Abstract
Free full text

GNA33 of Neisseria meningitidis Is a Lipoprotein Required for Cell Separation, Membrane Architecture, and Virulence
Abstract
GNA33 is a membrane-bound lipoprotein with murein hydrolase activity that is present in all Neisseria species and well conserved in different meningococcal isolates. The protein shows 33% identity to a lytic transglycolase (MltA) from Escherichia coli and has been shown to be involved in the degradation of both insoluble murein sacculi and unsubstituted glycan strands. To study the function of the gene and its role in pathogenesis and virulence, a knockout mutant of a Neisseria meningitidis serogroup B strain was generated. The mutant exhibited retarded growth in vitro. Transmission electron microscopy revealed that the mutant grows in clusters which are connected by a continuous outer membrane, suggesting a failure in the separation of daughter cells. Moreover, sodium dodecyl sulfate-polyacrylamide gel electrophoresis analysis of culture supernatant revealed that the mutant releases several proteins in the medium. The five most abundant proteins, identified by matrix-assisted laser desorption ionization-time-of-flight mass spectrometry analysis, belong to the outer membrane protein family. Finally, the mutant showed an attenuated phenotype, since it was not able to cause bacteremia in the infant rat model. We conclude that GNA33 is a highly conserved lipoprotein which plays an important role in peptidoglycan metabolism, cell separation, membrane architecture, and virulence.
The shape and osmotic stability of most bacteria are maintained by the polymeric glycopeptide murein (peptidoglycan), a network of glycan strands interlinked by short peptides. Expansion of the cell wall during bacterial growth and splitting of the septum requires the coordinate action of enzymes which cleave covalent bonds within the murein sacculus and add new peptidoglycan units. For Escherichia coli, it has been proposed that a multienzyme complex (hydrolases and synthases) could be responsible for such a task (9). Thus, murein hydrolases, such as murein lytic transglycosylase A (MltA), are likely to be involved in the enlargement of the murein net and therefore to be essential for growth and division of the bacterial cell wall to allow separation of the daughter cells. Lytic transglycolases are a lysozyme subclass of murein hydrolases which not only cleave the β1-4 glycosidic bond between N-acetylmuramic acid and N-acetylglucosamine but also transfer the glycosyl bond onto the C-6 hydroxyl group of the terminal muramyl moiety, thus forming a 1,6-anhydromuramic acid residue.
During screening of the Neisseria meningitidis serogroup B genome to identify novel vaccine candidates and putative virulence factors, we identified a 47-kDa lipoprotein, genome-derived Neisseria antigen 33 (GNA33 [NMB0033]), that is highly conserved among N. meningitidis serogroup B strains as well as other Neisseria species (18). Database sequence comparison shows that GNA33 shares 33% identity and 41% homology with the 38-kDa membrane-bound MltA of E. coli. gna33 has been cloned and expressed in E. coli (18), and the lipoprotein nature of the recombinant protein has been demonstrated by incorporation of [3H]palmitate (11). The functional homology of GNA33 with MltA has been confirmed, since the recombinant protein is able to degrade both insoluble murein and unsubstituted glycan strands (11). The formation of 1,6-anhydrodisaccharide tri- and tetrapeptide reaction products demonstrated that GNA33 is a lytic transglycolase (11). In E. coli an MltA deletion mutant as well as mutants with double or triple mutations in the other lytic transglycosylases (MltB and Slt70) showed no obvious phenotype (6, 16, 21). Compared to the case for rod-shaped bacilli such as E. coli, little work has been done to understand the cell division process in gram-negative cocci.
To further define the function of GNA33 in N. meningitidis and its role in growth and cell separation, a knockout mutant was made. Here, we describe the construction and characterization of this mutant and show that GNA33 is essential for cell separation, membrane architecture, and virulence.
MATERIALS AND METHODS
Bacterial strains and growth conditions.
