Abstract
Free full text

Membrane-Anchored Serine Proteases in Health and Disease
Abstract
Serine proteases of the trypsin-like family have long been recognized to be critical effectors of biological processes as diverse as digestion, blood coagulation, fibrinolysis, and immunity. In recent years, a subgroup of these enzymes has been identified that are anchored directly to plasma membranes, either by a carboxy-terminal transmembrane domain (Type I), an amino-terminal transmembrane domain with a cytoplasmic extension (Type II or TTSP), or through a glycosylphosphatidylinositol (GPI) linkage. Recent biochemical, cellular, and in vivo analyses have now established that membrane-anchored serine proteases are key pericellular contributors to processes vital for development and the maintenance of homeostasis. This chapter reviews our current knowledge of the biological and physiological functions of these proteases, their molecular substrates, and their contributions to disease.
I. Introduction
Proteolytic enzymes comprise over 2% of the known proteome, and their participation in many essential biological processes is well established. The serine proteases constitute one of the largest families of proteolytic enzymes and are well recognized for their pivotal roles in physiological processes as diverse as development, digestion, coagulation, inflammation, and immunity. These enzymes share a common catalytic mechanism for selective cleavage of specific substrates and are frequently involved in consecutive proteolytic reactions or protease cascades, where one protease precursor or zymogen is the substrate for an active protease. This shared mechanism confers the advantage that a single signal may be specifically and irreversibly amplified every time a downstream zymogen is activated, providing the capacity for unleashing a burst of proteolytic potential.
Most of the well-characterized members of the S1 family of serine proteases are either secreted enzymes or exocytosed from secretory vesicles into the extracellular environment. Trypsin and chymotrypsin, the main intestinal digestive enzymes, are prototype members of the S1 family. Over the past 10 years, a structurally distinct group of S1 serine proteases, termed broadly as the membrane-anchored serine proteases, has emerged that are synthesized with amino- or carboxy-terminal extensions that serve to anchor their serine protease catalytic domains directly at the plasma membrane1, 2 (Fig. 1 ).

Domain structures of the human membrane-anchored serine proteases. Structures are grouped according to similarity in domain structure to each other. Consensus domains are as indicated at the bottom of the figure. The location of each protein domain (amino acid numbering) is indicated above the domain. Amino and carboxy termini are as indicated. Protease domain: serine protease domain; activation domain: pro-domain; TM: transmembrane domain; GPI anchor: glycosylphosphatidylinositol linkage domain; LDLRA: LDL receptor class A domain; MAM: meprin, A5 antigen, and receptor protein phosphatase μ domain; CUB: Cls/Clr, urchin embryonic growth factor and bone morphogenetic protein-1; SEA: sea urchin sperm protein, enteropeptidase, agrin domain; Fz: frizzled domain; SR: Group A scavenger receptor domain.
The largest group of membrane-anchored serine proteases is the Type II transmembrane serine proteases or TTSPs.1 These proteases are synthesized with an amino-terminal signal anchor that is not removed during synthesis, but serves as a transmembrane domain that positions the protease in the plasma membrane with a cytoplasmic amino-terminal domain of variable length (20–160 amino acids) and the catalytic serine protease domain at the carboxy-terminus.1 These serine proteases are synthesized as single-chain precursors or zymogens; activation produces a two-chain form with the chains linked by a disulfide bridge, so that the active enzyme remains membrane bound. Nineteen human TTSPs have been identified and may be categorized into four subfamilies: Hepsin/TMPRSS, Matriptase, HAT/DESC, and Corin3, 4 (Fig. 1). Mammalian orthologs, as well as different isoforms between humans and rodents, exist for many if not all of the TTSPs.4, 5, 6 There also exist two nonmammalian TTSPs, Drosophila stubble-stubbloid (st-sb)7 and corin.8
In contrast to the TTSPs, additional membrane-anchored serine proteases of the S1 family each possess an amino-terminal signal peptide and enter the secretory pathway. These enzymes are also synthesized as zymogens, with an amino-terminal extension that acts as a pro-peptide, requiring proteolytic cleavage to generate the active enzyme. The Type I transmembrane serine protease, tryptase γ1, is the only human membrane-anchored serine protease synthesized with a carboxy-terminal hydrophobic extension that serves as a transmembrane domain.9, 10 The carboxy-terminal extensions of prostasin and testisin are modified posttranscriptionally with a glycosylphosphatidylinositol (GPI) linkage that anchors these proteases in the plasma membrane.11, 12, 13, 14, 15
The membrane-anchored serine proteases are proving to be key components of the cell machinery for activation of precursor molecules in the pericellular microenvironment, with several playing vital roles during development and the maintenance of homeostasis. There is also growing evidence for their participation in the pathogenesis of inflammatory and neoplastic diseases. Endogenous protein substrates targeted by membrane-anchored serine proteases include peptide hormones, growth and differentiation factors, receptors, enzymes, adhesion molecules, and viral coat proteins.16 A number of insights into our understanding of the unique physiological functions of the membrane-anchored serine proteases and their involvement in human pathology have come from a combination of biochemical analyses, animal models, and human patient studies. However, our current understanding of the impact of the membrane-anchored serine proteases on many biological, physiological, and pathological processes is far from complete. This chapter provides a historical perspective on the discovery of these enzymes, current knowledge of their activities and their regulation, and the functional consequences of the activities of these proteases in mammalian physiology and disease. For the interested reader, several other reviews have focused on different aspects of their nomenclature, classifications into subgroups, gene structure and chromosomal localization, tissue- and cell-specific distribution, and biochemical properties.1, 2, 3, 4, 16, 17
II. Structural Features
All of the membrane-anchored serine proteases have membrane-anchoring domains and structurally conserved serine protease catalytic domains. The TTSPs have additional extracellular stem regions that separate the catalytic domains from their transmembrane domains. The extracellular regions of the membrane-anchored serine proteases are believed to be essential to the biological and physiological functions ascribed to these enzymes.
A. Catalytic Domains
The zymogen forms of the membrane-anchored serine proteases are activated by proteolytic cleavage following an arginine or lysine amino acid present in a highly conserved activation motif separating the pro- and catalytic domains. The catalytic mechanism of the membrane serine proteases involves a catalytic triad of three amino acids, serine (nucleophile), aspartate (electrophile), and histidine (base), present in highly conserved sequence motifs. While the geometric orientations of these amino acid residues are similar, the protein folds are variable, which contribute to their selective substrate specificities. The catalytic reaction follows a two-step mechanism for hydrolysis of substrates in which a covalently linked enzyme-peptide intermediate is formed, with the loss of a peptide fragment.18 This acylation step is followed by a deacylation step which occurs by a nucleophilic attack on the intermediate by water, resulting in hydrolysis of the peptide.
Some insights into the structural features that contribute to the unique catalytic and substrate specificities of the membrane-anchored serine proteases have been obtained through comparative analyses of amino acid sequences1, 2, 19 combined with tertiary structural analyses.20, 21, 22, 23, 24, 25, 26 Consistent with the family of S1 serine proteases, each catalytic domain possesses two adjacent, six-stranded β-barrel domains that are connected by three trans-domain segments. The catalytic triad amino acids are located along the junction between the two barrels, with the active site cleft running perpendicular to this junction.27 The size, shape, and charge distribution within the formed binding pocket of the active enzyme are determinants of substrate specificity. These pockets are defined by differing substrate-binding subsites (e.g., S4–S2′) and loop regions that surround the active site cleft.23
The specificity for cleavage of substrates with the positively charged amino acid residues, lysine or arginine, in the P1 position (the position directly preceding the cleaved peptide bond) is conferred by the presence of a conserved aspartate residue at the bottom of the binding pocket of all of the activated enzymes.2 The rate of cleavage is influenced by the amino acid residues surrounding the P1 residue, numbered P1 to Pn, counting outward from the amino-terminal side of the peptide bond that is cleaved during hydrolysis, and numbered P1′ through Pn′ from the carboxy-terminal side.28 In recent years, recombinant catalytic domains of several of the membrane-anchored serine proteases have been produced in various laboratories and peptide screening assays applied to quantitatively identify cleavage preferences for the P1 and P1′ amino acids, and surrounding amino acid positions.29, 30, 31, 32, 33, 34, 35
Several of the biochemically purified TTSPs rapidly undergo autocatalytic activation in vitro (matriptase29, 36 matriptase-2,37 hepsin,38 TMPRSS2,39 TMPRSS3,40 TMPRSS4,41 HAT-like 3/TMPRSS11C42). Thus, mutation of active site catalytic triad residues of several of these TTSPs prevents activation site cleavage.3, 36, 42, 43 It has been speculated that autoactivation of these TTSPs could contribute to the initiation of proteolytic cascades at the cell membrane, although the signals that induce internal cleavage or autoactivation in vivo have not yet been defined.
B. Extracellular “Stem” Regions
While the catalytic domains of the Type I and GPI-anchored serine proteases lie directly proximal to the membrane anchoring domain, the TTSPs are characterized by the presence of up to 11 extracellular structural domains present between their catalytic domains and the transmembrane domain (Fig. 1). These “stem” regions appear to serve as regulatory and/or binding interaction domains,1 and are believed to variously contribute to the cellular localization, activation, inhibition, and/or substrate specificity of these enzymes.44 The most common stem region structural domain is the low-density lipoprotein (LDL) receptor class A domain (LDLRA): corin contains eight, matriptase contains four, enteropeptidase two, and TMPRSS2 and TMPRSS4 one each of these domains (Fig. 1). In addition to the LDLRA domains, there are Group A scavenger receptor (SR) domains, frizzled domains, Cls/Clr, urchin embryonic growth factor and bone morphogenic protein 1 (CUB) domains, sea urchin sperm protein, enterokinase, agrin (SEA) domains, and meprin, A5 antigen, and receptor protein phosphatase μ (MAM) domains. The specific contributions of each stem domain to TTSP proteolytic activities have not yet been well defined, although for several of the TTSPs, the stem domain is required for efficient cleavage of their physiological substrates.45, 46
Targeted release of the extracellular domain, or ectodomain shedding, occurs for several of the membrane-anchored serine proteases, enabling the release of the soluble catalytic domain into the extracellular space. Soluble shed protease forms have been reported for enteropeptidase, HAT, matriptase, matriptase-2, and prostasin.11, 47, 48, 49
C. Membrane-Anchoring Domains
Surface localization studies demonstrate that membrane-anchored serine proteases normally localize to the cell surface and are differentially distributed on apical or basolateral surfaces of polarized cells in patterns unique for each protease.3, 4 Studies indicate that the TTSP transmembrane domains are dispensable for the catalytic activities of many of the TTSPs, including enteropeptidase, matriptase, hepsin, corin, and MSPL.4 It appears that the function of the transmembrane domain in TTSPs is not to enhance their catalytic activities but to target the TTSPs to plasma membranes and localize their activities at specific sites. Similarly, the GPI-anchored serine proteases are found to associate with lipid raft microdomains at cell surfaces, but do not require these anchors for catalytic activity. Loss of membrane polarity, such as that in tumors, has been associated with redistribution and/or mislocalization of several of the membrane-anchored serine proteases.50, 51 In this regard, the significance of cell membrane association for the membrane-anchored serine proteases differs from that for blood-clotting proteases.
