Boston, MA] in the absence and presence of 100-fold excess of the unlabeled E2). After washing with 0.1% bovine serum albumin in PBS, SDS lysis buffer (0.5% SDS, 0.05 M Tris-HCl, pH 8.0, 1 mM dithiothreitol) was added and cells were shaken overnight. Specific binding of [3H]E2 was calculated as the difference between total and nonspecific binding.
Microarrays and Data Analysis
The expression of ERs in the U2OS-ERα and ERβ cells were induced with doxycycline in the absence or presence of 10 nM E2, 1 μM raloxifene, or 1 μM tamoxifen for 18 h. The U2OS-ERα or ERβ cells were washed with PBS and then 1 ml of TRIzol was added to the cells. Total RNA was prepared according to the manufacturer's protocol. DNase-I treated RNAs were purified further using the RNeasy columns. Total RNA was used to synthesize double-stranded cDNA by using Superscript Choice System incorporating a T7 RNA polymerase promoter. Biotin-labeled antisense cRNA was prepared using the BioArray High-Yield RNA Transcript Labeling kit transcription kit using 6 μg of total RNAs and the oligo-dT primer 5′ GGCCAGTGAATTGTAATACGACTCACTATAGGGAGGCGG-(dT)24. cRNAs were purified with the RNeasy columns and then 20 μg of cRNAs was fragmented at 94°C for 30 min in 40 μl of 40 mM Tris-acetate, pH 8.1, 100 mM KOAc, 30 mM MgOAc. The fragmented samples (n = 4 from untreated, n = 4E2,n = 3 raloxifene, and n = 3 tamoxifen) were hybridized with the Affymetrix Test3 Arrays and the human U95Av2 GeneChips and scanned at the Molecular Biology and Genomics Laboratory at San Francisco General Hospital. The data of untreated versus treated samples were analyzed using the Microarray Suite Version 5.0 with the default parameters. The comparative data generated for each treated group were analyzed further in Microsoft Excel. Genes displaying no signal change relative to controls in at least three experiments were considered insignificant and excluded from further analysis. Genes displaying either increase or decrease signal were selected for further analysis only if they had a ±0.8 or ±1.2 signal log ratio mean value (±1.74- and ± 2.3-fold change, respectively) and were statistically significant (p < 0.05) in at least three separate experiments.
Semiquantitative Reverse Transcription-Polymerase Chain Reaction (RT-PCR) and Real-Time RT-PCR
The U2OS cells were treated for 18 h with E2, raloxifene, or tamoxifen. Reverse transcription was performed in a 10-μl reaction with 1 μg of total RNA, 50 mM Tris-HCl, pH 8, 75 mM KCl, 3 mM MgCl2, 10 mM dithiothreitol, 500 μM each dNTPs, 50 ng of random hexamers, and SuperScript II at 42°C 1 h. The cDNA was diluted 10-fold and then 1 μl of the dilution was used in a 12.5-μl PCR reaction containing 66 mM Tris-HCl, pH 9, 16 mM (NH4)2SO4, 140 μg/ml bovine serum albumin, 0.4 μM each primer, 200 μM each dNTP, 2 mM MgCl2, 4% glycerol, 4% dimethyl sulfoxide, and 1 U of Platinum TaqDNA polymerase. PCR was done for 94°C at 30 s, followed by cycles at 94°C for 10 s, 55-72°C 20 s, and then 72°C 30 s. Twenty-four to 36 PCR cycles were used, depending on the genes amplified. The following primers were used for PCR: α-anti-trypsin (α-AT), 5′ TGCCACCGCCATCTTCTTCC and 5′ ACATGGCCCCAGCAGCTTCAGTCC; WISP-2, 5′ AGCCCTGCGACCAACTCCAC and 5′ GGCCGCACACCCACTCAGG; Mda-7, 5′ TATTGTGCCCCATGCTTCTTTACC and 5′ CCCCACCCCAATGCTCTGTC; NKG2C, 5′ TCCCCGAATACAAGAACGCAGAA and 5′ TTGGGAGAAAGAGGGTAGAATGAT; cDNA clone image 996282, 5′ GCTCTCCTGGGCAGCGTTGTG and 5′ CTCCGAGTTTATTGGGTGTTTGTT; transforming growth factor β3 (TGFβ3), 5′ GGTGGTCCTGGCCCTGCTGAA and 5′ GCTCCCGGGTGCTGTTGTAAAG; G0S2, 5′ GCTCGCGCTGCCTCCTGCTC and 5′ TTGCGCTTCTGGGCCATCATCTC; thrombin receptor, 5′ GATCCCCGGTCATTTCTTCTC and 5′ ACCACCGCCGGCTTCTTGACCTTCA; and NKG2E, 5′ TCCCCGAATACAAGAACGCAGAA and 5′ TTAATTGGGAGAAAGAGGGTAGAA. Glyceraldehyde-3-phosphate dehydrogenase primers 5′ ACCACAGTCCATGCCATCAC and 5′ TCCACCACCCTGTTGCTGTA were used for internal control. PCR products were loaded onto 2% agarose Tris borate-EDTA gels, and visualized by ethidium bromide staining.
Real-time RT-PCR was performed with the iQ SYBR green supermix on the Bio-Rad iCycler Thermal Cycler system. The typical temperature profile was an initial denaturation at 94°C, 3 min, followed by 40 cycles at 94°C for 10 s, 60-64°C for 20 s, and then 72°C for 30 s. The data were collected and analyzed using the comparative threshold cycle method.
Northern Blotting
Twenty micrograms of total RNAs from untreated and 10 nM E2-treated doxycycline-induced U2OS-ERα and ERβ cells were used for Northern blot. Keratin 19 (K19) cDNA (nt 170-311, accession no. Y00503) was amplified by RT-PCR with primers 5′ CGTGTCCTCCGCCCGCTTTGTGTC and 5′ GGAGGCCAGGCGGTCGTTGAGGTT, ligated into the pGEM vector, and verified by DNA sequencing on both strands. The cDNA insert was labeled with [32P]dCTP by random priming, and 2 × 106 cpm/ml probe was hybridized with the blot overnight at 64°C in 0.5 M Na2HPO4, 7% SDS, 1 mM EDTA, and 100 μg/ml salmon sperm DNA. The blot was washed twice in 0.1× SSC/0.1% SDS at 64°C for 20 min and subjected to autoradiography.
Chromatin immunoprecipitation (ChIP)
After an overnight treatment with 10 nM E2, the U2OS-ERα and ERβ cells were fixed in 1% formaldehyde solution and washed with PBS, collected, and lysed on ice in the presence of protease inhibitors. The nuclear pellet was sonicated, and chromatin was collected by centrifugation. Extracts were pre-cleared with protein G-Sepharose. Before adding anti-ERα or anti-ERβ, an aliquot of each sample was removed to use as an input for PCR. Immunoprecipitation was performed on a rocking platform at 4°C overnight, and immunocomplexes were captured by protein G-Sepharose beads and washed several times. Isolated chromatin was phenol-extracted and precipitated with ethanol. PCR was done with K19 primers 5′ TCCAGCCTGGGTGACAGAGC and 5′ TCCAAGTTCACCCCAACCTGA, which span the consensus ERE and half ERE in the K19 enhancer region (Choi et al., 2000).