N. meningitidis strains MC58, NMB, and BZ232 and their respective gna33 mutants were grown on GC agar base or in GC broth with supplements at 37°C in 5% CO2, unless otherwise stated. For selection of transformants, erythromycin was used at a concentration of 7 μg/ml. E. coli strain DH5 was used for cloning and grown on Luria-Bertani agar base or in Luria-Bertani medium supplemented with ampicillin (100 μg/ml) or erythromycin (200 μg/ml).
Construction of ΔGNA33 mutants of N. meningitidis.
Mutants of strains MC58, NMB, and BZ232 (MC58 ΔGNA33, NMB ΔGNA33, and BZ232 ΔGNA33, respectively) in which the gna33 gene was replaced by allelic exchange with an antibiotic cassette were prepared by transforming the parent strain with the plasmid pBSUDGNA33ERM. This plasmid contains upstream and downstream flanking regions for allelic exchange, a truncated gna33 gene, and the ermC gene (encoding erythromycin resistance). The upstream flanking region (including the start codon) from position −867 to +75 and the downstream flanking region (including the stop codon) from position +1268 to +1744 were amplified from MC58 by using the primers U33FOR (5′-GCTCTAGAGATGAGTCGAACACAATGAACAATGTCCTGA [the XbaI site is underlined]), U33REV (5′-TCCCCCGGGCTCTTGCTTTGGCAGGCGGCGA [the SmaI site is underlined]), D33FOR (5′-TCCCCCGGGCACGGGATATGTGTGGC [the SmaI site is underlined]), and D33REV (5′-CCCGCTCGAGAGTAGGGACAACCGG [the XhoI site is underlined]). Fragments were cloned into pBluescript (Stratagene, Milan, Italy) and transformed into E. coli DH5 by using standard techniques (19). Once all subcloning was complete, naturally competent Neisseria strains MC58, NMB, and BZ232 were transformed by selecting a few colonies grown overnight on GC agar plates and mixing them with 20 μl of 10 mM Tris-HCl (pH 6.5) containing 1 μg of plasmid DNA. The mixture was spotted onto a chocolate agar plate, incubated for 6 h at 37°C with 5% CO2, and then diluted in phosphate buffered-saline (PBS) and spread on GC agar plates containing 7 μg of erythromycin per ml. PCR with forward primer 5′-CGCGGATCCCATATGCAAAGAAAGAGAATCCCAA and reverse primer 5′-GCTCTGAGGGCGACGACAGGCGG was used to verify that correct allelic exchange with the chromosomal gna33 gene had occurred, as indicated by the absence of an 800-bp PCR product, indicating that the gene was replaced by a 1.2-kb fragment resulting in the insertion of the erythromycin cassette. Lack of GNA33 expression was confirmed by Western blot analysis as described below.
Preparation of outer membrane vesicles (OMVs).
N. meningitidis strains MC58, MC58 ΔGNA33, BZ232, and BZ232 ΔGNA33 were grown overnight at 37°C with 5% CO2 on five GC plates, harvested with a loop, and resuspended in 10 ml of 20 mM Tris-HCl (pH 7.5)-2 mM EDTA. Heat inactivation was performed at 56°C for 45 min, and the bacteria were disrupted by sonication for 5 min on ice (50% duty cycle, 50% output; Branson Sonifier with a 3-mm microtip). Unbroken cells were removed by centrifugation at 5,000 × g for 10 min, and the supernatant containing the total cell envelope fraction was recovered and further centrifuged overnight at 50,000 × g at 4°C. The pellet containing the membranes was resuspended in 2% Sarkosyl-20 mM Tris-HCl (pH 7.5)-2 mM EDTA and incubated at room temperature for 20 min to solubilize the inner membranes. The suspension was centrifuged at 10,000 × g for 10 min to remove aggregates, and the supernatant was further centrifuged at 50,000 × g for 3 h. The pellet, containing the outer membranes, was washed in PBS and resuspended in the same buffer. The protein concentration was measured by the Bio-Rad (Hercules, Calif.) detergent-compatible protein assay (modified Lowry method), using bovine serum albumin (BSA) as a standard.