III. Regulation by Endogenous Inhibitors
As is the case with all serine proteases, enzyme activities must be tightly regulated in order to prevent inappropriate and frequently destructive proteolysis. The catalytic activities of the membrane-anchored serine proteases are regulated by endogenous protease inhibitors, specifically the Kunitz-type transmembrane serine protease inhibitors, hepatocyte growth factor (HGF) activator inhibitor (HAI)-1/SPINT1 and HAI-2/SPINT2, and several of the serine protease inhibitor family known as serpins. Kunitz-type inhibitors are a class of serine protease inhibitors present in all metazoa, whose prototype is the bovine pancreatic trypsin inhibitor (BPTI). They are competitive inhibitors acting in a substrate-like manner and form very stable complexes of 1:1 stoichiometry with their target enzymes, inhibiting their activity.52 Matriptase,53, 54, 55, 56 hepsin,57 and prostasin31, 48, 57, 58 are regulated by the Kunitz inhibitors, HAI-1 and/or HAI-2.
Serpin-type inhibitors utilize an irreversible conformational mechanism presenting a “pseudo-substrate” exposed binding loop to the protease which, upon cleavage, forms a covalent complex with the target protease.59, 60 The serpins α1-antitrypsin, α2-antiplasmin, antithrombin III, protein C inhibitor, PAI-1, and protease nexin I are effective inhibitors of various recombinant catalytic domains of the membrane-anchored serine proteases (reviewed in Ref. 16).
IV. The Type I Transmembrane Serine Protease
A. Tryptase γ1
Tryptase γ1 or transmembrane tryptase (TMT) was identified as a major granule constituent of numerous populations of human and mouse mast cells.9, 10, 61, 62 The gene that encodes tryptase γ1 lies on human chromosome 16p13.3, a large locus that contains 14 serine protease-like genes.9, 63 Expression of tryptase γ1 is restricted to cells of hematopoietic origin and has been studied in mast cells.62 The Type I transmembrane domain at the carboxy-terminus of tryptase γ1 causes its cellular retention when mast cells are induced to release the contents of their secretory granules, at which time it reaches the external face of the plasma membrane.62
The physiological functions of tryptase γ1 remain unknown. Mast cells play beneficial immunosurveillance roles in host defense, particularly during parasitic infections, and are also implicated in asthma and other pathological conditions.64, 65 Administration of recombinant tryptase γ1 into the trachea of mice promotes airway hyperresponsiveness and increased expression of interleukin-13 in bronchial alveolar lavage fluids of these animals. This effect was not obtained in STAT6- and IL-4Rα-null mice, indicating that tryptase γ1 exerts its adverse effect in the lung in this animal model primarily by inducing the expression of IL-13, a central mediator of allergic asthma.
Tryptase γ1 has been expressed as a recombinant catalytic domain and its substrate specificity characterized using combinatorial peptide substrate libraries.33 Tryptase γ1 prefers multibasic residues in the P4–P1 positions and has a strong preference for aromatic residues in the P2 position.33 It has also been shown to be rapidly inactivated by the human plasma serpin α1-antitrypsin62 and a submicromolar synthetic inhibitor,33 in vitro.
V. The Type II Transmembrane Serine Proteases
A. HEPSIN/TMPRSS Subfamily
The HEPSIN/TMPRSS (transmembrane protease/serine) subfamily has seven members comprising hepsin, TMPRSS2, TMPRSS3, TMPRSS4, TMPRSS5/spinesin, MSPL (mosaic serine protease large-form), and enteropeptidase.4 All of these enzymes possess a Group A SR domain in their stem region, preceded by a single LDLRA domain in TMPRSS2, TMPRSS3, TMPRSS4, and MSPL or by an array of SEA, LDLRA, CUB, and MAM domains in enteropeptidase (Fig. 1).
1. Enteropeptidase
Enteropeptidase is an intestinal protease discovered by Ivan Pavlov in Russia.66 Using surgically modified dogs, Pavlov and colleagues studied the digestive system and found that pancreatic proteases were made as inactive forms. Upon entering the gut, latent pancreatic proteases were activated by another enzyme in the upper section of the intestine. Such an activation mechanism, which occurs outside of the pancreas, is important to prevent autoactivation of pancreatic proteases, thereby avoiding harmful tissue damage. Pavlov named the intestinal enzyme enterokinase for its transformative activation activity. Currently, the enzyme is called enteropeptidase to reflect its proteolytic, but not kinase, activity.
Initial biochemical studies on enteropeptidase were carried out in the early 1900s, notably by Ernst Waldschmidt-Leitz and colleagues at the Biochemical Institute of the German Technical College in Prague.67 Porcine enteropeptidase was partially purified from duodenal fluids and shown to activate trypsinogen from pancreatic extracts. More kinetic studies were done by Moses Kunitz at the Rockefeller Institute for Medical Research in Princeton, New Jersey, demonstrating enzymatic conversion of crystalline trypsinogen to trypsin using purified porcine enteropeptidase.68, 69 By the 1970s, more advanced methods were used to purify enteropeptidase from porcine, bovine, and human intestinal mucosa.70, 71 The protein was found to have a heavy chain of approximately 120 kDa and a light chain of approximately 35 kDa connected by a disulfide bond. Such a two-chain structure was known for chymotrypsin and other serine proteases. Enteropeptidase cleaves trypsinogen at the activation site, DDDDK↓I. The activity is inhibited by Kunitz pancreatic trypsin inhibitor, BPTI, and small molecule serine protease inhibitors, such as diisopropyl fluorophosphate (DFP), p-aminobenzamidine, and benzamidine, but not by chicken ovomucoid, soybean trypsin inhibitor, chymostatin, pepstatin A, or bestatin.66
The partial amino-terminal sequence of the bovine enteropeptidase light chain was determined by Edman degradation, revealing its homology to other trypsin-like serine proteases.72 This sequence information helped to clone human, bovine, and porcine enteropeptidase cDNAs.73, 74, 75, 76 The open reading frame of human enteropeptidase cDNA encodes a Type II transmembrane protein of 1019 amino acids with a calculated mass of 113 kDa and 17 potential N-linked glycosylation sites. The overall protein domain structure is shown in Fig. 1. Within the protease domain, the activation cleavage site, the catalytic triad, and the key cysteine residues are well conserved, indicating that enteropeptidase and other trypsin-like proteases have similar three-dimensional structures and comparable activation and catalytic mechanisms.
The human PRSS7 gene, encoding enteropeptidase, is located on chromosome 21q21.73 The gene has 25 exons and spans approximately 90-kb in length. Enteropeptidase mRNA is expressed mostly in the duodenum and, at lower levels, in the proximal segment of jejunum, consistent with Pavlov's finding that the enzyme was in the upper part of the small intestine. In these tissues, enteropeptidase mRNA is expressed in the enterocytes and goblet cells of the villus.77 As a transmembrane protein, enteropeptidase is expected to remain at the site of expression, consistent with the previous observation that its activity was associated with the brush border of enterocytes.78, 79 Low levels of enteropeptidase mRNA were detected in other tissues, including stomach, colon, brain, and skin. The biological significance, if any, of its expression outside the digestive system remains unknown.
Enteropeptidase is anchored on the cell surface through its amino-terminal transmembrane domain. Other structural elements also play a role in its membrane targeting. The mucin-like repeats in the SEA domain and N-glycans in the protease domain have been found necessary for apical sorting.80, 81 Like many membrane proteins, enteropeptidase is shed from the cell surface. Hadorn et al. showed that enteropeptidase was released into the small intestine lumen when brush-border membranes were treated with bile-acids or cholescystokinin–pancreozymin.82 To date, enzymes responsible for enteropeptidase shedding have not been characterized. It remains to be determined whether the shedding represents a physiological mechanism regulating enteropeptidase activity in the gut.
The mechanism responsible for the activation of the enteropeptidase zymogen is unclear. In transfected cells, enteropeptidase was expressed as a single-chain molecule and no autoactivation was observed, indicating that other proteases are required to activate enteropeptidase.80 Trypsin activates enteropeptidase efficiently, suggesting a possible mechanism of reciprocal activation between enteropeptidase and trypsin.66 For such an activation cycle to start, however, at least a fraction of enteropeptidase needs to be activated before trypsinogen enters the gut. Zamolodchikova et al. identified a novel serine protease, duodenase, from bovine duodenal mucosa.83 The enzyme, which is approximately 30 kDa and consists of 226 amino acids, is expressed in the secretory epithelial cells of Brunner's glands in the proximal segment of the duodenum.84 Duodenase has a preferred cleavage sequence with Lys at P1 and Pro at P2. It activated recombinant enteropeptidase, although the rate of the activation was approximately 100 times slower than that of trypsin.85 It remains to be firmly established whether duodenase is a physiological enteropeptidase activator. More recently, other proteases have been shown to modulate enteropeptidase activity. For example, beta-site APP-cleaving enzyme1 (BACE1) was detected in pancreatic islets and shown to inactivate enteropeptidase by cleavage of its light chain.86 This inactivation may represent a defense mechanism to inhibit enteropeptidase that enters the pancreatic duct accidentally, thereby preventing trypsinogen activation in the pancreas.
One of the remarkable features of enteropeptidase is its unique substrate specificity, which recognizes Lys at P1 and a cluster of four Asp residues at P2–P5. Within this recognition sequence, a Lys or an Arg residue at P1 and Lys residues at P2 and P3 appear to be most important for efficient cleavage.87 The structural determinants for enteropeptidase substrate specificity have been localized in its protease domain. There is a group of four conserved basic residues, R/KRRK at positions 96–99, which were suspected to interact with the acidic P2–P5 residues in the trypsinogen activation site.74 In a crystal structure of bovine enteropeptidase light chain, Lys99 residue was found to have extensive contacts with the P2 and P4 Asp residues.22 Lys99 residue is conserved in enteropeptidase from many species. Substitution of Lys99 with Ala by mutagenesis prevented enteropeptidase from activating trypsinogen. In contrast, substituting Lys96, Arg97, and Arg98 residues for bovine enteropeptidase activity had less significant effects.22 The unique enteropeptidase substrate specificity has been exploited in protein engineering. The DDDDK↓I sequence is frequently used in recombinant proteins that require specific cleavage.