RESULTS
The Inducible-U2OS-ERα and U2OS-ERβ Cell Lines Synthesize Exclusively ERα and ERβ, Respectively
U2OS cells were stably transfected with a tetracycline-inducible vector to express ERα or ERβ. In the absence of doxycycline, the Tet repressor is bound to the Tet response elements in the cytomegalovirus promoter, preventing the transcription of the ER cDNA. Doxycycline binds to the Tet repressor, causing it to be released from the promoter, thereby allowing the cytomegalovirus promoter to drive the expression of ERα and ERβ.
The inducible expression of ER in the U2OS cell lines was characterized by performing Western blots, immunoprecipitation, immunohistochemistry, and receptor binding assays. The addition of doxycycline produced a time-dependent accumulation of ERα or ERβ protein (Figure 1A). There seemed to be very little, if any, “leaky” expression of ERα and ERβ in the absence of doxycycline. Furthermore, no ERβ (U2OS-ERβ, lane 7) was detected in ERα cells, nor was ERα detected in ERβ cells (U2OS-ERα, lane 14). Immunohistochemistry (Figure 1B) and immunoprecipitation (Figure 1C) studies confirmed that U2OSERα cells synthesized only ERα and that U2OS-ERβ cells expressed exclusively ERβ. After an 18-h treatment with doxycycline, the U2OS-ERα and U2OS-ERβ cell lines contained 69,000 and 54,000 receptors per cell by [3H]E2 binding studies (our unpublished data), respectively. Our results demonstrate that these cell lines can be used to identify target genes that are regulated exclusively by ERα or ERβ in response to E2 and SERMs.

Characterization of the stable U2OSERα and U2OS-ERβ cell lines. (A) Doxycycline produces a time-dependent increase in ERα and ERβ. The U2OS-ERα (left) and U2OS-ERβ (right) cell lines were treated with 1 μg/ml doxycycline for increasing times before performing Western blots. Lanes 7 and 14 show that there is no ERβ detectable in the U2OS-ERα cells and no ERα detectable in the U2OS-ERβ cells, respectively. (B) Immunohistochemistry of U2OS-ERα and U2OS-ERβ cells. Cells were treated with 1 μg/ml doxycycline for 18 h on slides, fixed with formalin, and stained for ERα and ERβ as described in MATERIALS AND METHODS. Cells labeled 1 and 5 were not induced with doxycycline. Cells labeled 2 and 6 were induced with doxycycline but did not receive primary antibody. Cells labeled 3 and 7 were induced with doxycycline and stained with anti-ERβ and anti-ERα, respectively. Cells labeled 4 and 8 were induced with doxycycline and stained with anti-ERα and anti-ERβ, respectively. (C) Immunoprecipitation of ERα and ERβ in the stable cell lines. Cells were treated with 1 μg/ml doxycycline for 18 h and the immunoprecipitated with anti-ERα (lanes 3, 4, and 7) and anti-ERβ (lanes 2, 8, and 9). Lanes 1 and 6 show a positive control from cell lysate of U2OS-ERα and U2OS-ERβ cells, respectively. All three techniques demonstrate that ERα is detected only in the ERα cells, whereas ERβ is detected exclusively in the ERβ cells.
Genes Regulated by ERα Are Distinct from Those Regulated by ERβ in Response to E2 and SERMs
To identify genes regulated by ERα and/or ERβ, the U2OSERα and U2OS-ERβ cell lines were treated with doxycycline for 18 h to induce ER expression in the absence or presence of 10 nM E2, 1 μM raloxifene, or 1 μM tamoxifen. Total RNA was used to prepare cRNA for hybridization with human U95Av2 microarrays (Affymetrix), which contain 12,600 known genes. Six sets of comparative expression data of untreated versus each treated group were used to determine the genes regulated in ERα and ERβ cells. In both U2OS-ERα and U2OS-ERβ cells, a total of 228, 190, and 236 genes were activated or repressed by E2, raloxifene, and tamoxifen, respectively (Table 1). Table 2 shows a partial list of the statistically significant (p < 0.05) regulated genes that had a mean ± 0.8 signal log ratio value (±1.74-fold change). E2 activated 67 genes in the U2OS-ERα cells and 121 in the U2OS-ERβ cells (Table 1). Only 34 genes were activated by E2 in both cell lines. E2 repressed 36 genes in U2OS-ERα cells and 42 genes in U2OS-ERβ cells, whereas only four genes were repressed by E2 in both cell lines. These findings demonstrate that only 38 of the 228 (17%) genes are regulated by both ERα and ERβ with E2.
Table 1.
Differential gene regulation by E2 and SERMs in the U2OS-ERα and U2OS-ERβ cell lines

(A) Doxycycline-induced U2OS-ERα and U2OS-ERβ cells were treated with 10 nM E2, 1 μM raloxifene, or 1 μM tamoxifen for 18 h. Microarray data obtained from human Affymetrix U95Av2 gene chips from untreated versus ligand-treated samples were analyzed using the Affymetrix Microarray Suite Version 5.0. Candidate genes displaying a statistically significant (p < 0.05) increase or decrease signal changes relative to controls in at least three experiments were further selected by a ±0.8 signal log ratio mean cut-off (±1.74-fold). The numbers of genes activated or repressed in ERα, ERβ, and both ERα + ERβ cell lines are shown. Asterisks (*) indicate the number of common genes regulated by SERMs in the ERα cells that displayed opposite expression patterns compared with ERβ cells. Real-time RT-PCR for α-anti-trypsin, K19, WISP-2, Mda-7, NKG2C, and NKG2E was performed on U2OS-ERα and U2OS-ERβ samples treated for 18 h with 10 nM E2, 1 μM raloxifene, or 1 μM tamoxifen. Fold-changes in the U2OS-ERα and U2OS-ERβ samples were calculated relative to the untreated samples.
Table 2.