Preparation of whole-cell extracts.
N. meningitidis strains MC58, MC58 ΔGNA33, BZ232, and BZ232 ΔGNA33 were grown overnight on a GC plate at 37°C in 5% CO2. Colonies from each strain were collected and used to inoculate 7 ml of Mueller-Hinton broth, containing 0.25% glucose, to reach an optical density at 620 nm (OD620) of 0.05 to 0.06. The culture was incubated for approximately 2.5 h at 37°C with shaking until an OD620 of 0.5 was reached and then centrifuged for 10 min at 1,000 × g. The supernatant was discarded, and the pellet was resuspended in 500 μl of PBS. Heat inactivation was performed at 56°C for 30 min.
TCA precipitation of proteins from culture supernatant.
N. meningitidis strains MC58 and MC58 ΔGNA33 were grown in 200 ml of GC culture medium in a humidified atmosphere containing 5% CO2 to an OD620 of 0.5. Bacterial cells were removed by a 10-min centrifugation at 6,400 × g, and the culture medium was filtered through a 0.22-μm-pore-size filter (Millipore, Bedford, Mass.). Proteins were precipitated with 10% trichloracetic acid (TCA)-0.4% deoxycholate (30 min at 4°C), and the pellet was successively washed with 1% TCA and acetone and dried with Speed-Vac (Savant, Holbrook, N.Y.). The pellet was solubilized with 15 mM Tris-HCl (pH 8.8)-1 mM dithiothreitol.
SDS-PAGE and Western blot analysis.
Total cell extract, TCA-precipitated culture medium, and OMV preparations of strains MC58, MC58 ΔGNA33, BZ232, and BZ232 ΔGNA33 were analyzed with a sodium dodecyl sulfate (SDS)-10% polyacrylamide gel as described by Laemmli (14), using a Mini-Protean II electrophoresis apparatus (Bio-Rad). Samples were suspended in SDS sample buffer (0.06 M Tris-HCl [pH 6.8], 10% [vol/vol] glycerol, 2% [wt/vol] SDS, 5% [vol/vol] 2-mercaptoethanol, 10 μg of bromophenol blue per ml) and heated to 100°C for 5 min before SDS-polyacrylamide gel electrophoresis (SDS-PAGE) (3 μg/lane). For Western blotting, the gel was equilibrated with buffer (48 mM Tris-HCl, 39 mM glycine [pH 9.0], 20% [vol/vol] methanol) and transferred to a nitrocellulose membrane (Bio-Rad) by using a Trans-Blot (Bio-Rad) semidry electrophoretic transfer cell. The nitrocellulose membranes were blocked with 10% (wt/vol) skim milk in PBS containing 0.2% (wt/vol) sodium azide. Anti-His tag-GNA33 monoclonal antibody (MAb) 25 (8) was diluted in PBS containing 1% (wt/vol) BSA and 1% (wt/vol) Tween 20. Bound antibody was detected by using rabbit anti-mouse immunoglobulin (immunoglobulins G, A, and M)-alkaline phosphatase conjugate polyclonal antibody (Zymed, South San Francisco, Calif.) and Sigma Fast 5-bromo-4-chloro-3-indolylphosphate-nitroblue tetrazolium substrate (Sigma Aldrich, St. Louis, Mo.).
MALDI-TOF mass spectrometry.