Enteropeptidase deficiency impairs food digestion and absorption.88 To date, a number of patients who had low or undetectable enteropeptidase activity in intestinal biopsies or duodenal fluid samples have been identified. These patients suffer from diarrhea, vomiting, edema, anemia, and hypoproteinemia. Consequently, the patients fail to gain weight in early infancy. Genetic studies have shown deletion or nonsense mutations in the PRSS7 gene in enteropeptidase-deficient patients.66 For these patients, treatment with pancreatic extracts, which contain active trypsin, helps to initiate proteolytic reactions in the gut. Usually, the therapy is effective for the patients to absorb nutrients and gain weight. Apparently, the digestive enzyme reactions, once started, are self-sustained. Pancreatic extracts can be discontinued over time without causing gastrointestinal symptoms in these patients.89
2. Hepsin
Hepsin was originally cloned in Earl Davie's Laboratory at the University of Washington. Leytus et al. screened human liver cDNA libraries, which were rich in serine proteases.90 A novel cDNA encoding a trypsin-like serine protease was identified by degenerate oligonucleotides. The protease was named hepsin for its hepatic expression. Rat and mouse hepsin cDNAs were isolated subsequently, which share high sequence homology with human hepsin.91, 92 The full-length human hepsin cDNA, approximately 1.8-kb in length, encodes a polypeptide of 417 amino acids with a calculated mass of 45 kDa. Its protein domain structure is shown in Fig. 1. There is one potential N-linked glycosylation site at residue 112 in the SR-like domain.
The human HPN gene, encoding hepsin, is on chromosome 19 at q11-13.2 and has 14 exons. Hepsin mRNA expression is abundant in the liver.93 Other tissues such as the kidney, pancreas, stomach, prostate, and thyroid express low levels of hepsin mRNA. The promoter sequence of the mouse Hpn gene has been characterized.92 In a 274-bp sequence upstream of the transcription initiation site, several potential binding sites for transcription factors such as SP1, AP2, C/EBP, LF-A1, and E-box have been identified. This sequence showed strong promoter activities when tested in human HepG2 cells, indicating that it retains the essential elements required for hepatic expression.
In hepatocytes, hepsin is synthesized as a single-chain zymogen. Human hepsin purified from hepatocytes had an apparent mass of approximately 51 kDa on Western blots.93 A similar molecular mass was found for native rat and recombinant human hepsin. During protein purification, human and rat hepsin were readily activated to become a two-chain molecule. It is unclear whether the activation was mediated by autocleavage or other proteases in hepatocytes. The membrane association and topology of hepsin have been confirmed by immunostaining and proteolytic digestion.
In peptide substrate-based assays, hepsin favors basic residues at the P1 position. Thr/Leu/Asn, Gln/Lys, and Pro/Lys residues are favored at the P2, P3, and P4 positions, respectively.31 This substrate profile does not appear to be unique. Somoza et al. have solved the crystal structure of a soluble human hepsin that included the SR-like and protease domains.21 As expected, hepsin protease domain has an architecture of two six-stranded β barrels. There are several structurally distinct loops, including an especially large one between residues 241 and 256, which may interact with its substrates. The activity of hepsin is inhibited by nonspecific inhibitors such as leupeptin, aprotinin, antipain, 4-amidiophenylmethylsulfonyl fluoride, N α-tosyl-l-lysine chloromethyl ketone, soybean trypsin inhibitor, and antithrombin III.94 No inhibition was detected with EDTA, indicating that divalent ions are not required for hepsin activity toward peptide substrates. More recently, HAI-1 and HAI-2 have been reported to be potent hepsin inhibitors.31, 95
While many proteins are activated or cleaved by hepsin in vitro,96, 97, 98, 99 the physiological function of hepsin remains unclear. These proteins include blood clotting factors VII, IX, and XII; pro-urokinase; pro-HGF; liver microsomal glutathione transferase; matriptase; prostasin; epidermal growth factor receptor; and laminin. It is unknown how many of them are hepsin substrates in vivo. Studies have suggested that hepsin may play a role in blood coagulation, hepatocyte proliferation/growth, and embryonic development.43, 100, 101 Such functions, however, have not been validated in vivo, as hepsin null mice are fertile, grow normally, and exhibit no spontaneous bleeding.102, 103 Unexpectedly, however, hepsin appears to be important in normal hearing. Guipponi et al. detected hepsin mRNA expression in the inner ear.104 Hepsin null mice have been found to have abnormal cochlea, reduced myelin protein expression in the auditory nerve, and severely impaired hearing. The biochemical basis for hepsin function in cochlear development has not been determined. Apparently, hepsin null mice have low levels of plasma thyroxine, a hormone important for cochlear development.104 It is unknown whether impaired hearing in hepsin null mice is a result of thyroid hormone deficiency.
Multiple lines of evidence suggest a potential role of hepsin in human prostate cancer growth and progression. Microarray studies detected high levels of hepsin mRNA in human prostate cancers.105, 106, 107, 108 Elevated hepsin mRNA expression appears to correlate with the disease severity. Single nucleotide polymorphisms (SNPs) in or near the human HPN gene are associated with prostate cancer susceptibility and tumor aggressiveness.109 In functional studies, Xuan et al. showed that antibodies neutralizing hepsin protease activity did not block prostate or ovarian cancer cell growth in culture but inhibited their invasion in a Matrigel basement membrane.110 In other studies, however, prostate or other cancer cell lines stably transfected with hepsin cDNA were less invasive in culture or nude mice. Because stably transfected cells often undergo phenotypic changes during selection, it is difficult to determine whether observed differences were related to different levels of hepsin expression. To circumvent this problem, Klezovitch et al. created transgenic mice with high levels of hepsin in prostate epithelium, mimicking hepsin overexpression in prostate cancer patients.111 The results show that hepsin overexpression did not affect cell proliferation but altered basement membrane structures in prostates in these mice. When the mice were crossed with a nonmetastasizing prostate cancer model, prostate cancer cells became more invasive, causing metastasis in the liver, lung, and bone. Thus, hepsin promotes prostate cancer progression and metastasis in vivo, suggesting that hepsin inhibitors may be developed to treat prostate cancer in patients.
3. TMPRSS2
TMPRSS2 cDNA was originally cloned by exon trapping in a gene mapping study of human chromosome 21.112 The TMPRSS2 gene, located at human chromosome 21q22.3,112 is approximately 44 kb in length and includes 14 exons.113 The full-length TMPRSS2 cDNA encodes a polypeptide of 492 amino acids, with a domain structure shown in Fig. 1. Mouse TMPRSS2, also called epitheliasin, was isolated from a kidney cDNA library.114 Human TMPRSS2 mRNA is expressed in many tissues, including the prostate, breast, bile duct, kidney, colon, small intestine, pancreas, ovary, salivary gland, stomach, and lung.114, 115 In these tissues, TMPRSS2 protein and mRNA are mostly in the epithelial cells. In human prostate cancers, TMPRSS2 protein is localized on the apical membrane of secretory epithelia and in the lumen of the glands.39 Similar luminal staining patterns are found in human colon cancers. Many human prostate- and colon-derived cells, including APC-4, LNCaP, LoVo, T84, and Colo-205, also express TMPRSS2 mRNA.39
In vitro-translated TMPRSS2 protein is detected as a zymogen of approximately 54 kDa,39 whereas native and recombinant human TMPRSS2 proteins have a higher molecular mass (~ 60–70 kDa) due to N-linked glycosylation.39, 116 In human prostate cancer tissues or cells, approximately 32-kDa TMPRSS2 protease domain may also be detected, suggesting that the protease is partially activated. This cleavage appears to be due to TMPRSS2 autoactivation, as active site mutation prevented the cleavage of TMPRSS2 in transfected HEK 293T cells.39 To date, TMPRSS2 substrate specificity and catalytic properties have not been well characterized.
The physiological roles of TMPRSS2 are currently unknown. In a Xenopus oocyte expression system, TMPRSS2 expression reduced epithelial sodium channel (ENaC) current and protein levels.117 In addition, TMPRSS2 activates influenza virus by cleaving hemagglutinin, suggesting that the enzyme may contribute to virus invasion of human airways.118, 119 Secreted forms of TMPRSS2 have recently been found in human seminal prostasomes, suggesting its potential role in regulating sperm function.116 In prostate cancer cells, TMPRSS2 was shown to activate PAR-2.120 It is unclear whether these activities are critical in vivo. TMPRSS2 null mice are viable and fertile with no reported abnormalities.121
The human TMPRSS2 gene promoter has a 15-bp androgen response element at position − 148 relative to the putative transcription start site. Consistently, TMPRSS2 mRNA expression is elevated in androgen-stimulated prostate cancer (LNCaP) cells.115 The upregulation of TMPRSS2 mRNA by androgen appears to be mediated by the androgen receptor.39 Androgen treatment is also reported to increase TMPRSS2 zymogen activation in cell culture and in a mouse xenograft model, suggesting that TMPRSS2 may contribute to prostate cancer development and progression in an androgen-dependent manner.