Subset of the regulated genes in each treatment identified by the microarrays
Gene | Function | Mean signal log ratio ± S.E. | Accession number | |
---|---|---|---|---|
ERα, E2 | Keratin 19 | Cell structure | 5.45 ± 0.97 | Y00503 |
Transglutaminase | Protein modification | 2.93 ± 0.15 | M55153 | |
Angiotensinogen | Blood pressure regulation | 2.45 ± 0.50 | K02215 | |
WISP-2 | Signal transduction | 2.43 ± 0.39 | AF100780 | |
α1 antitrypsin | Serine proteinase inhibitor | 1.63 ± 0.18 | X01683 | |
G protein-coupled receptor | Signal transduction | 1.33 ± 0.28 | D38449 | |
Progression associated protein | Cell proliferation | −1.43 ± 0.47 | Y07909 | |
Hyaluronan synthase 2 | Cell proliferation | −2.08 ± 0.77 | U54804 | |
ERβ, E2 | Mda-7 | Tumor suppressor | 4.68 ± 0.78 | U16261 |
Keratin 19 | Cell structure | 3.55 ± 0.38 | Y00503 | |
Putative cyclin G1 interacting protein | Unknown | 2.03 ± 0.42 | U61836 | |
Metalloproteinase | Proteolysis and peptidolysis | 1.40 ± 0.30 | L23808 | |
TRAF-interacting protein 1-TRAF | Signal transduction | 1.28 ± 0.17 | U59863 | |
Prepro-relaxin H2 | Pregnancy | 1.08 ± 0.10 | X00948 | |
WISP-2 | Signal transduction | 0.83 ± 0.15 | AF100780 | |
Fibroblast growth factor receptor (K-sam) | Oncogenesis | −1.1 ± 0.29 | M87770 | |
ERα, raloxifene | NKG2C | Cellular defense response | 2.40 ± 0.82 | AJ001684 |
Zinc finger transcriptional regulator | mRNA catabolism | 1.70 ± 0.20 | M92843 | |
Mitochondrial isocitrate dehydrogenase | Carbohydrate metabolism | 1.33 ± 0.50 | X69433 | |
Transforming growth factor β3 | Cell-cell signalling | 1.23 ± 0.43 | X14885 | |
Phosphatidic acid phosphohydrolase homolog | Lipid metabolism | 0.97 ± 0.34 | AF017786 | |
Tumor-associated membrane protein homolog | Oncogenesis | −1.00 ± 0.13 | U43916 | |
Microfibril-associated glycoprotein 2 | Extracellular matrix | −1.47 ± 0.65 | U37283 | |
MHC class III HSP70-2 | Heat shock response | −1.57 ± 0.21 | M59830 | |
ERβ, raloxifene | cDNA DKFZp586A0522 | Unknown | 2.10 ± 0.35 | AL050159 |
γ-aminobutyric acid receptor type A ρ1 subunit | Signal transduction | 1.63 ± 0.45 | M62400 | |
β-filamin | Cytoskeletal anchoring | −1.47 ± 0.10 | AF042166 | |
Radiation-inducible immediate-early gene | Cell growth/maintenance | −1.50 ± 0.07 | S81914 | |
Neutrophil oxidase factor | Cellular defense response | −2.50 ± 0.13 | M32011 | |
Endothelin 3 | Signal transduction | −3.23 ± 1.02 | X52001 | |
NKG2C | Cellular defense response | −5.20 ± 0.08 | AJ001684 | |
cDNA clone image 996282 | Unknown | −5.27 ± 1.63 | AA532495 | |
ERα, tamoxifen | G0S2 | G0/G1 switch | 2.97 ± 0.60 | M69199 |
NKG2E | Cellular defense response | 2.23 ± 0.62 | AJ001685 | |
Forkhead protein | Anti-apoptosis | 1.03 ± 0.32 | AF032885 | |
Flotillin-1 | Caveolae formation | 1.00 ± 0.12 | AF089750 | |
Thrombin receptor | Blood clotting | −1.40 ± 0.35 | M62424 | |
cDNA DKFZp586G2222 | Unknown | −1.83 ± 0.50 | AL080111 | |
cDNA clone image 302798 | Adenylate cyclase activation | −1.90 ± 0.44 | N90755 | |
cNA clone image 2368811 | Signal transduction | −2.30 ± 0.74 | AI743745 | |
ERβ, tamoxifen | Nicotinamide N-methyltransferase | Xenobiotic metabolism | 1.60 ± 0.44 | U08021 |
Involucrin | Keratinocyte differentiation | −1.67 ± 0.33 | M13903 | |
v-maf musculoaponeurotic fibrosarcoma (avian) oncogene family, protein F | DNA-binding protein | −1.97 ± 0.25 | AL021977 | |
Tumor-associated 120 kDa nuclear protein | RNA processing | −2.43 ± 1.17 | D13413 | |
Protein tyrosine phosphatase precursor | Protein dephosphorylation | −2.57 ± 0.50 | D15049 | |
Factor XIII subunit A | Blood coagulation | −3.40 ± 0.87 | M14539 | |
NKG2E | Cellular defense response | −5.20 ± 0.73 | AJ001685 |
Cells were treated and the microarray analysis was done as described in Table 1: A complete list of genes regulated by E2, raloxifene, and tamoxifen are presented as supplementary material.
Raloxifene and tamoxifen activated and repressed a number of genes in the U2OS-ERα and U2OS-ERβ cell lines. Similar to E2, the genes regulated by raloxifene or tamoxifen in U2OS-ERα cells were distinct from those regulated in U2OS-ERβ cells. However, two distinguishing features occurred with SERMs compared with E2. First, many more genes were activated or repressed by SERMs in U2OS-ERβ cells compared with the ERα cells. For example, 52 and 26 genes were induced by raloxifene and tamoxifen, respectively, in ERβ cells, but only 10 and 21 genes were induced in ERα cells (Table 1). Although 101 and 129 genes were repressed by SERMs in ERβ cells, only 10 and 38 genes were inhibited in ERα cells. Second, the majority of genes regulated by SERMs in both ERα and ERβ cells displayed opposing expression patterns. Raloxifene regulated 17 genes in opposite directions, whereas tamoxifen regulated 12 genes in opposite directions. For example, raloxifene activated NGK2C in the U2OS-ERα cells and inhibited NGK2C in the U2OS-ERβ cells (Table 1).
The regulation of α-AT, K19, WISP-2, Mda-7, NKG2C, and NKG2E by E2, raloxifene, or tamoxifen in the U2OS-ERα and ERβ cell lines was verified by real-time PCR (Table 1). Furthermore, the regulation of some genes by E2 (WISP-2 and α-AT), raloxifene (NKG2C and 996282), and tamoxifen (NKG2E and G0S2) was dose dependent (Figure 2). Overall, only 17-18% of the genes regulated by E2 were also regulated by raloxifene or tamoxifen, and 37% of the genes regulated by raloxifene were also regulated by tamoxifen in both U2OS-ERα and ERβ cell lines (Table 3A). The name of all genes regulated by E2, tamoxifen, and raloxifene are presented as a supplementary material. We also found little overlap of genes regulated by ERα and ERβ when the cutoff for regulation by E2 and SERMs was increased to 2.3-fold (Table 3B). These results clearly demonstrate that the majority of genes regulated by ERα are different from those regulated by ERβ in response to E2 or SERMs.
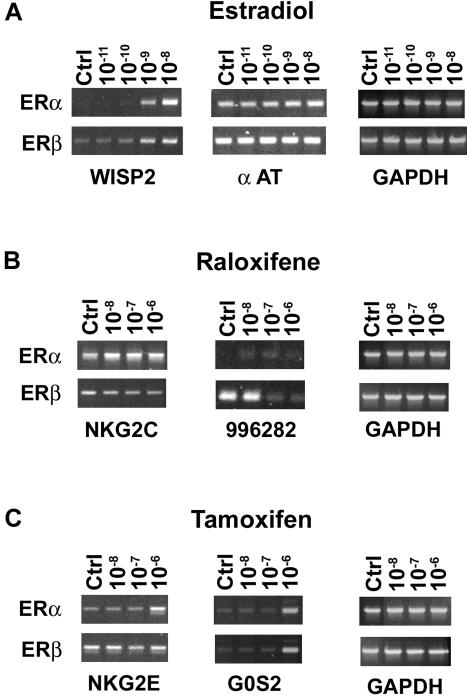
Regulation of selected genes by E2 and SERMs in the U2OS-ERα and ERβ cell lines. Doxycycline-induced U2OS-ERα and ERβ cells were treated for 18 h with 10-11-10-8 M E2 (A), 10-8-10-6 M raloxifene (B), or 10-8-10-6 M tamoxifen (C). The extracted total RNA was analyzed by RT-PCR as described in MATERIALS AND METHODS. The genes examined were the WISP-2, α-AT, NKG2C, cDNA clone image 996282, NKG2E, and G0S2. Glyceraldehyde-3-phosphate dehydrogenase was used as an internal control. The data presented were representative of at least three experiments.
Table 3.