Protein bands were excised from the gel, washed with 100 mM ammonium bicarbonate-acetonitrile (50:50, vol/vol), and dried with a Speed-Vac centrifuge (Savant). The dried spots were digested at 37°C for 2 h by adding 7 to 10 μl of a solution containing 50 mM ammonium bicarbonate, 5 mM dithiothreitol, and 0.012 μg of sequencing-grade trypsin (Promega, Madison, Wis.) per μl. After digestion, 5 μl of 0.1% trifluoroacetic acid was added, and the peptides were desalted and concentrated with ZIP-TIPs (C18; Millipore). Samples were eluted onto the mass spectrometer target (Anchorchip 384 to 400 μm; Bruker, Bremen, Germany) with 2 μl of 2,5-dihydroxybenzoic acid (5 g/liter) in 50% acetonitrile-0.1% trifluoroacetic acid and allowed to air dry at room temperature. Matrix-assisted laser desorption ionization-time-of-flight (MALDI-TOF) spectra were acquired on a Biflex III MALDI-TOF apparatus (Bruker) equipped with a 337-nm N2 laser and a SCOUT 384 multiprobe. Spectra were acquired in positive mode; acceleration and reflector voltages were set at 19 and 20 kV, respectively. Typically, each spectrum was determined by averaging 100 laser shots. Spectra were externally calibrated by using a combination of four standards (angiotensin II [1,046.54 Da], substance P [1,347.74 Da], bombesin [1,619.82 Da], and ACTH18-39 clip human [2,465.20 Da]) spotted on the probe adjacent to the samples. Protein identification was carried out by both automatic and manual comparisons of experimentally generated monoisotopic peaks of peptides in the mass range of 700 to 3,000 Da with computer-generated fingerprints, using the Mascot program run on proprietary databases.
Growth experiments.
All growth experiments were carried out at 37°C with 5% CO2. The suspension of organisms was adjusted to an initial OD620 of 0.05 in 7 ml of GC medium and incubated with continuous end-over-end rotation. The OD was measured every 20 min, and at each time point bacteria were plated out in triplicate to obtain the corresponding CFU per milliliter. Growth studies were preformed three times. To determine whether the ΔGNA33 mutant is able to grow in complement-inactivated serum, both the wild-type and mutant strains were grown for 3 h in heat-treated (56°C, 30 min) rabbit serum. Serial dilutions were carried out, and bacteria were plated out to determine CFU.
Transmission electron microscopy.
Bacteria were grown in GC medium to an OD620 of 0.5 and centrifuged, and the pellets were fixed for 1 h in 2.0% glutaraldehyde diluted in PBS. After fixation, the pellets were washed with PBS and then postfixed in 1% osmium tetroxide for 1 h at 4°C. Samples were then dehydrated with a graded series of ethanol solutions and embedded in Epon-Araldite. Ultrathin sections were routinely stained with uranyl acetate. The samples were then viewed and photographed in a Philips CM10 transmission electron microscope operated at 80 kV.
Virulence in infant rats.
The ability of BZ232 ΔGNA33 to cause bacteremia was tested in infant rats challenged intraperitoneally (i.p.). The assay was preformed as described previously (17). In brief, 5- to 8-day-old-pups from litters of outbred Wistar rats (Charles River, Raleigh, N.C.) were randomly redistributed to the nursing mothers. After overnight growth on chocolate agar, several colonies were inoculated into Mueller-Hinton broth (starting OD620 of ~0.1), and the test organism was grown for approximately 2.5 h to an OD620 of ~0.6 (early log phase). The bacteria were washed once in PBS plus 1% BSA and diluted to the required CFU per milliliter, and 100 μl of the mixture was administered i.p. Blood specimens were obtained 18 h after the bacterial challenge by puncturing the heart with a needle and syringe containing approximately 10 μl of heparin (1,000 U/ml; Fujisawa USA, Deerfield, Ill.) without preservative. Aliquots of 1, 10, and 100 μl of blood were plated onto chocolate agar. The CFU per milliliter of blood was determined after overnight incubation of the plates at 37°C with 5% CO2. To determine whether the mutant is sensitive to heparin, the parent and mutant strains were resuspended in PBS and incubated with or without heparin for 10 min. Bacteria were then plated out to determine the CFU.
RESULTS
GNA33 knockout characterization.