Frequent gene fusions between TMPRSS2 and members of the E26 transformation specific (ETS) transcription factor family have been identified in many prostate cancer patients.122 In these patients, the 5′-untranslated region of TMPRSS2 is fused with the transcription factor ERG or ETV genes. As a result, the androgen-responsive promoter elements of TMPRSS2 drive the expression of the ETS family transcription factors to promote prostate cancer progression and invasion. Among these chromosomal rearrangements, TMPRSS2–ERG gene fusions are most frequent, accounting for approximately 50% of prostate cancers. Molecular studies show that abnormal ERG expression disrupts normal androgen receptor signaling and activates epigenetic programs, thereby inducing tumorigenesis.123 Although ERG enhances the expression of many proteases, including urokinase-type plasminogen activator (uPA), matrix metalloproteinase (MMP)-3, and MMP9, TMPRSS2 protease activity is unlikely to have a direct role in ERG downstream events, because the TMPRSS2 protease domain coding sequence is never included in the fusion genes.124 Studies have shown that in prostate cancers, ETS transcription factor genes are also fused to other genes that contain androgen-responsive elements.125 These rearrangements have been attributed recently to androgen-induced co-recruitment of the DNA-processing enzyme topoisomerase 2B to sites of genomic breakpoints.126
4. TMPRSS3
TMPRSS3 was identified as a novel serine protease overexpressed in ovarian cancers and was originally named TADG-12 (tumor-associated differentially expressed gene-12).127 Subsequent reports showed that this protease was also overexpressed in pancreatic and breast cancers. Independently, the gene encoding TMPRSS3 was identified as a candidate gene located within the disease locus (DFNB10) for autosomal recessive deafness,128 previously mapped by linkage analysis to chromosome 21q22.129 The human TMPRSS3 is approximately 24 kb in length and contains 13 exons.130 The approximately 2.4-kb TMPRSS3 cDNA encodes a polypeptide of 454 amino acids, with an overall domain structure similar to TMPRSS2 (Fig. 1). In fact, these two proteins share approximately 63% sequence similarities. TMPRSS3 mRNA is expressed in a variety of tissues, including the kidney, lung, colon, thymus, stomach, and cochlea.130
TMPRSS3 mutations have been found in patients with congenital and childhood onset autosomal recessive deafness. Scott et al. first identified an 8-bp deletion and insertion of 18 β-satellite tandem repeats in the TMPRSS3 gene in a Palestinian family with congenital deafness.128 In the same study, a second splice site mutation in the TMPRSS3 gene causing a frameshift was found in a Pakistani family with childhood-onset deafness. To date, deletion, insertion, frameshift, and nonsense TMPRSS3 gene mutations have been reported in over 20 patient families with nonsyndromic autosomal recessive deafness.130 Most mutations occur in exons coding for the LDL receptor repeat, SR repeat, or protease domain, resulting in complete or near complete loss of the protease activity. These studies demonstrate that defects in the TMPRSS3 gene are responsible for hearing loss in these patient families.
Biochemical mechanisms by which TMPRSS3 regulates normal hearing remain unknown. Analogous to hepsin, TMPRSS3 mRNA is expressed in the cochlea.131 Considering that hepsin null mice have severely impaired hearing, it is possible that the TTSPs participate in a proteolytic pathway required for normal cochlear structure and/or hearing function. ENaC is known to regulate Na+ levels in the perilymph of the inner ear. Guipponi et al. have shown that TMPRSS3 activates ENaC in vitro and that most naturally occurring mutations prevented TMPRSS3 activation in cell-based experiments.40 It is unclear, however, whether ENaC is a physiological TMPRSS3 substrate in the inner ear. It has been reported that pseudohypoaldosteronism type 1 patients, who are homozygous for mutations in the ENaCα subunit, do not suffer hearing loss,132 suggesting that TMPRSS3 may act on other substrates in vivo.
5. TMPRSS4
TMPRSS4, initially called TMPRSS3 and also known as CAP2, was originally identified in pancreatic cancers.133 The gene encoding TMPRSS4 is located on human chromosome 11q23.3. Human TMPRSS4 protein consists of 437 amino acids and a domain structure, as shown in Fig. 1. TMPRSS4 mRNA is expressed in the pancreas, lung, stomach, colon, kidney, skin, and eye.131, 133 The biological function of TMPRSS4 in these tissues is unknown. Like TMPRSS2, TMPRSS4 is able to activate ENaC when coexpressed in Xenopus oocytes40, 134, and TMPRSS4 was recently shown to cleave in the inhibitory ENaCγ subunit.135 TMPRSS4 activates influenza virus hemagglutinin, suggesting that it may facilitate viral infection in lung tissues.136
TMPRSS4 mRNA overexpression has been documented in many cancers, including pancreatic, gastric, colorectal, lung, and thyroid. 133, 137 In lung and colon cancer cells, knockdown of TMPRSS4 expression by siRNA reduced cell proliferation and invasion, whereas TMPRSS4 overexpression promoted epithelial–mesenchymal-like transition in culture and metastasis in nude mice.138, 139 The effects of TMPRSS4 in cancer cells appeared to be mediated by upregulating integrin α5 and its downstream molecules.140 These data suggest a role of TMPRSS4 in cancer progression and metastasis, although its proteolytic targets remain unknown.
6. Spinesin
Spinesin, also known as TMPRSS5, was cloned from a human spinal cord library,141 and named spinesin for spinal cord-enriched trypsin-like protease. The human TMPRSS5 gene is located on human chromosome 11q23. The spinesin protein domain structure is shown in Fig. 1. On Western blots, human spinesin appeared as a major band of approximately 52 kDa, representing its zymogen form.141 In cerebrospinal fluids, a smaller spinesin band of approximately 50 kDa was detected, suggesting that spinesin may be shed from the cell surface.142 Recombinant soluble spinesin is active toward peptide substrates with an optimal pH of approximately 10.142 The functions of spinesin are unknown, although its predominant expression in the brain and spinal cord may suggest that its primary function will be in the central nervous system. More recently, spinesin mRNA was detected in rat inner ear tissues, indicating a possible role in the auditory system.131
7. MSPL
Mosaic serine protease long-form (MSPL), also known as TMPRSS13, was isolated from a human lung cDNA library.143 The human TMPRSS13 gene has 12 exons and is located on chromosome 11q23.2. Several alternatively spliced forms from this gene have been identified.34 MSPL mRNA is expressed in the lung, placenta, pancreas, and prostate, and encodes a protease of 581 amino acids (Fig. 1). The recombinant human MSPL migrated at approximately 60 kDa and prefers synthetic peptide substrates with Arg at the P1 position. MSPL activity is inhibited by aprotinin, benzamidine, and soybean trypsin inhibitor.34 The physiological functions of MSPL remain unknown. Similar to other TTSPs, MSPL was recently shown to induce avian influenza virus replication by activating the viral hemagglutinin, suggesting a potential role in human airway viral infections.144
B. Matriptase Subfamily
The matriptase subfamily consists of the three highly homologous proteases: matriptase, matriptase-2, and matriptase-3, and a protein with an atypical mosaic structure, polyserase-1 (Fig. 1). All matriptases exhibit a similar stem region with one SEA, two CUB, and three (matriptase-2 and matriptase-3) or four (matriptase) LDLRA domains. In polyserase-1, the transmembrane domain is followed by a single LDLRA domain and a tandem repeat of three serine protease catalytic domains, referred to as serase-1, -2, and -3. Although structurally similar, the members of this TTSP subfamily appear to have quite divergent biological functions.
1. Matriptase
In 1993, Matriptase (also known as MT-SP1, TADG-15, CAP3, epithin, and ST14) was identified as a new gelatinolytic activity in conditioned medium from cultured breast cancer cells.145 It was molecularly cloned by several groups at the turn of the millennium.53, 146, 147 The matriptase gene, ST14, is located on human chromosome 11q24-25 and encodes a polypeptide of 855 amino acids. Orthologs of matriptase are present in all vertebrate genomes examined to date, indicating conserved evolutionary functions.3 Matriptase is the most widely expressed member of the matriptase subfamily and is found in the epithelial compartments of most embryonic and adult tissues.148, 149, 150, 151, 152, 153 A number of studies in different laboratories have revealed insights into the catalytic properties and unique physiological functions of matriptase.
Matriptase is an 80- to 90-kDa cell surface glycoprotein, with the modular structure illustrated in Fig. 1. Matriptase is synthesized as an inactive, single-chain zymogen and its activation is extraordinarily complex (reviewed in44). Matriptase activation requires two sequential endoproteolytic cleavages, the first in the amino-terminal SEA domain and the second within the highly conserved activation cleavage site R↓VVGG, in the serine protease domain. Proteolytic autoactivation of matriptase appears to be controlled by the stem region, posttranslational modifications, and the cellular localization of the protease44; however, the specific mechanisms that trigger the activation of matriptase are incompletely understood.154 The inhibition of activated matriptase by HAI-1 was first documented by the identification of matriptase/HAI-1 complexes in human milk, in conditioned medium of cultured mammary epithelial cells, and in a number of cancer cell lines.50
Matriptase is widely expressed in various epithelial cells during mouse development. Matriptase can first be detected on embryonic day (E)10 in the epithelial lining of several tissues of the embryo proper, such as the forming olfactory placode, oral cavity and foregut, intestine, inner ear, and apical ectodermal regions of the limbs.149 However, matriptase may be expressed even earlier in development, possibly already at the pre- or peri-implantation stage.155 At E14.5 and E16.5, respectively, matriptase becomes expressed in the developing hair follicles and the interfollicular epidermis.148 Starting on E8.5, matriptase is also present in chorionic trophoblasts of the mouse placenta, and a similar pattern of expression is also observed in the human placenta.149, 151 During postnatal life, the highest levels of matriptase expression are found in the epithelium of the gastrointestinal tract, matrix cells of the hair follicles, and the distal and collecting tubules of the kidney, while lower levels of matriptase are present in the granular/transitional layer of the epidermis and in the epithelial compartments of developing teeth, vomeronasal cavity, trachea, bronchioles, thymus, inner ear, gall bladder, urinary bladder, ureter, prostate, seminal vesicle, epididymis, uterus, and oviduct.148, 152, 153 In addition to epithelial tissues, matriptase is also expressed by certain leukocyte populations in humans, including monocytoid cells and mast cells.152, 156, 157
Matriptase has emerged to play a critical role in skin formation, in epidermal differentiation and skin function (Fig. 2 ). Initial analyses of matriptase null mice uncovered a critical function for matriptase in the development of epidermal tissues.158, 159 Matriptase-ablated mice die shortly after birth, due to a severe dehydration that results from an impaired epidermal barrier function. Follicular structures are also affected by matriptase loss, as shown by the absence of whiskers and generalized hypoplasia of pelage hair follicles of null mice. These phenotypes were linked to defects in the initiation of caspase-14-calpain I, bleomycin hydrolase-mediated processing of the epidermal polyprotein, profilaggrin, into filaggrin monomer units and subsequently, into free hygroscopic amino acids, which partake in the formation of the cornified envelope of the uppermost layers of the epidermis and serve as a source of water binding free amino acids that contribute to skin hydration.160 Matriptase-deficient epidermis also exhibits a defect in the formation of lamellar granules, specialized secretory vesicles that contain lipid material required for the formation of extracellular lipid lamellae within the cornified layer, and display impaired formation of epidermal tight junctions within the granular layer.158, 161

Matriptase is critical for the development and functions of the epidermis. Matriptase activation, triggered by unknown mechanisms in skin, activates the GPI-anchored prostasin zymogen. Prostasin activation is required for epidermal tight junction formation, epidermal lipid synthesis, and induction of caspase-14-calpain I, and bleomysin hydrolase-mediated processing of the epidermal polyprotein, profilaggrin, into filaggrin monomer units, and subsequently, into free hygroscopic amino acids, which participate in the formation of the cornified envelope and contribute to skin hydration. Matriptase deficiency is linked to a rare form of skin disease, referred to as autosomal recessive ichthyosis with hypotrichosis (ARIH), or ichthyosis, follicular atrophoderma, and hypotrichosis (IFAH). Matriptase is also capable of activating proinflammatory pro-kallikrein-related peptidases that are associated with stratum corneum detachment and are responsible for the runaway kallikrein proteolytic cascade associated with LEKTI-deficiency/Netherton syndrome. HAI-1 regulates matriptase in the epidermis.