Summary of genes commonly regulated by E2 and SERMs in the U2OS-ERα and U2OS-ERβ cell lines
A | |||
---|---|---|---|
Treatments | Total number of genes | Number of common genes | Percentage of common genes |
E2 vs. raloxifene | 228 vs. 190 | 65 | 18% |
E2 vs. tamoxifen | 228 vs. 236 | 68 | 17% |
Raloxifene vs. tamoxifen | 190 vs. 236 | 116 | 37% |
B | |||
---|---|---|---|
Treatments | Total number of genes | Number of common genes | Percentage of common genes |
E2 vs. raloxifene | 105 vs. 103 | 34 | 20% |
E2 vs. tamoxifen | 105 vs. 115 | 35 | 28% |
Raloxifene vs. tamoxifen | 103 vs. 115 | 62 | 40% |
(A) Using a ±1.74 fold-change cutoff in the microarray analysis, 228, 190, and 236 genes were regulated by E2, raloxifene, and tamoxifen, respectively, in U2OS-ERα and U2OS-ERβ cell lines. Among these genes, 65, 68, and 116 were commonly regulated by E2 and raloxifene, E2 and tamoxifen, and raloxifene and tamoxifen, respectively. (B) Using a ±2.3 fold-change cutoff, 105, 103, and 115 genes were regulated by E2, raloxifene, and tamoxifen, respectively. Thirty-four, 35, and 62 were commonly regulated by E2 and raloxifene, E2 and tamoxifen, and raloxifene and tamoxifen, respectively.
Bone-Related Genes Regulated by ERα Are Distinct from Those Regulated by ERβ in Response to E2 and SERMs
Because bone cells were used for these studies, we decided to further analyze the subset of regulated genes known to be involved in bone homeostasis or metabolism. We identified 30 genes that were differentially regulated in the ERα and ERβ cells treated with E2 or SERMs (Table 4). E2 activated four bone-related genes in the ERα cells. Only one of these genes was also induced by raloxifene, whereas another gene was activated by tamoxifen. Two genes were activated only by E2, and one gene was specifically activated by raloxifene. In the ERβ cells, a total of 16 genes were activated by all three drugs, but only one was induced by both raloxifene and tamoxifen, whereas the remaining eight, six, and one genes were uniquely activated by E2, raloxifene, and tamoxifen, respectively.
Table 4.
ERα- and ERβ-regulated genes involved in bone homeostasis and metabolism

Thirty different candidate genes with known bone-related functions identified by the microarrays are categorized by treatments with E2, raloxifene, or tamoxifen in U2OS-ERα and ERβ cells. Venn diagrams show the number of bone-related genes activated and repressed by E2, raloxifene, and tamoxifen. The unique genes regulated only by ERα were multiple exostoses type II protein EXT2.1, osteoclastogenesis inhibitory factor, insulin-like growth factor binding protein 5, transforming growth factor β3, latent transforming growth factor-β binding protein 2, and OB-cadherin 2. Hindlimb expressed homeobox protein backfoot, ATP sulfurylase/APS kinase 2, cyclooxygenase-2, bone morphogenetic protein 4, Mad protein homolog, transforming growth factor β-induced gene product (BIGH3), platelet-derived growth factor receptor α, TGF-β type II receptor α, lumican, transforming growth factor β1 binding protein, α-1 type XI collagen, metalloprotease/disintegrin/cysteine-rich protein precursor, and cellular fibronectin were uniquely regulated in ERβ.
Seven bone genes were repressed in the ERα cells in response to E2 and SERMs, but only one was inhibited by both E2 and tamoxifen, whereas two, one, and three genes were inhibited specifically by E2, raloxifene, and tamoxifen, respectively. In the ERβ cells, the three drugs repressed 11 genes, and three of these genes were commonly regulated by both SERMs. Three, two, and three genes were inhibited specifically by E2, raloxifene, and tamoxifen, respectively. Overall, 6 and 13 unique genes were regulated in the ERα and ERβ cells, respectively, when treated with E2 and SERMs. Thus, the U2OS-ERα and ERβ cell lines displayed differential expression patterns of bone-related genes in response to E2, tamoxifen, and raloxifene.
The Effect of ERα Protein Level on Gene Expression Patterns in the U2OS-ERα Cell Line
To evaluate whether gene regulation patterns also occur at lower ER levels, we treated the U2OS-ERα cells for 18 h with 10 nM E2 and increasing amounts of doxycycline. Immunoblotting shows that the level of ERα expression increased with the dose of doxycycline (Figure 3A). As determined by semiquantitative RT-PCR, no induction of WISP-2 mRNA was observed in cells not treated with doxycycline. In contrast, even at the lowest dose of doxycycline (0.1 μg/ml), we detected an E2-dependent induction of WISP-2 in the U2OSERα cells (Figure 3B, lane 7).
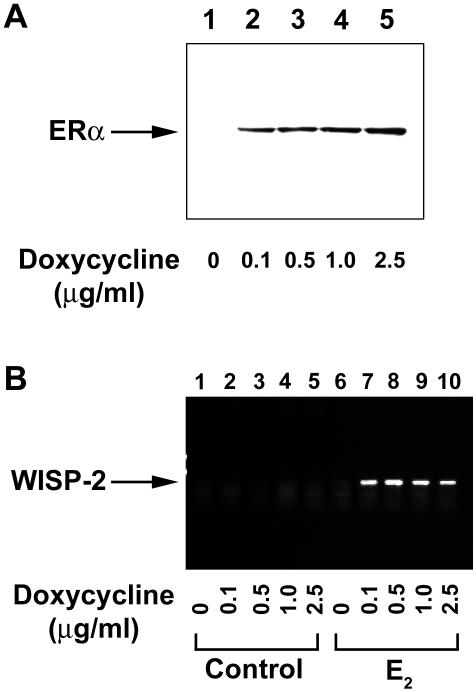
Effect of ERα protein level on WISP-2 expression in the U2OS-ERα cell line. The U2OS-ERα cells were treated for 18 h with 10 nM E2 and increasing concentrations of doxycycline (0.1-2.5 μg/ml). (A) The level of ERα expression was determined by Western blot analysis with anti-ERα antibodies. (B) The expression of WISP-2 was evaluated by semiquantitative RT-PCR in control (lanes 1-5) and E2-treated (lanes 6-10) U2OS-ERα cell line.
We also examined the expression patterns of raloxifene- and tamoxifen-specific genes, after only a 3-h exposure to doxycycline, when the expression of ERα was comparatively low (Figure 1A, lane 3). We found that raloxifene activated TGFβ3 (Figure 4A, lane 3), and tamoxifen activated G0S2 (lane 10) and repressed thrombin receptor (lane 15) at 3-h drug treatment by semiquantitative RT-PCR. Whereas some of the E2 and SERM targets identified by the microarrays could be secondary, regulated by gene products induced earlier by liganded ERs, the findings that these genes are also regulated by 3 h suggest that some of the genes represent direct ER targets. Consistent with the microarray data, similar results were obtained with TGFβ3, G0S2 and thrombin receptor by real-time RT-PCR after 18-h exposure to raloxifene or tamoxifen (Figure 4B).
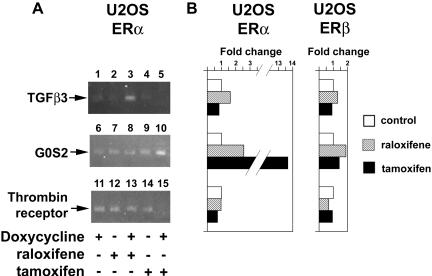
Regulation of SERM-specific genes in the U2OS-ERα cell line. (A) U2OSERα cells were treated with 1 μg/ml doxycycline and 1 μM raloxifene or 1 μM tamoxifen for 3 h. The expression patterns of TGFβ3 (induced by raloxifene), G0S2 (induced by tamoxifen), and thrombin receptor (inhibited by tamoxifen) were evaluated by semiquantitative RT-PCR. (B) U2OS-ERα and ERB cells were treated for 18 h with doxycycline and SERMs, and the expression patterns of TGFβ3, G0S2, and thrombin receptor were measured by real-time qualitative RT-PCR.