A mutation of the gna33 gene was generated in strains MC58, NMB, and BZ232, and the deletion of the gene was confirmed by PCR with chromosomal DNA. Protein expression was analyzed by immunoblotting in bacterial total lysates or in OMV preparations from wild-type and mutant strains, using anti-GNA33 MAb 25, which recognizes GNA33 and a mimotope present in serotype P1.2 of PorA (8). The results reported in Fig. Fig.11 show that on the 2996 strain, used as positive control, the MAb recognizes two bands of 41 and 47 kDa. The upper band of 47 kDa corresponds to the GNA33 protein, whereas the lower band of 41 kDa corresponds to the PorA antigen, consistently with the finding that the GNA33 antigen mimics a surface-exposed epitope on loop 4 of PorA in strains of serotype P1.2 (8). The 47-kDa band, corresponding to the GNA33 protein, is present in the OMV preparations and total lysates of MC58 (lanes 2 and 3, respectively) and is absent in the OMV preparation and total lysate of MC58 ΔGNA33 (lanes 4 and 5). In the case of the BZ232 strain, there are two reactive bands in the total cell lysates and OMV preparations (lanes 6 and 7), but only the 41-kDa band is present in the BZ232 ΔGNA33 mutant (lanes 8 and 9), consistent with its P1.2 serotype. The antibodies were not able to recognize GNA33 on the surface of the MC58 strain by fluorescence-activated cell sorter analysis, suggesting that the protein is not surface exposed (data not shown).
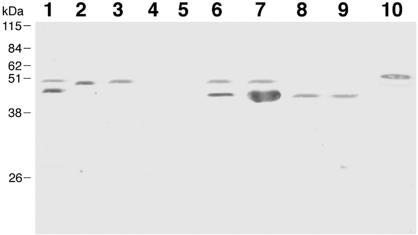
Detection of GNA33 in Western blotting analysis of recombinant GNA33, total lysates, and OMV preparations with anti-GNA33 MAb 25. Lane 1, control strain 2996; lane 2, MC58 OMVs; lane 3, MC58 total lysate; lane 4, MC58 ΔGNA33 OMVs; lane 5, MC58 ΔGNA33 total lysate; lane 6, BZ232 OMVs; lane 7, BZ232 total lysate; lane 8, BZ232 ΔGNA33 OMVs; lane 9, BZ232 ΔGNA33 total lysate; lane 10, recombinant GNA33 from strain 2996. As expected, no reactivity is observed with the mutant strains; however, the antiserum cross-reacts with another band at 47 kDa. This band has been characterized as PorA (8).
The GNA33 mutant exhibits aberrant cell morphology and decreased viability.
To evaluate whether GNA33 has any influence on the growth rate or colony morphology of N. meningitidis, the wild-type MC58, NMB, and BZ232 strains and the mutant MC58 ΔGNA33, NMB ΔGNA33, and BZ232 ΔGNA33 strains were grown on solid or liquid medium. Following overnight incubation of the strains on agar plates, no differences in colony morphology between wild-type and mutant strains could be visualized with the naked eye or with a light microscope (data not shown). To evaluate the influence on growth, the strains were grown for 5 h at 37°C, and samples were collected every 20 min. Each sample was evaluated for OD and for the number of bacterial colonies by plating on GC agar plates. The results of the experiment related to the MC58 and MC58 ΔGNA33 strains are reported in Fig. Fig.2.2. As shown, the ΔGNA33 mutant exhibits a retardation in growth compared to the parent MC58 strain (Fig. (Fig.2a).2a). Furthermore, the number of CFU of MC58 ΔGNA33 per milliliter was much lower than that of the wild-type MC58 strain (Fig. (Fig.2b).2b). At an OD620 of 0.5, the numbers of viable colonies were 6.7 × 108 CFU/ml for the MC58 strain and 4.2 ×107 CFU/ml for the mutant strain, indicating duplication times of approximately 20 min for MC58 and 40 min for MC58 ΔGNA33, resulting in a dramatic reduction in the viable counts. The same results were obtained with the other strains (data not shown).