More recent studies suggest that matriptase is part of an epidermal proteolytic cascade and enables epidermal differentiation through the activation of the GPI-anchored membrane serine protease, prostasin. This is evidenced in part by the identical phenotype of matriptase- and prostasin-deficient mouse skin, and by the absence of active prostasin in matriptase-deficient epidermis.150, 162 Matriptase and prostasin are found together in a variety of other simple, stratified, and pseudo-stratified epithelia,163 suggesting that matriptase may be a candidate activator of the prostasin zymogen in additional physiological settings.
Several recent reports have linked a rare form of skin disease, referred to, respectively, as autosomal recessive ichthyosis with hypotrichosis (ARIH) or ichthyosis, follicular atrophoderma, and hypotrichosis (IFAH), to homozygosity or compound heterozygosity for an assortment of mutations in the human ST14 gene, encoding matriptase.164, 165, 166 ARIH/IFAH patients present with mild to moderate ichthyosis, indicative of impaired barrier function, and hair follicle hypoplasia associated with fragile, brittle, dry, and slow-growing scalp hair. All these symptoms resemble transplanted skin from matriptase null mice or skin from matriptase hypomorphic mice.158, 167 Importantly, prostasin activation is impaired in ARIH/IFAH epidermis,165 further supporting the belief that activation of the prostasin zymogen may be a principal function for matriptase during epidermal differentiation.
Matriptase is also linked to the initiation of the Netherton syndrome in a LEKTI-deficient mouse model through the premature activation of an epidermal pro-kallikrein protease cascade.168 Deficiency in the serine protease inhibitor, LEKTI, is the etiological origin of the Netherton syndrome, and causes detachment of the stratum corneum and chronic inflammation due to excessive kallikrein-related protease activity. Matriptase was demonstrated to initiate activation of proinflammatory pro-kallikrein-related peptidases that are associated with stratum corneum detachment. Moreover, ablation of matriptase from LEKTI-deficient mice dampened inflammation, eliminated aberrant protease activity, prevented detachment of the stratum corneum, and improved the barrier function of the epidermis, demonstrating that the runaway kallikrein proteolytic cascade associated with LEKTI-deficiency was dependent on matriptase activity.
The wide expression of matriptase in epithelial tissues, including simple epithelia of most organs, suggested that matriptase could have functions in epithelial biology beyond the epidermis, but attempts to investigate this notion were initially hampered by the perinatal lethality of matriptase null mice. The subsequent generation of viable matriptase hypomorphic mice167 and matriptase conditional knockout mice161 afforded the opportunity to explore the function of matriptase in global epithelial biology. Tissue-specific embryonic and postnatal ablation of matriptase from a variety of epithelial tissues, including orofacial epithelium, salivary gland epithelium, lacrimal gland epithelium, and the epithelium of stomach, and small and large intestine, in all cases were associated with severe epithelial dysfunction, with two distinct phenotypes emerging: (a) loss of epithelial function, such as barrier and secretory capacity, but preservation of epithelial anatomy (salivary glands, tear glands, stomach, small intestine) and (b) loss of epithelial function, followed by loss of anatomical integrity (skin, orofacial, and colonic epithelium). In all epithelia, the absence of matriptase uniformly was associated with the rapid loss of epithelial tight junction function, compatible with the localization of matriptase on the lateral membrane of polarized epithelial cells.161, 169 In polarized monolayers of epithelial cells cultured ex vivo, downregulation of matriptase decreased transepithelial electrical resistance and increased paracellular diffusion of macromolecules. Decreased matriptase was associated with enhanced expression and incorporation of the permeability-associated, “leaky” tight junction protein, claudin-2, at intercellular junctions, suggesting that the reduced barrier integrity was caused, at least in part, by an inability to regulate claudin-2 expression and incorporation into tight junctions.169
Matriptase is nearly ubiquitously coexpressed with the Kunitz-type transmembrane serine protease inhibitors, HAI-1 and HAI-2, in adult and embryonic tissues56, 149, 151, 155, 170, 171, 172, and both HAI-1 and HAI-2 display potent matriptase inhibitory activity in purified systems.36, 56, 170, 173, 174 Knockout studies have revealed that HAI-1 and HAI-2 are both required for the completion of embryonic development in mice.149, 151, 155, 175 However, both HAI-1/matriptase double-deficient and HAI-2/matriptase double-deficient mice complete embryonic development, thereby revealing matriptase as a principal inhibitory target for both protease inhibitors.149, 155 Loss of HAI-1 inhibition of matriptase in mice leads to failure of the chorionic epithelium to differentiate into the placental labyrinth, which prevents embryonic development beyond mid-gestation,149 whereas other developmental processes proceed normally. HAI-2 inhibition of matriptase, however, is essential at three distinct developmental processes: (a) early (< E8.5) embryonic development, which proceeds normally in HAI-2 null embryos in the absence, but not in the presence of matriptase; (b) placental development, where the failure of the placental labyrinth to undergo branching morphogenesis in HAI-2 null embryos can be prevented by loss of matriptase; and (c) neural tube closure, in which the development of exencephaly and spina bifida is partially rescued by the matriptase deficiency.155 The functional interactions between matriptase, HAI-1, and HAI-2 during development appear to be uniquely complex for a protease–protease inhibitor system. Thus, not only the complete loss of HAI-1 or HAI-2 but also the combined haploinsufficiency for both inhibitors causes embryonic lethality (nonallelic noncomplementation), which can be prevented by haploinsufficiency for matriptase, suggesting that the two inhibitors functionally cooperate in regulating the activity of matriptase.155
The requirement for matriptase regulation by HAI-1 is not restricted to development. Thus, the loss of HAI-1 from adult tissues is associated with fatal epithelial dysfunction in mice,176 but HAI-1-deficient mice in which the level of matriptase is genetically reduced by 85–99% are fertile, healthy, have normal long-term survival, and possess histologically unremarkable epithelia. Similar findings were obtained from studies in zebrafish, where the combined loss of function of the two HAI-1 encoding genes compromises the integrity of embryonic epidermal keratinocytes and causes skin inflammation and embryonic death 18–26 h postfertilization. All of these defects can be fully rescued by a simultaneous downregulation of matriptase.177
Matriptase has received significant attention in the field of cancer biology due to its remarkably consistent expression in tumors of epithelial origin. Following the first description of matriptase as a major gelatinolytic activity in breast carcinoma,145, 178 the protease was reported to be expressed in a wide variety of other benign and malignant tumors of epithelial origin, including prostate, ovarian, cervical, gastric, colon, renal cell, esophageal, oral squamous cell carcinoma (SCC), and malignant pleural mesothelioma (reviewed in Ref. 179). In most carcinomas, malignant progression is associated with a significant increase in matriptase mRNA or protein expression. For example, in ductal mammary carcinoma, elevated levels of matriptase correlate with both tumor and nodal staging.180 A clinical study in node-negative breast cancer patients showed a tight correlation between the expression of matriptase, cMet, and HAI-1, and poor patient outcome.181 Likewise, more than fivefold overexpression of matriptase was detected in a study of ovarian cancer, compared to normal ovarian tissues, and this increased expression of matriptase correlated with tumor aggressiveness.182, 183 Furthermore, although matriptase was more frequently expressed in stage I/II tumors than in more advanced-stage III/IV tumors, an increased matriptase/HAI-1 ratio was indicative of the poor clinical outcome of advanced-stage tumors, suggesting that loss of protease inhibition may play a role in the late stages of the disease.184 In prostate and cervical carcinoma, a several-fold increase in matriptase expression was reported for malignant versus nonmalignant tissues, and this correlated with Gleason score and histopathological grade, respectively, of the tumors.185, 186 Other common human cancers with significantly elevated matriptase levels include carcinomas of the pancreas, lung, kidney, and liver.179 In contrast, significant downregulation of matriptase and HAI-1 has been detected in the samples of gastric and colorectal carcinoma.172 However, an analysis of the clinicopathological parameters in colorectal adenoma and carcinoma tumors showed an increased matriptase/HAI-1 ratio in the tumors compared to the corresponding tissue from control individuals.187 This finding may suggest that in tissues of the gastrointestinal tract, which exhibit high expression of matriptase and HAI-1,152, 188, 189 the tumorigenic process does not involve further elevation in the expression of the two proteins, but rather may be associated with altered levels of an HAI-1-free, uninhibited form of matriptase. SCC of the skin represents a second example of matriptase being dysregulated, not by overexpression, but rather by the translocation of the expression of the protease from a differentiated postmitotic compartment in the epidermis to a basal compartment with high tumorigenic potential.148
Matriptase is unique among pericellular proteases in that it possesses a strong oncogenic potential when misexpressed in an epithelial tissue.173 Even low-level matriptase expression in basal keratinocytes of transgenic mice suffices to induce severe hyperproliferation of epidermal keratinocytes, which gradually progresses to invasive SCC. Both the epidermal hyperproliferation and the formation of matriptase-induced skin tumors are completely abolished by coexpression of HAI-1, demonstrating that the proteolytic activity of matriptase is critical to its oncogenic potential. The molecular mechanisms of matriptase-induced epithelial carcinogenesis remain to be determined. In cell-free or cell-based assays, matriptase can activate several proteins that have been previously associated with malignant progression, including pro-hepatocyte growth factor/scatter factor (pro-HGF),29, 190 pro-macrophage stimulating protein-1 (pro-MSP-1),191 pro-urokinase plasminogen activator (pro-uPA),29, 190 protease activated receptor-2 (PAR2),29, 192 and the src-associated transmembrane protein SIMA135/CDCP1.193, 194
2. Matriptase-2