E2 Increases K19 mRNA Expression and Recruits ERα and ERβ to the K19 Gene
To establish that ERs interact directly with a regulated gene identified in the inducible cell lines, we examined the effects of E2 on transcriptional regulation of the keratin 19 gene. This gene was chosen because the identification of a near-consensus ERE and half ERE (Choi et al., 2000) permitted us to design PCR primers spanning this region for the ChIP assays. As shown by Northern blot analysis in Figure 5A, the expression of K19 was induced in U2OS-ERα and U2OS-ERβ cells treated with 10 nM E2. To determine whether ERα and ERβ are recruited to the endogenous K19 ERE enhancer, we performed ChIP assays with E2-treated U2OS-ERα and U2OS-ERβ cells. As shown in Figure 5B, E2 recruited ERα and ERβ to the region of the endogenous K19 gene that contains the ERE enhancer. These results demonstrate that ERs can be detected at the native ERE of an estrogen-inducible gene known to be a physiological ER target.
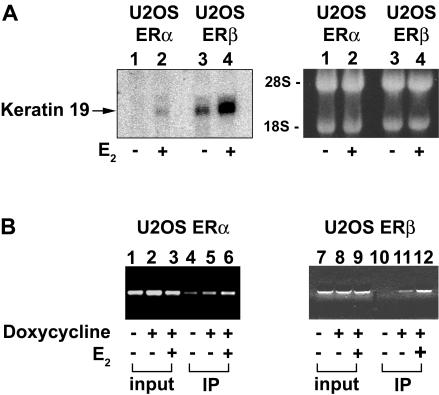
Regulation of keratin 19 in the U2OS-ERα and U2OSERβ cell lines. (A) E2 increases K19 mRNA levels in U2OS-ERα and U2OS-ERβ cells. Northern blot was performed with 20 μg of total RNAs from doxycycline-induced U2OS-ERα or U2OS-ERβ cells incubated in the absence (-) or presence of 10 nM E2 (+) overnight. Before transfer to a nylon blot and hybridization with the K19 cDNA probe (left), the gel containing ethidium bromide-stained RNAs (right) was photographed for a loading control. (B) ERα and ERβ bind the ERE enhancer in the endogenous K19 gene. ChIP assays were performed using U2OS-ERα (left) and U2OS-ERβ (right) cells. Anti-ERα- and anti-ERβ-precipitated DNAs were amplified with PCR primers spanning the near consensus ERE and half ERE in the K19 enhancer region (Choi et al., 2000). PCR products from input chromatin before and after immunoprecipitation (IP) are shown.
E2 and SERMs Increase the Expression of the Same Genes in MCF-7 Breast Cancer Cell Line
To investigate whether genes identified by microarrays are also regulated in cells not transfected with ERs, we examined the effect of the three drugs on several genes in MCF-7 breast cancer cells, which express endogenous ERα protein. Similar to the U2OS-ERα cells, E2 also increased the expression of K19, WISP-2, and α-AT in MCF-7 cells (Figure 6A). Raloxifene also increased the expression of NKG2C and clone 996282, whereas tamoxifen increased NKG2E and G0S2 in MCF-7 cells (Figure 6, B and C). Thus, several target genes identified by microarrays in U2OS-ERα cells are regulated similarly in MCF-7 cells that express endogenous ERα.
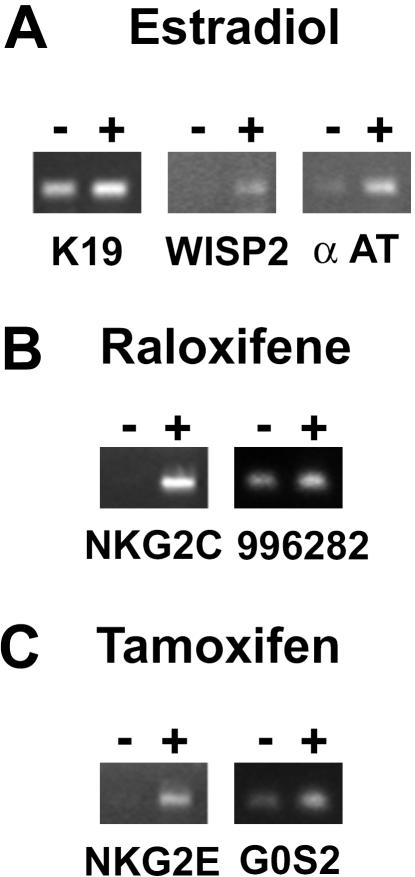
Regulation of selected genes by E2 and SERMs in the breast cancer MCF-7 cell line. ERα expressing MCF-7 cells were treated for 18 h with 10 nM E2 (A), 1 μM raloxifene (B), or 1 μM tamoxifen (C). The expression patterns of K19, WISP-2, α-AT, NKG2C, unknown cDNA 996282, NKG2E, and G0S2 were determined by semiquantitative RT-PCR.
DISCUSSION
We used microarray technology to identify genes regulated by estrogens and SERMs. Our results demonstrated that most genes regulated by E2 and SERMs in ERα cells were distinct from those genes regulated in ERβ cells. Because these results were obtained with a single time point and one concentration of each drug, it is possible that other patterns of gene regulation may occur with other treatment regimens. In both U2OS-ERα and U2OS-ERβ cell lines, we found that E2, raloxifene, and tamoxifen activated and repressed a total of 228, 190, and 236 genes, respectively, of the 12,600 genes on the GeneChip. Among the genes activated by E2 were keratin 19 and WISP-2, which are known estrogen-inducible genes (Choi et al., 2000; Inadera et al., 2000). Raloxifene increased TGFβ3, which is a known gene regulated by raloxifene in bone (Yang et al., 1996). Most genes regulated by E2 and SERMs in U2OS cells are novel ER targets. Importantly, the regulation of several target genes persisted even when the levels of ERs were lowered by reducing the concentration of doxycycline or by shortening the time of exposure to doxycycline to 3 h. These observations suggest that our microarray results are not due to overexpressed ERs or nonspecific squelching of transcription factors. We also showed that ERα and ERβ are recruited to the ERE enhancer in the keratin 19 gene by ChIP assays. Thus, ERs interacted with a known target gene for estrogens in the stable cell lines. In addition, some regulated genes identified in the U2OS ERα cells were also regulated in MCF-7 cells that express endogenous ERα. Collectively, these observations indicate that the regulated genes in U2OS cells identified by the arrays are authentic target genes.
The complex pattern of gene regulation by E2 and SERMs is surprising. Only 38 of 288 (17%) genes were commonly regulated by E2 in U2OS-ERα and ERβ cells. In comparison, Richer et al. (2002) demonstrated that 25 of 94 (27%) genes were commonly regulated by progesterone in cell lines stably transfected with progesterone receptor A or B. Furthermore, most genes regulated by SERMs differed from each other and from those genes regulated by E2. Only 27% of the genes regulated by raloxifene were also regulated by tamoxifen. Although raloxifene and tamoxifen are classified as SERMs, our results demonstrate that their pathways of actions diverge at the level of gene expression. The finding that tamoxifen and raloxifene regulate different sets of genes could explain why only tamoxifen increases endometrial cancer. Differences in gene expression in response to SERMs were also observed in the ER-negative breast cancer cell line (MDA-MB-231) stably transfected with ERα (Levenson et al., 2002). Our most striking observation was that SERMs regulated some genes in opposite directions with ERα and ERβ. For example, NKG2C was increased by raloxifene in ERα cells, but repressed by raloxifene in ERβ cells. The mechanism and functional significance whereby SERMs regulate some genes in opposite directions requires a better characterization of the promoter elements in those genes. It is unlikely that the differences in gene profiles resulted from different levels of the ERα and ERβ, because receptor binding assays demonstrated that the ERα and ERβ cell lines contained comparable numbers of receptors.