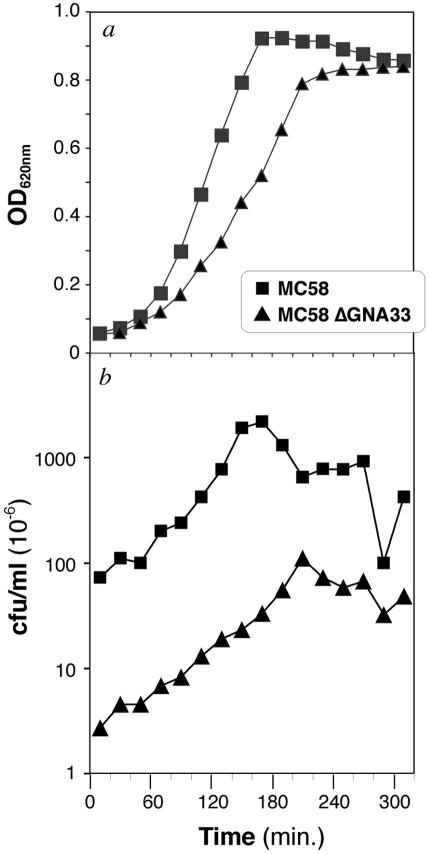
Comparison of the viabilities of the wild-type MC58 and the GNA33 knockout mutant on the basis of OD620 (a) and CFU per milliliter (b). The mutant MC58 ΔGNA33 strain shows a significantly reduced growth rate and lower CFU with respect to the parent strain.
To verify whether GNA33 plays a role in cell size or cell shape, transmission electron microscopy was used to examine the morphology of the GNA33 mutant. As shown in Fig. Fig.3,3, the mutant morphology (Fig. 3c and d) is different from the typical diplococcal morphology of the wild-type MC58 (Fig. 3a and b). The mutant grows in clusters (Fig. (Fig.3c),3c), and while the inner membrane is separated, the outer membrane remains continuous around the clusters (Fig. (Fig.3d),3d), resulting in abnormal cell morphology, with cells of various sizes with an undivided septum. The clusters are represented by cells which are unable to separate. The fact that the mutant grows in clusters is likely to result in misleading outcomes with the colony counts. The bacterial colonies obtained with the mutant strain may be generated by clusters of cells and not by individual bacteria. The NMB and BZ232 mutants exhibited the same morphology (data not shown), confirming that the phenotype observed is a result of the deletion of the gna33 gene and not a strain-dependent phenomenon.
The GNA33 mutant releases outer membrane proteins.
Since the mutant is impaired in cell separation, we investigated whether the mutation in the gna33 gene may also affect membrane protein assembly. To address this issue, we analyzed the extracellular proteins present in strain MC58 and MC58 ΔGNA33 culture supernatants at an OD620 of 0.5. The culture medium was filtered through a 0.22-μm-size-pore membrane and concentrated by TCA precipitation. Twenty micrograms of protein was separated by SDS-PAGE (Fig. (Fig.4).4). In the culture medium of the mutant strain MC58 ΔGNA33 (Fig. (Fig.4,4, lane 4), several proteins not present in the supernatant of the wild-type strain (Fig. (Fig.4,4, lane 3) were visible on the gel. The five most abundant proteins (Fig. (Fig.4,4, lane 4) were in-gel digested with trypsin and analyzed by MALDI-TOF mass spectrometry for protein identification. The proteins were identified as the outer membrane protein PorA (NMB1429), the major outer membrane PIB (NMB2039), the class 4 outer membrane protein (NMB0382), the class 5 outer membrane protein (NMB1053), and a putative adhesin protein (NMB2095). With the exception of NMB2095, the other proteins are also the major proteins identified in the MC58 OMV preparation (Fig. (Fig.4,4, lane 5). The only two faint bands visible in the culture supernatant of the wild-type culture (Fig. (Fig.4,4, lane 3) were both identified as the class 5 outer membrane protein (NMB1053). In conclusion, the deletion in the gna33 gene seems to affect correct topological organization of the N. meningitidis membrane, resulting in the selective release of several outer membrane proteins.
Virulence of the GNA33 knockout mutant.