Matriptase-2, also called TMPRSS6, was independently cloned from liver tissues by two groups in 2002 and 2003 and shown to express a membrane serine protease with homology to matriptase that displayed proteolytic activity toward various macromolecular substrates.37, 195 Matriptase-2 is encoded by the TMPRSS6 gene located on human chromosome 22q12.3. Matriptase-2 is approximately 90-kDa cell surface glycoprotein with a modular structure (Fig. 1) and is synthesized as an inactive, single-chain zymogen. Cell surface matriptase-2 is efficiently shed into the conditioned medium of transfected cells in an active two-chain form by proteolytic cleavage within the second CUB domain of the noncatalytic stem region.196 Whereas matriptase is expressed in a large number of embryonic and adult epithelia, matriptase-2 expression is largely confined to adult and fetal liver in humans and mice, with minor expression in the kidney, uterus, and nasal cavity.37, 195
A breakthrough in the understanding of the physiological function of matriptase-2 was enabled by the generation of matriptase-2 knockout mice and by the identification of loss of function mutations in the TMPRSS6 gene as a cause of the human autosomal recessive disorder, iron-refractory iron deficiency anemia (IRIDA). Both mice and humans with matriptase-2 deficiency suffer from very low iron levels and severe microcytic anemia.197, 198, 199 Matriptase-2 expressed by liver cells functions as a suppressor of the hepatic hormone, hepcidin, which in turn internalizes the iron export protein, ferroportin, on enterocytes and macrophages to reduce iron uptake. Thus, matriptase-2 is a key regulator of systemic iron hemostasis. Hepcidin suppression by matriptase-2 appears to occur at the transcriptional level, as hepcidin mRNA levels are elevated in both matriptase-2-deficient humans and mice.197, 198, 199 This suppression of hepcidin gene transcription has been linked to matriptase-2-mediated degradation of hemojuvelin, a cofactor for bone morphogenetic protein, and a key regulator of hepcidin gene activation.200, 201
3. Matriptase-3
Matriptase-3 was identified by bioinformatic analysis in 2005 as a protease with high homology to matriptase.202 The TMPRSS7 gene encoding matriptase-3 is located on human chromosome 3q13.2 and encodes an approximately 90-kDa glycosylated protein detected on the cell surface.202 Orthologs of the matriptase-3 gene are present in all vertebrates analysed to date, including fish, birds, rodents, canines, and primates. The pattern of expression of matriptase-3 is conserved in mice and humans, with the highest levels of mRNA expression in the brain, skin, eye, salivary gland, and the reproductive tissues, including prostate, testis, epididymis, ovary, and uterus. The recombinant matriptase-3 catalytic domain can hydrolyse synthetic peptide substrates with a strong preference for arginine in the P1 position, and shows proteolytic activity toward several macromolecular substrates, including gelatin, casein, and albumin.202 The activated matriptase-3 catalytic domain forms stable inhibitor complexes with an array of serpins, including PAI-1, PCI, α1-proteinase inhibitor, α2-antiplasmin, and antithrombin III in vitro. Matriptase-3 loss of function studies in animals or humans with matriptase-3 deficiency has not been reported, and the physiological function of the protease remains to be determined.4
4. Polyserase-1/Serase1B
Polyserase was cloned from a human liver cDNA as a unique type II transmembrane serine protease with three serine protease domains, two of which display catalytic activity.203 mRNA capable of encoding full-length human polyserase-1 is detected predominantly in the skeletal muscle, heart, kidney, liver, placenta, and the brain. In addition, a shorter splice variant, termed serase-1B, which contains only the first of the three serine protease domains of polyserase-1, has been described in mice and humans, with its highest expression detected in the liver, small intestine, pancreas, testes, and the peripheral blood CD14+ and CD8+ cells.35, 203 Recombinant serase-1B shows proteolytic activity toward synthetic peptide substrates, converted pro-uPA into active uPA, and could be inhibited by the serpins, aprotinin, α2-antiplasmin, and PAI-1.35
The human polyserase gene, called TMPRSS9, is located on human chromosome 19p13.3. The 5′ promoter region of the mouse polyserase-1 gene has been characterized and contains a GATA motif, a glucocorticoid responsible element, and an E-box sequence required for maximal promoter activity.204 The physiological function of polyserase has not been elucidated.
C. Corin Subfamily
Corin, the single member of the corin subfamily, is characterized by a complex stem region composed of two frizzled domains, eight LDLRA domains, and one Group A SR domain (Fig. 1).
1. Corin
Human corin cDNA was originally cloned from the heart. In a search for novel serine proteases in the cardiovascular system, Yan et al. identified a partially expressed sequence in a genomic database and subsequently cloned the full-length cDNA encoding corin.205 The protease was named corin for its abundant cardiac expression. Independently, Hooper et al. isolated human corin cDNA from HeLa-derived cells that were resistant to tumor necrosis factor-α-induced apoptosis.206 Corin was also cloned from a mouse heart library as a novel protein containing LDL receptor-like repeats, and was named LRP4 (LDL receptor-related protein 4).207
The human CORIN gene is present on chromosome 4p12-13, consists of 22 exons, and spans approximately 200 kb.208 Corin is highly expressed in fetal and adult cardiomyocytes. Low levels of corin mRNA are detected in the kidney, bone, brain, skin, and pregnant uterus.109 In both human and mouse corin genes, there is a 5′-flanking region that contains conserved binding sites for TBX5, GATA, NKX2.5, NF-AT, and Kruppel-like transcription factors.208 Among them, a conserved GATA-binding site is critical for interacting with GATA-4 transcription factor that is important for heart-specific gene expression. GATA-4 protein is known to act downstream of the calcineurin/NF-ATc3 signaling pathway in cardiomyocytes. Most likely, this signaling pathway is involved in regulating corin expression in the heart.
The full-length human corin cDNA encodes a polypeptide of 1042 amino acids with a calculated molecular mass of approximately 116 kDa and a domain structure shown in Fig. 1. The presence of two frizzled-like cysteine-rich domains in the corin extracellular region is unique among the TTSPs.4 Native and recombinant human, rat, and mouse corin proteins appear as bands of approximately 150–200 kDa on immunoblots, largely due to N-glycosylation which has been confirmed by glycosidase digestion.99 Human corin has 19 predicted N-linked glycosylation sites in its extracellular region, whereas rat and mouse corin has 17 N-glycosylation sites. Corin does not contain detectable amounts of O-glycans or sialic acids.209, 210
Corin is synthesized as a zymogen with no detectable activity in functional assays, indicating that cleavage at Arg801 within the conserved activation site, R↓ILGG is necessary for its activity.211 A small fraction of activated corin is detected in transfected HEK 293 cells and cardiomyocytes210; however, the enzyme(s) responsible for corin activation in these cells have not been determined. Recombinant corin with an engineered activation site has a trypsin-like catalytic activity, favoring peptide substrates with Arg/Lys residues at P1, Pro/Phe/Gly at P2, and small neutral amino acids at the P3 position.211 The activity is inhibited by leupeptin, aprotinin, and soybean trypsin inhibitor and, unlike many serine proteases, corin activity is not inhibited in the presence of human plasma.211
The topology of corin on the cell membrane has been confirmed by cell surface protein labeling and protease digestion.99, 209 In addition to the transmembrane domain, N-glycans on corin also play a role in its cell surface targeting and activation.210, 212 Inhibition of N-glycosylation or removal of certain N-glycosylation sites by site-directed mutagenesis impaired corin cell surface expression and zymogen activation. The transmembrane domain is not necessary for corin enzyme activity, since soluble corin without the transmembrane domain exhibited similar activities toward small peptide and biological substrates when compared to that of wild-type corin.211
Wu et al. identified corin as the physiological pro-atrial natriuretic peptide (pro-ANP) convertase.213 ANP, also called atrial natriuretic factor (ANF), is a cardiac hormone that regulates blood pressure and cardiac function by promoting natriuresis, diuresis, and vasodilation. The function of ANP is mediated by its receptor, NPR-A, which promotes intracellular cGMP production. In mice, deficiency in ANP or its receptor causes spontaneous hypertension, demonstrating the importance of the ANP pathway in regulating blood pressure.213 In humans, SNPs and mutations in the NPPA gene encoding ANP have been reported in patients with hypertension and heart disease.214
In cardiomyocytes, ANP is made as a precursor molecule, pro-ANP. Upon secretion under high blood volume or pressure, pro-ANP is converted to active ANP by proteolytic cleavage. This activation mechanism was recognized for many years, but the responsible enzyme remained unidentified. In cell-based studies, corin activated pro-ANP in a sequence-specific manner.215 This activity was confirmed in cardiomyocytes and with purified recombinant corin. Chan et al. made corin null mice, which were viable and fertile.216 In these mice, lack of corin prevents pro-ANP processing in the heart and causes salt-sensitive hypertension. Corin null mice develop cardiac hypertrophy and exhibit impaired cardiac function. This hypertrophic heart phenotype has been confirmed in a naturally occurring mutant mouse strain, Kit W-sh, in which the corin gene is disrupted by genetic inversion.217 These data show that corin-mediated pro-ANP processing is critical for regulating blood pressure and cardiac function (Fig. 3 ). Curiously, corin null mice appear to have a lighter coat color, and this phenotype depends on the agouti gene.218 As corin mRNA and protein are expressed in the dermal papilla in mice, corin may act in the skin to regulate hair color formation by an agouti pathway-dependent mechanism.
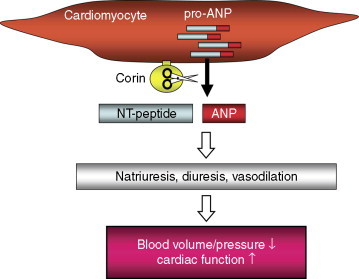
Corin-mediated pro-ANP processing in the heart. In cardiomyocytes, ANP is made as a precursor, pro-ANP. Upon secretion, pro-ANP is cleaved by corin, generating an inactive N-terminal (NT) peptide and an active ANP. ANP promotes natriuresis, diuresis, and vasodilation, thereby reducing blood volume/pressure and improving cardiac function.
In addition to ANP, BNP (B-type natriuretic peptide) and CNP (C-type natriuretic peptide) are additional members of the human natriuretic peptide family, which share similar protein sequences and structures. The function of BNP is to promote natriuresis and diuresis, whereas CNP is involved in endothelial cell growth and chondrocyte differentiation. In cell-based studies, corin also cleaved pro-BNP, although the reaction was less efficient than that for pro-ANP.215 Recent studies indicate that both furin and corin convert pro-BNP to BNP.219 In contrast, pro-CNP is processed by furin but not corin.220 It is interesting that these natriuretic peptides have evolved from an ancestor gene, but their activation processing requires different enzymes.