Our observation that ERα and ERβ regulate different genes in response to E2 and SERMs underscores the complexity of steroid receptor-mediated gene transcription. The complexity likely arises from presence of different types of response elements in target promoters and the differential utilization of cofactors and their regulatory surfaces by ERα or ERβ. Three classes of response elements have been described in gene promoters: simple, composite, and tethering. Steroid receptors bind directly and independently to simple elements such as the classic ERE, whereas they bind to DNA in conjunction with other transcription factors at composite elements. Tethering elements include AP-1, Sp1, and nuclear factor-κB (Kushner et al., 2000; Abdelrahim, 2002; Tzagarakis-Foster et al., 2002), which recruit ERs to promoters indirectly through protein-protein interactions. Multiple coregulators interact with ERs to mediate transcriptional regulation, including the p160 proteins (SRC1, GRIP1, and AIB1) (Onate et al., 1995; Hong et al., 1996; Anzick et al., 1997), CBP/p300 (Kamei et al., 1996), and TRAP/DRIP (Fondell et al., 1996; Rachez and Freedman, 2001) complexes. Using a factor pair analysis approach, Rogatsky et al. (2002) showed not only distinct coregulatory proteins but also different active surfaces of the same factors are being selectively engaged by the glucocorticoid receptor in different response elements contexts. The ligand also determines coregulator binding specificity. E2 recruits coactivators, such as GRIP1 (Hong et al., 1996; McKenna et al., 1999), whereas raloxifene and tamoxifen recruit corepressors, such as N-CoR to ERs (Shang et al., 2000; Shang and Brown, 2002). Thus, the interaction of ERα and ERβ with different ligands and response elements, and their recruitment of distinct factors and cofactor surfaces may be largely responsible for the differences in gene expression profiles observed by the microarrays with estrogens and SERMs.
Clinical studies have shown that estrogens or SERMs induce distinct effects in different tissues. For example, estrogens increase the risk of breast cancer, whereas the SERMs prevent ER-positive breast tumors. Identifying the mechanisms whereby estrogens or SERMs produce tissue-specific effects is critical for developing safer drugs for preventing and treating breast cancer and conditions associated with estrogen deficiency. Our results suggest that, in addition to ligand-specific recruitment of coregulators, the relative expression of ERα and ERβ in different cell types may also account for tissue-specific responses to estrogens or SERMs. Our findings indicate that estrogens and SERMs will produce a distinct phenotype in cells that express predominantly ERα compared with those expressing ERβ by regulating different set of genes. Furthermore, any change in the ratio ERα to ERβ in tissues that occurs with aging or disease states may alter the tissue response to estrogens or SERMs. In future studies, it will be of interest to determine whether other patterns of gene regulation occur in response to estrogens and SERMs in cells that express different ratios of ERα to ERβ.
Our microarray analysis has identified multiple ERα and ERβ target genes regulated by E2 and SERMs. These genes provide the groundwork necessary to characterize the different types of response elements that are present in their promoters and to determine the underlying mechanism whereby these genes are differentially regulated by ERα and ERβ. Understanding the mechanisms whereby ERα and ERβ regulate different genes in response to estrogens and SERMs is critical for the development of more tissue-selective and safer drugs for menopausal symptoms and breast cancer.
Acknowledgments
We thank P. Chambon and J.-A. Gustafsson for providing plasmids and Paul Webb for critical reading of the manuscript. This work was supported by a Leukemia and Lymphoma Society Special Fellowship to I. R. and a Bank of America Giannini postdoctoral fellowship to C.T.-F., and by grants to K.R.Y from the National Institutes of Health and National Science Foundation and to D.C.L. from the National Institutes of Health (DK-061966), Paul Beeson Physician Faculty Scholars in Aging Research Program (funded by Alliance for Aging Research, John A. Hartford Foundation, Commonwealth Fund and Starr Foundation), and National Institute of Child Health and Human Development Women's Reproductive Health Research Program.
Notes
Article published online ahead of print. Mol. Biol. Cell 10.1091/mbc.E03-06-0360. Article and publication date are available at www.molbiolcell.org/cgi/doi/10.1091/mbc.E03-06-0360.
Abbreviations used: ChIP, chromatin immunoprecipitation; E2, 17β-estradiol; ER, estrogen receptor(s); ERE, estrogen response element; K19, keratin 19; PBS, phosphate-buffered saline; RT, reverse transcription; SERM, selective estrogen receptor modulator(s); WISP-2, WNT1-inducible signaling pathway protein-2.
Online version of this article contains supplementary material. Online version is available at www.molbiolcell.org.
References
- Abdelrahim, M., Samudio, I., Smith, R., 3rd, Burghardt, R., and Safe, S. (2002). Small inhibitory RNA duplexes for Sp1 mRNA block basal and estrogen-induced gene expression and cell cycle progression in MCF-7 breast cancer cells. J. Biol. Chem. 277, 28815-28822. [Abstract] [Google Scholar]
- An, J., Ribeiro, R.C., Webb, P., Gustafsson, J.A., Kushner, P.J., Baxter, J.D., and Leitman, D.C. (1999). Estradiol repression of tumor necrosis factor-alpha transcription requires estrogen receptor activation function-2 and is enhanced by coactivators. Proc. Natl. Acad. Sci. USA 96, 15161-15166. [Europe PMC free article] [Abstract] [Google Scholar]
- An, J., Tzagarakis-Foster, C., Scharschmidt, T.C., Lomri, N., and Leitman, D.C. (2001). Estrogen receptor beta-selective transcriptional activity and recruitment of coregulators by phytoestrogens. J. Biol. Chem. 276, 17808-17814. [Abstract] [Google Scholar]
- Anzick, S.L., Kononen, J., Walker, R.L., Azorsa, D.O., Tanner, M.M., Guan, X.Y., Sauter, G., Kallioniemi, O.P., Trent, J.M., and Meltzer, P.S. (1997). AIB1, a steroid receptor coactivator amplified in breast and ovarian cancer. Science 277, 965-968. [Abstract] [Google Scholar]
- Baker, V., Draper, M., Paul, S., Allerheiligen, S., Glant, M., Shirfren, J., and Jaffe, R. (1998). Reproductive endocrine and endometrial effects of raloxifene hydrochloride, a selective estrogen receptor modulator, in women with regular menstrual cycles. J. Clin. Endocrinol. Metab. 83, 6-13. [Abstract] [Google Scholar]