Corin variants exist in hypertensive patients. Dries et al. have identified two SNPs (T555I/Q568P) in the human CORIN gene.221 These SNPs are located on a minor corin gene allele that is present primarily in African-Americans with hypertension. It is known that African-Americans have a high prevalence of cardiovascular disease, but the underlying mechanisms remain unclear. Rame et al. have shown that individuals with these corin SNPs have an enhanced cardiac response to high blood pressure, which leads to severe left ventricular hypertrophy.222 Under pathological conditions, cardiac hypertrophy impairs heart functions. In patients with heart failure, the presence of this corin variant allele is associated with worse clinical outcomes such as hospitalization and death.223 Changes in the amino acids, T555I/Q568P, encoded by the SNPs occur within the second frizzled-like domain, an extracellular region demonstrated to be functionally important.46 Examination of the effect of these amino acid substitutions on corin function in cell-based studies showed that the corin variant, T555I/Q568P, exhibited markedly reduced activity in processing pro-ANP and pro-BNP.224 Apparently, the reduced activity was caused by impaired corin zymogen activation but not cell surface expression.224 The data indicate that these polymorphisms alter corin structure and function, which may contribute to elevated blood pressure and cardiac hypertrophy in patients. Thus, corin defects may present an important mechanism underlying cardiovascular disease.
Soluble corin has been detected in human plasma, suggesting that corin is shed from the cell surface and subsequently circulates in the blood.225 Interestingly, plasma corin levels were significantly lower in patients with heart failure and the reduction correlated with the disease severity.226 In contrast, patients with acute myocardial infarction had similar levels of plasma corin compared to that of normal controls, suggesting that low plasma corin levels are associated more closely with pathological changes in heart failure than that in acute ischemic cardiac injury. The protease(s) responsible for corin shedding have not been determined. Low levels of plasma soluble corin in patients with heart failure may reflect reduced corin production, accelerated clearance of plasma corin, and/or downregulation of corin shedding in failing hearts. Additional studies will be required to elucidate corin shedding mechanisms under physiological and pathological conditions, and whether decreased plasma corin could be used as a biomarker for the diagnosis of heart failure.
D. HAT/DESC Subfamily
The HAT/DESC (human airway trypsin-like protease/differentially expressed in squamous cell carcinoma) subfamily comprises five members in humans, HAT, DESC1, TMPRSS11A, HAT-like 4/TMPRSS11F, and HAT-like 5, and two additional members in rodents, HAT-like 2/DESC4 and HAT-like 3.3, 4 All genes encoding members of this subfamily are located within a single gene cluster on chromosome 4q in humans (5 E1 in mice), suggesting that they originated by gene duplication from a common ancestor.227 Of all TTSPs, members of the HAT/DESC subfamily exhibit the simplest modular structure of the stem region, which consists of a single SEA domain located adjacent to the cell surface. SEA domains are structurally homologous protein modules that have been identified in many mucin-like proteins. These domains undergo spontaneous posttranslational confirmation-driven hydrolysis of the glycine–serine peptide bond within a conserved GSVVV sequence, although the two peptides remain tightly associated.228 Little is known about most of the members of the HAT/DESC subfamily, with HAT, DESC1, and TMPRSS11A being the best characterized.
1. HAT
HAT was originally characterized as a novel 28-kDa protease from the sputum of patients with chronic airway diseases.229 The cDNA was subsequently cloned and the protease was named HAT for human airway trypsin-like protease.230 HAT cDNA encodes an integral membrane protein of 418 amino acids, with a predicted molecular mass of approximately 46-kDa, which is released in a soluble form from the cell surface.230 Hence, significant HAT-related activity can be detected in the extracellular airway fluids of patients with chronic airway inflammatory diseases, particularly in patients with asthma.229, 231, 232 Within human tissues, the airways and the skin have been shown to express HAT.233, 234 In the airways, HAT is expressed in the apical cilia layer of tracheobronchial epithelial cells.234, 235
There is little information regarding the physiological functions and candidate proteolytic targets of HAT; however, HAT upregulation is found to be associated with inflammatory environments.233 The available evidence, obtained from the study of in vitro cleavage reactions and cell-based assays, supports a potential role for HAT in respiratory homeostasis during inflammatory responses through a capacity to increase mucin gene expression, control fibrin deposition, and stimulate bronchial fibroblast proliferation in airway epithelial cells in vitro. 231, 235, 236 HAT has been demonstrated to have the capacity to degrade fibrinogen, to activate pro-uPA,232, 235 to activate membrane receptors such as PAR-2,232, 235, 236, and to cleave the D1–D2 linker sequence of human uPAR (h-uPAR), which prevents interaction with the extracellular matrix component, vitronectin.237 The physiological relevance of these cleavages, if any, remains untested.
HAT is one of the several TTSPs found to cleave the surface glycoprotein hemaglutinin (HA) of the influenza virus.118, 238 HA is responsible for initiating influenza virus replication by mediating binding to sialic acid-containing cell surface receptors and fusion of the viral envelope with the endosomal membrane. HA precursor proteins require cleavage at a single arginine amino acid to trigger membrane fusion. Membrane-bound HAT was shown to cleave newly synthesized HA before or during the release of progeny virions as well as HA of incoming viruses prior to endocytosis at the cell surface, supporting its contribution to the facilitation of virus activation and spread.
2. DESC1
Differentially expressed in squamous cell carcinoma (DESC1) was originally identified by representational difference comparative analysis of RNA from SCC of the head and neck compared with matched normal tissue.239 DESC1 is expressed in normal epithelial cells of prostate, skin, testes, head, and neck, whereas it is downregulated in 11/12 SCCs,239 suggesting that it may serve as a tumor marker. The human DESC1 gene encodes a polypeptide of 442 amino acids. The mouse ortholog of DESC1 shares 72% identity with human DESC1.227 Both proteases are expressed in similar anatomical locations and are predicted to have common functions in the development and maintenance of oral epidermal tissues and the male reproductive tract.227
The physiological functions of DESC1 are not known. DESC1 is upregulated during the induction of terminal keratinocyte differentiation.240 Madin-Darby canine kidney (MDCK) cells expressing exogenous human DESC1 display enhanced motility and an increase of tubular forms in a three-dimensional collagen lattice following HGF treatment.241 Kinetic studies using internally quenched peptides227, 242 and structural analyses of the DESC1 catalytic domain23 reveal DESC1 substrate preference for large hydrophobic residues in P4/P3, for small residues in P2, Arg or Lys in P1, and hydrophobic residues in P1′ and P3′. Mouse DESC1 forms stable inhibitory complexes with PAI-1 and PCI, suggesting that these serpins might be regulators of DESC1 proteolytic activities in DESC1 expressing tissues.227
3. TMPRSS11A
Most of the remaining members of the HAT/DESC family have not yet been well characterized. HATL1, also known as TMPRSS11A, is expressed in the upper respiratory tract (pharynx and trachea) and has been shown to have the capability to cleave recombinant, native, full-length S-protein trimer (triSpike) of the severe acute respiratory syndrome, coronavirus (SARS-CoV) in vitro. 243
VI. The GPI-Anchored Serine Proteases
A. Prostasin
Prostasin, also known as PRSS8 and channel-activating protease (CAP)-1, was originally identified and characterized from human seminal fluid.11 Subsequent studies using a Xenopus oocyte expression system and mammalian epithelial cell cultures independently showed that prostasin expression increased amiloride-sensitive ENaC current.134, 244, 245, 246 As a result, prostasin became the first membrane-anchored serine protease to be implicated in the modulation of fluid and electrolyte regulation via proteolysis of ENaCs.
The PRSS8 gene encoding prostasin belongs to the family of genes located on the syntenic regions of human chromosome 16p13.3 and mouse chromosome 1714, 247, 248, 249, and this family includes genes encoding both testisin and tryptase γ1.9 The mouse frizzy (fr) and rat “hairless” (fr(CR)) mutations are natural variants of the murine prostasin gene (Prss8). The deduced amino acid sequence of PRSS8 predicts a preproenzyme consisting of 343 amino acids, which shows 34–42% identity to human acrosin, plasma kallikrein, and hepsin. Prostasin is posttranscriptionally modified with GPI-anchor11, 12, 13 and associates with lipid rafts.13 In polarized epithelia, prostasin localizes to apical membranes.12, 250, 251 Soluble forms of prostasin are found in human urine252, 253 and are elevated in hypertensive patients.254 Prostasin has been found to be released from the cell surface by an endogenous GPI-specific phospholipase D113 or, alternatively, may be shed via a tryptic-like proteolytic cleavage in its hydrophobic C-terminal domain.11, 255
Tertiary structures of prostasin24, 25, 26 and analyses for substrate preference using positional scanning combinatorial substrate libraries32 have revealed insights into prostasin's unique catalytic activity. Prostasin was found to have a substrate preference for polybasic substrates: in position P4, preference was for arginine or lysine; in P3, for histidine, lysine, or arginine; in P2, for basic or large hydrophobic amino acids; and in P1, for arginine and lysine.32 Prostasin showed no activity with substrates containing isoleucine in position P1′, providing an explanation for the inability of prostasin to autoactivate. Prostasin activity was also highly influenced by mono- and divalent metal ions, which were potent inhibitors and substrate specific modulators of enzymatic activity. Interestingly, structural analyses showed that the S1 subsite loop of prostasin exhibits a large degree of conformational variation and directly binds the divalent cation, Ca2+, being able to move to block or to expose the S1 subsite.24
The prostasin zymogen is proteolytically cleaved to an active enzyme by matriptase in vitro and in vivo in skin150 and when coexpressed with hepsin in cultured cells.96 Low levels of plasmin found in urine from patients with nephritic syndrome may also activate prostasin in the context of ENaC activation.256 Intriguingly, the zymogen form of matriptase was also able to be converted to an active protease by the addition of active prostasin, suggesting that, in certain cellular contexts, prostasin might function both upstream and downstream of matriptase.192 Comparison of the skin phenotypes of conditional prostasin-deficient mice with matriptase-deficient mice supports the participation of both serine proteases in the same protease-signaling cascade in the skin.162 Antithrombin III and protease nexin I are inhibitors of cell surface-associated activities of prostasin257, and protease nexin I is an effective inhibitor of prostasin-mediated activation of ENaC.257 Prostasin is also inhibited by the Kunitz-type inhibitors, HAI-1/SPINT131, 48, 57, 58 and HAI-2/SPINT2,32, 258 similar to matriptase.