- Bernstein, L., Deapen, D., Cerhan, J., Schwartz, S., Liff, J., McGann-Maloney, E., Perkman, J., and Ford, L. (1999). Tamoxifen therapy for breast cancer and endometrial cancer risk. J. Natl. Cancer Inst. 91, 1654-1662. [Abstract] [Google Scholar]
- Brzozowski, A.M., Pike, A.C., Dauter, Z., Hubbard, R.E., Bonn, T., Engstrom, O., Ohman, L., Greene, G.L., Gustafsson, J.A., and Carlquist, M. (1997). Molecular basis of agonism and antagonism in the oestrogen receptor. Nature 389, 753-758. [Abstract] [Google Scholar]
- Choi, I., Gudas, L.J., and Katzenellenbogen, B.S. (2000). Regulation of keratin 19 gene expression by estrogen in human breast cancer cells and identification of the estrogen responsive gene region. Mol. Cell Endocrinol. 164, 225-237. [Abstract] [Google Scholar]
- Cohen, F.J., and Lu, Y. (2000). Characterization of hot flashes reported by healthy postmenopausal women receiving raloxifene or placebo during osteoporosis prevention trials. Maturitas 34, 65-73. [Abstract] [Google Scholar]
- Cummings, S., et al. (1999). The effect of raloxifene on risk of breast cancer in postmenopausal women. J. Am. Med. Assoc. 281, 2189-2197. [Abstract] [Google Scholar]
- Delmas, P., Bjarnason, N., Mitlak, B., Ravoux, A.-C., Shah, A., Huster, W., Draper, M., and Christiansen, C. (1997). Effects of raloxifene on bone mineral density, serum cholesterol concentrations, and uterine endometrium in post-menopausal women. N. Engl. J. Med. 337, 1641-1647. [Abstract] [Google Scholar]
- Ettinger, B., Black, D., Mitlak, B., Knickerbocker, R., Nickelsen, T., Genant, H., Christiansen, C., and Delmas, P. (1999). Reduction of vertebral fracture risk in postmenopausal women with osteoprosis treated with raloxifene. Results from a 3-year randomized clinical trial. J. Am. Med. Assoc. 282, 637-644. [Abstract] [Google Scholar]
- Feng, W., Ribeiro, R.C., Wagner, R.L., Nguyen, H., Apriletti, J.W., Fletterick, R.J., Baxter, J.D., Kushner, P.J., and West, B.L. (1998). Hormone-dependent coactivator binding to a hydrophobic cleft on nuclear receptors. Science 280, 1747-1749. [Abstract] [Google Scholar]
- Fisher, B., et al. (1998). Tamoxifen for the prevention of breast cancer: report of the National Surgical Adjuvant Breast and Bowel Project P-1 study. J. Natl. Cancer. Inst. 90, 1371-1388. [Abstract] [Google Scholar]
- Fisher, B., et al. (1996). Five versus more than five years of tamoxifen therapy for breast cancer patients with negative lymph nodes and estrogen receptor-positive tumors. J. Natl. Cancer Inst. 88, 1529-1542. [Abstract] [Google Scholar]
- Fondell, J.D., Ge, H., and Roeder, R.G. (1996). Ligand induction of a transcriptionally active thyroid hormone receptor coactivator complex. Proc. Natl. Acad. Sci. USA 93, 8329-8333. [Europe PMC free article] [Abstract] [Google Scholar]
- Green, S., Walter, P., Kumar, V., Krust, A., Bornert, J.M., Argos, P., and Chambon, P. (1986). Human oestrogen receptor cDNA: sequence, expression and homology to v-erb-A. Nature 320, 134-139. [Abstract] [Google Scholar]
- Hong, H., Kohli, K., Trivedi, A., Johnson, D.L., and Stallcup, M.R. (1996). GRIP1, a novel mouse protein that serves as a transcriptional coactivator in yeast for the hormone binding domains of steroid receptors. Proc. Natl. Acad. Sci. USA 93, 4948-4952. [Europe PMC free article] [Abstract] [Google Scholar]
- Inadera, H., Hashimoto, S., Dong, H.Y., Suzuki, T., Nagai, S., Yamashita, T., Toyoda, N., and Matsushima, K. (2000). WISP-2 as a novel estrogen-responsive gene in human breast cancer cells. Biochem. Biophys. Res. Commun. 275, 108-114. [Abstract] [Google Scholar]
- Johnson, S.R. (1998). Menopause and hormone replacement therapy. Med. Clin. North Am. 82, 297-320. [Abstract] [Google Scholar]
- Kamei, Y., et al. (1996). A CBP integrator complex mediates transcriptional activation and AP-1 inhibition by nuclear receptors. Cell 85, 403-414. [Abstract] [Google Scholar]
- Kuiper, G., and Gustafsson, J. (1997). The novel estrogen receptor-beta sub-type: potential role in the cell- and promoter-specific actions of estrogens and anti-estrogens. Fed. Eur. Biochem. Soc. Lett. 410, 87-90. [Abstract] [Google Scholar]
- Kushner, P.J., Agard, D.A., Greene, G.L., Scanlan, T.S., Shiau, A.K., Uht, R.M., and Webb, P. (2000). Estrogen receptor pathways to AP-1. J. Steroid Biochem. Mol. Biol. 72, 311-317. [Abstract] [Google Scholar]
- Levenson, A.S., Svoboda, K.M., Pease, K.M., Kaiser, S.A., Chen, B., Simons, L.A., Jovanovic, B.D., Dyck, P.A., and Jordan, V.C. (2002). Gene expression profiles with activation of the estrogen receptor alpha-selective estrogen receptor modulator complex in breast cancer cells expressing wild-type estrogen receptor. Cancer Res. 62, 4419-4426. [Abstract] [Google Scholar]
- Love, R., Mazess, R., Barden, H., Epstein, S., Newcomb, P., Jordan, V., Carbone, P., and DeMets, D. (1992). Effects of tamoxifen on bone mineral density in postmenopausal women with breast cancer. N. Engl. J. Med. 326, 852-856. [Abstract] [Google Scholar]
- McDonnell, D.P. (2000). Selective estrogen receptor modulators (SERMs): a first step in the development of perfect hormone replacement therapy regimen. J. Soc. Gynecol. Investig. 7, S10-S15. [Abstract] [Google Scholar]
- McDonnell, D.P., and Norris, J.D. (2002). Connections and regulation of the human estrogen receptor. Science 296, 1642-1644. [Abstract] [Google Scholar]
- McKenna, N.J., Lanz, R.B., and O'Malley, B.W. (1999). Nuclear receptor coregulators: cellular and molecular biology. Endocr. Rev. 20, 321-344. [Abstract] [Google Scholar]
- Onate, S.A., Tsai, S.Y., Tsai, M.J., and O'Malley, B.W. (1995). Sequence and characterization of a coactivator for the steroid hormone receptor superfamily. Science 270, 1354-1357. [Abstract] [Google Scholar]
- Paech, K., Webb, P., Kuiper, G., Nilsson, S., Gustafsson, J.-A., Kushner, P., and Scanlan, T. (1997). Differential ligand activation of estrogen receptors ERa and ERb at API sites. Science 277, 1508-1510. [Abstract] [Google Scholar]
- Pike, A.C., Brzozowski, A.M., Hubbard, R.E., Bonn, T., Thorsell, A.G., Engstrom, O., Ljunggren, J., Gustafsson, J.A., and Carlquist, M. (1999). Structure of the ligand-binding domain of oestrogen receptor beta in the presence of a partial agonist and a full antagonist. EMBO J. 18, 4608-4618. [Europe PMC free article] [Abstract] [Google Scholar]
- Rachez, C., and Freedman, L.P. (2001). Mediator complexes and transcription. Curr. Opin. Cell Biol. 13, 274-280. [Abstract] [Google Scholar]