The data suggest that the “open probability” of ENaCs is increased by prostasin, resulting in an increased cellular uptake of sodium (Na+) which can, in turn, regulate the homeostasis of extracellular fluid volume, blood pressure, and Na+ reabsorption.259 Prostasin cleaves the ENaCγ subunit in the extracellular loop at a site (K186) distal to a furin cleavage site, and releases a 43-amino acid inhibitory peptide that results in increased open probability of the channel and full activation.260, 261 Recent studies have localized this inhibitory peptide to a key 11-mer tract, R158-F168 (RFLNLIPLLVF), which is capable of inhibiting wild-type ENaC expressed in Xenopus oocytes and endogenous channels in airway epithelial cells.262 The channel-activating activity of prostasin is likely to be of pathophysiological significance. In the lung, adequate levels of ENaC activity are essential for controlling airway surface liquid volumes. Mice with targeted disruption of the prostasin gene in lung epithelium show impaired ENaC-mediated alveolar fluid clearance.263 In addition, increased ENaC activation in the airway of cystic fibrosis patients has been linked to excessive prostasin activity on the surface of lung epithelium.264, 265 Prostasin has been identified as a candidate gene for the development of hypertension in youths,266 which may also be associated with increased ENaC activation.267 Increased levels of urinary prostasin are found in hypertensive patients,252 and inhibition of prostasin in a rat model of hypertension significantly reduced blood pressure.268 Inhibition of prostasin-dependent activation of ENaCs is considered a potential therapeutic strategy for modulating surface liquid volume in cystic fibrosis and salt-sensitive hypertension.
Prostasin also exhibits pleiotropic activities associated with the activation of growth factors, G protein-coupled receptors, and the activation of proteolytic cascades.269, 270, 271 Less well-characterized potential targets of prostasin include PAR2 and the epidermal growth factor receptor.269 Soluble recombinant prostasin has also been reported to activate PAR2 indirectly, through a matriptase-dependent mechanism.192 Prostasin activity is further implicated in the regulation of epithelial barrier function,13 which is believed to be unrelated to ENaC processing activity, suggesting that prostasin may also target as yet unidentified substrates involved in the regulation of epithelial barrier integrity and permeability. Downregulation of prostasin is associated with tumor progression269, 272, 273 through as yet unknown mechanisms.
B. Testisin
Testisin was originally cloned from human eosinophils and from HeLa cervical carcinoma cells.15, 274 Human testisin has been known as eosinophil serine protease 1 (esp-1)274 and murine testisin247 has been called tryptase 4248 and TESP5.14 The testisin gene (PRSS21) belongs to the family of genes on the syntenic regions of human chromosome 16p13.3 and mouse chromosome 17.9, 14, 247, 248, 249 Several isoforms of human testisin have been identified, which are believed to be generated by alternate intron–exon junctional sliding.249, 275 Testisin is abundantly expressed by male germ cells and sperm15, 247, 276, and is also present in capillary endothelial cells277 and in eosinophils.248, 274
Analyses of the promoter sequences of the human and mouse genes encoding testisin have revealed that the region surrounding the transcription initiation site lacks a TATA consensus sequence, but contains a CCAAT sequence and includes a 385-bp 5′-CpG island.247, 249 There is a strong correlation with DNA hypermethylation of the 5′-CpG rich island and silencing of testisin gene expression.278 Furthermore, the 5′-flanking region of the testisin gene contains several consensus response elements, including Sp1, AP1, and several testis-specific elements.247, 249, 275
Testisin is posttranscriptionally modified by the addition of a carboxy-terminal GPI-membrane anchor and has been demonstrated to be compartmentalized at plasma membranes within the dynamic microenvironment of cholesterol-rich membrane microdomains or lipid rafts.14 Naturally occurring and recombinant testisin proteins are cell-associated,14, 15, 248 but may be released from cell membranes by exposure to exogenous bacterial phosphatidylinositol-specific phospholipase C (PI-PLC).14
Developmental evidence links testisin to the maturation and function of mammalian sperm. Testisin is detected on spermatogenic cells throughout spermatogenesis and on mature sperm in humans,276 mice,14, 247, 276 and rats,279 which suggests a similar function among these mammalian species. Mice deficient in testisin display several functional abnormalities associated with epididymal sperm maturation and fertilizing ability.276, 280 Epididymal sperm lacking testisin show an increased tendency toward decapitation, heterogeneity in sperm form and angulated flagella, decreased numbers of motile sperm, and abnormal sperm volume regulation. Although testisin-deficient mice exhibit normal fertility when bred by continuous mating, these functional sperm defects result in a decreased ability to fertilize oocytes in short-term mating studies.276 The data suggest an essential requirement for testisin during sperm cell maturation processes which occur during epididymal transit and are required for normal fertilizing ability. Functional overlap between testisin and the related fertilization serine protease, acrosin, may compensate for respective functional fertilizing abilities, since acrosin-deficient mice exhibit normal fertility, but acrosin/testisin double knockout mice are subfertile.281 It has been proposed that aberrant regulation of testisin may contribute to certain secondary male infertility syndromes such as “easily decapitated” spermatozoa in humans.276
Absence of testisin expression is associated with testicular tumor formation and progression15, and overexpression of testisin promotes advanced-stage disease in ovarian carcinomas.282 Epigenetic gene silencing may contribute to the downregulation of both prostasin and testisin expression in cancers.272, 278, 282, 283, 284
VII. Perspectives
Over the past few decades, membrane-anchored serine proteases have emerged to play key roles in many diverse aspects of mammalian physiology, including food digestion, fluid balance, blood pressure regulation, hearing and inner ear development, epithelial barrier function, sperm maturation, and regulation of iron homeostasis (Table I ). The associations of mutant alleles of the membrane-anchored serine proteases with disease, the misregulation of these enzymes in malignant growth, and the hijacking of their activities by viruses to facilitate infection and propagation, all highlight the pathological impact of misregulation of their activities. Despite remarkable progress in the past few years, many questions remain unanswered. How these proteases intersect and modulate the protease cascades in the pericellular microenvironment, the signals that trigger their activation, the repertoire of endogenous substrates, and the inhibitors that modulate their activities, are important issues to address. As suitable molecular tools, such as antibodies, activity-based probes, animal models, and human mutational analyses, are refined and developed, we will look forward to gaining a better understanding of the critical roles of these enzymes both in mammalian physiology and in disease.
Table I
Membrane-Anchored Serine Protease Physiological Functions in Mammalian Development and Tissue Homeostasis
Enteropeptidase | Proteolytic activation cascade in intestine |
Hepsin | Cochlear development and hearing (mice) |
TMPRSS3 | Hearing |
TMPRSS5 | Hearing |
Matriptase | Epidermal and hair follicle development and epithelial barrier functions |
Matriptase-2 | Regulates iron hemostasis; deficiency or mutation increases hepcidin levels, leading to iron-refractory iron deficiency anemia (IRIDA) |
Corin | Processes critical cardiac pronatriatic peptides involved in the regulation of blood pressure |
Prostasin | Epidermal and hair follicle development and epithelial barrier functions; regulation of ENaC-mediated alveolar sodium and water transport (mice) |
Testisin | Subfertility and aberrant sperm morphology (mice) |
Acknowledgments
This work was supported by grants from the National Institutes of Health (CA098369, HL084387, DK081376) (T. M. A.), (HL089298 and HL089298-S1) (Q. W.), the NIH Intramural Program (T. H. B.), and the Mary Kay Ash Charitable Foundation 075-07 (T. M. A.).
References
Full text links
Read article at publisher's site: https://doi.org/10.1016/b978-0-12-385504-6.00001-4
Read article for free, from open access legal sources, via Unpaywall:
https://www.ncbi.nlm.nih.gov/pmc/articles/PMC3697097
Citations & impact
Impact metrics
Citations of article over time
Smart citations by scite.ai
Explore citation contexts and check if this article has been
supported or disputed.
https://scite.ai/reports/10.1016/b978-0-12-385504-6.00001-4
Article citations
Pigs lacking TMPRSS2 displayed fewer lung lesions and reduced inflammatory response when infected with influenza A virus.
Front Genome Ed, 5:1320180, 31 May 2024
Cited by: 2 articles | PMID: 38883409 | PMCID: PMC11176495
Precision nutrition to reset virus-induced human metabolic reprogramming and dysregulation (HMRD) in long-COVID.
NPJ Sci Food, 8(1):19, 30 Mar 2024
Cited by: 2 articles | PMID: 38555403 | PMCID: PMC10981760
Review Free full text in Europe PMC
ACE2 and TMPRSS2 expression in patients before, during, and after SARS-CoV-2 infection.
Front Cell Infect Microbiol, 14:1355809, 28 Mar 2024
Cited by: 1 article | PMID: 38606293 | PMCID: PMC11007167
Copy Number Variation Regions Differing in Segregation Patterns Span Different Sets of Genes.
Animals (Basel), 13(14):2351, 19 Jul 2023
Cited by: 1 article | PMID: 37508128 | PMCID: PMC10376189
Intersection of Coagulation and Fibrinolysis by the Glycosylphosphatidylinositol (GPI)-Anchored Serine Protease Testisin.
Int J Mol Sci, 24(11):9306, 26 May 2023
Cited by: 1 article | PMID: 37298257 | PMCID: PMC10252689
Go to all (105) article citations
Similar Articles
To arrive at the top five similar articles we use a word-weighted algorithm to compare words from the Title and Abstract of each citation.
The cutting edge: membrane-anchored serine protease activities in the pericellular microenvironment.
Biochem J, 428(3):325-346, 01 Jun 2010
Cited by: 89 articles | PMID: 20507279 | PMCID: PMC3680374
Review Free full text in Europe PMC
Membrane anchored serine proteases: a rapidly expanding group of cell surface proteolytic enzymes with potential roles in cancer.
Cancer Metastasis Rev, 22(2-3):237-258, 01 Jun 2003
Cited by: 183 articles | PMID: 12784999
Review
Cell surface-anchored serine proteases in cancer progression and metastasis.
Cancer Metastasis Rev, 38(3):357-387, 01 Sep 2019
Cited by: 57 articles | PMID: 31529338 | PMCID: PMC6893142
Review Free full text in Europe PMC
Membrane-Anchored Serine Proteases and Protease-Activated Receptor-2-Mediated Signaling: Co-Conspirators in Cancer Progression.
Cancer Res, 79(2):301-310, 04 Jan 2019
Cited by: 35 articles | PMID: 30610085 | PMCID: PMC6335149
Review Free full text in Europe PMC
Funding
Funders who supported this work.
Intramural NIH HHS
NCI NIH HHS (4)
Grant ID: R01 CA098369
Grant ID: CA098369
Grant ID: P30 CA134274
Grant ID: R56 CA098369
NHLBI NIH HHS (5)
Grant ID: HL089298
Grant ID: HL084387
Grant ID: HL089298-S1
Grant ID: R01 HL084387
Grant ID: R01 HL089298
NIDDK NIH HHS (2)
Grant ID: DK081376
Grant ID: R01 DK081376