- Richer, J.K., Jacobsen, B.M., Manning, N.G., Abel, M.G., Wolf, D.M., and Horwitz, K.B. (2002). Differential gene regulation by the two progesterone receptor isoforms in human breast cancer cells. J. Biol. Chem. 277, 5209-5218. [Abstract] [Google Scholar]
- Rogatsky, I., Luecke, H.F., Leitman, D.C., and Yamamoto, K.R. (2002). Alternate surfaces of transcriptional coregulator GRIP1 function in different glucocorticoid receptor activation and repression contexts. Proc. Natl. Acad. Sci. USA 99, 16701-16706. [Europe PMC free article] [Abstract] [Google Scholar]
- Shang, Y., and Brown, M. (2002). Molecular determinants for the tissue specificity of SERMs. Science 295, 2465-2468. [Abstract] [Google Scholar]
- Shang, Y., Hu, X., DiRenzo, J., Lazar, M.A., and Brown, M. (2000). Cofactor dynamics and sufficiency in estrogen receptor-regulated transcription. Cell 103, 843-852. [Abstract] [Google Scholar]
- Shiau, A.K., Barstad, D., Loria, P.M., Cheng, L., Kushner, P.J., Agard, D.A., and Greene, G.L. (1998). The structural basis of estrogen receptor/coactivator recognition and the antagonism of this interaction by tamoxifen. Cell 95, 927-937. [Abstract] [Google Scholar]
- The Writing Group for the PEPI Trial. (1996). Effects of hormone therapy on bone mineral density: results from the postmenopausal estrogen/progestin interventions (PEPI) trial. J. Am. Med. Assoc. 276, 1389-1396. [Abstract] [Google Scholar]
- The Writing Group for the Women's Health Initiative. (2002). Risks and benefits of estrogen plus progestin in healthy postmenopausal women: principal results From the Women's Health Initiative randomized controlled trial. J. Am. Med. Assoc. 288, 321-333. [Abstract] [Google Scholar]
- Torgerson, D.J. (2000). HRT and its impact on the menopause, osteoporosis and breast cancer. Expert Opin. Pharmacother. 1, 1163-1169. [Abstract] [Google Scholar]
- Tzagarakis-Foster, C., Geleziunas, R., Lomri, A., An, J., and Leitman, D.C. (2002). Estradiol represses human T-cell leukemia virus type 1 Tax activation of tumor necrosis factor-alpha gene transcription. J. Biol. Chem. 277, 44772-44777. [Abstract] [Google Scholar]
- Yang, N., Venugopalan, M., Hardikar, S., and Glasebrook, A. (1996). Identification of an estrogen response element activated by metabolites of 17b-estradiol and raloxifene. Science 273, 1222-1224. [Abstract] [Google Scholar]
Articles from Molecular Biology of the Cell are provided here courtesy of American Society for Cell Biology
Full text links
Read article at publisher's site: https://doi.org/10.1091/mbc.e03-06-0360
Read article for free, from open access legal sources, via Unpaywall:
https://europepmc.org/articles/pmc363122?pdf=render
Citations & impact
Impact metrics
Citations of article over time
Alternative metrics
Smart citations by scite.ai
Explore citation contexts and check if this article has been
supported or disputed.
https://scite.ai/reports/10.1091/mbc.e03-06-0360
Article citations
Crosstalk of methylation and tamoxifen in breast cancer (Review).
Mol Med Rep, 30(4):180, 12 Aug 2024
Cited by: 0 articles | PMID: 39129315 | PMCID: PMC11338244
Review Free full text in Europe PMC
Multi-Anticancer Activities of Phytoestrogens in Human Osteosarcoma.
Int J Mol Sci, 24(17):13344, 28 Aug 2023
Cited by: 1 article | PMID: 37686148 | PMCID: PMC10487502
Review Free full text in Europe PMC
Metamifop as an estrogen-like chemical affects the pituitary-hypothalamic-gonadal (HPG) axis of female rice field eels (Monopterus albus).
Front Physiol, 14:1088880, 19 Jan 2023
Cited by: 1 article | PMID: 36744025 | PMCID: PMC9892845
Estrogen-mediated mechanisms in hypertension and other cardiovascular diseases.
J Hum Hypertens, 37(8):609-618, 01 Nov 2022
Cited by: 18 articles | PMID: 36319856 | PMCID: PMC10919324
Review Free full text in Europe PMC
Brain region- and sex-specific transcriptional profiles of microglia.
Front Psychiatry, 13:945548, 24 Aug 2022
Cited by: 17 articles | PMID: 36090351 | PMCID: PMC9448907
Go to all (146) article citations
Data
Data behind the article
This data has been text mined from the article, or deposited into data resources.
BioStudies: supplemental material and supporting data
Nucleotide Sequences (Showing 42 of 42)
- (3 citations) ENA - Y00503
- (2 citations) ENA - AJ001685
- (2 citations) ENA - AJ001684
- (2 citations) ENA - AF100780
- (1 citation) ENA - X00948
- (1 citation) ENA - N90755
- (1 citation) ENA - D38449
- (1 citation) ENA - AF032885
- (1 citation) ENA - U54804
- (1 citation) ENA - AI743745
- (1 citation) ENA - U43916
- (1 citation) ENA - U59863
- (1 citation) ENA - M92843
- (1 citation) ENA - AL080111
- (1 citation) ENA - M87770
- (1 citation) ENA - AF017786
- (1 citation) ENA - AF042166
- (1 citation) ENA - U61836
- (1 citation) ENA - D15049
- (1 citation) ENA - L23808
- (1 citation) ENA - M14539
- (1 citation) ENA - X01683
- (1 citation) ENA - K02215
- (1 citation) ENA - S81914
- (1 citation) ENA - AF089750
- (1 citation) ENA - U37283
- (1 citation) ENA - U16261
- (1 citation) ENA - AA532495
- (1 citation) ENA - D13413
- (1 citation) ENA - AL050159
- (1 citation) ENA - X69433
- (1 citation) ENA - M55153
- (1 citation) ENA - M59830
- (1 citation) ENA - X14885
- (1 citation) ENA - M32011
- (1 citation) ENA - X52001
- (1 citation) ENA - AL021977
- (1 citation) ENA - M62424
- (1 citation) ENA - U08021
- (1 citation) ENA - M69199
- (1 citation) ENA - M62400
- (1 citation) ENA - Y07909
Show less
Similar Articles
To arrive at the top five similar articles we use a word-weighted algorithm to compare words from the Title and Abstract of each citation.
Estrogen and the selective estrogen receptor modulator (SERM) protection against cell death in estrogen receptor alpha and beta expressing U2OS cells.
Mol Cell Endocrinol, 289(1-2):38-48, 25 Mar 2008
Cited by: 28 articles | PMID: 18455292
Estrogen receptor alpha and beta heterodimers exert unique effects on estrogen- and tamoxifen-dependent gene expression in human U2OS osteosarcoma cells.
Mol Endocrinol, 19(6):1555-1568, 31 Mar 2005
Cited by: 84 articles | PMID: 15802376
Cell type- and estrogen receptor-subtype specific regulation of selective estrogen receptor modulator regulatory elements.
Mol Cell Endocrinol, 299(2):204-211, 18 Nov 2008
Cited by: 17 articles | PMID: 19059307 | PMCID: PMC3420066
Molecular mechanisms of estrogen action: selective ligands and receptor pharmacology.
J Steroid Biochem Mol Biol, 74(5):279-285, 01 Nov 2000
Cited by: 154 articles | PMID: 11162936
Review
Funding
Funders who supported this work.
NIDDK NIH HHS (2)
Grant ID: R01 DK061966
Grant ID: DK-061966