We investigated the ability of the mutant strain to cause bacteremia in infant rats. Since previous experiments had shown that MC58 is unable to cause consistent and reproducible bacteremia in infant rats (data not shown), the BZ232 strain and its GNA33 mutant were used in the study. The virulence experiment was carried out by challenging 5-day-old infant rats i.p with 102 and 105 CFU of BZ232 or BZ232 ΔGNA33. Blood samples were obtained after 18 h and plated onto chocolate agar plates. The results obtained are summarized in Table Table1.1. At both challenge doses of 2.5 × 102 and 2.5 × 105 CFU, the parent BZ232 strain caused bacteremia, while the mutant was unable to cause bacteremia even at the highest challenge dose. As shown in Table Table1,1, all three animals given a challenge dose of approximately 105 CFU of strain BZ232 ΔGNA33 did not exhibit bacteremia after 18 h. In a second experiment, infant rats were challenged with 108 CFU of BZ232 ΔGNA33, and even at this dose the mutant strain failed to cause bacteremia. It is worth noting that the challenge dose of the mutant strain is based on the CFU of the clusters of bacteria, and thus a more bacteria are inoculated than with the wild-type strain.
TABLE 1.
N. meningitidis strain BZ232 in which GNA33 has been deleted is unable to cause bacteremia and death in infant rats challenged i.p.
Exp | Strain | Bacterial dose (CFU) | Results of blood culture 18 h after challenge
| |
---|---|---|---|---|
No. positive/total | Mean CFU/ml | |||
1 | BZ232 (wild type) | 2.5 × 102 | 4/4 | 2.52 × 106 |
2.5 × 105 | 3/3 | 1 × 107 | ||
BZ232 ΔGNA33 | 2 × 102 | 0/4 | ||
2 × 105 | 0/3 | |||
2 | BZ232 ΔGNA33 | 4.6 × 108 | 0/4 |
To verify whether the ΔGNA33 mutant is unable to cause bacteremia because of a susceptibility to killing in presence of heparin or to lyse in the serum, the wild-type and mutant strains were incubated with or without heparin for 10 min or grown in heat-treated serum for 3 h. For the wild-type strain, 14.3 × 105 bacteria were obtained in the absence of heparin, compared to 13.6 × 105 in the presence of heparin. In the case of the mutant strain, 2.6 × 104 bacteria were obtained in the absence of heparin, compared to 1.3 × 104 bacteria when incubated with heparin. These results show that the mutant strain is not more susceptible to killing in the presence of heparin than the parent strain. When the mutant was grown in heat-inactivated serum, the numbers of CFU at time zero for the wild-type strain and mutant strain were 2.3 × 107 and 8.5 × 105, respectively. After 3 h of incubation, the numbers of CFU for the wild-type strain and mutant strain were 1.7 × 109 and 4 × 107, respectively. The results therefore suggest that the mutant strain is able to grow in heat-treated serum. Moreover, the mutant strain exhibits a retardation in growth compared to the parent strain, consistent with data obtained when the strains were grown in GC broth.
DISCUSSION
In this study we show that deletion of the N. meningitidis gene coding for GNA33, which is homologous to the lytic transglycosylase MltA of E. coli, affects cell separation (preventing the formation of daughter cells) cell membrane assembly (causing a release of outer membrane proteins), and virulence. Instead of the characteristic diplococci, the GNA33 mutant shows abnormal cell morphology, which includes an undivided septum, with cells of various sizes growing in clusters of up to 17 cells. The bacterial cells in these clusters have a double septum and a continuous outer membrane.
In E. coli, a model for the coordinated metabolism of the murein layer has been suggested (9). The basis of the model is a multienzyme complex consisting of bifunctional murein transglycolsylases-transpeptidases (known as penicillin-binding proteins), lytic transglycosylases (Slt70, MltA, and MltB), and MipA (9). It has been suggested that there might be two such complexes, one for the synthesis of murein and another for its degradation. Formation of a complex could be a way for the secure and effective control of potentially autolytic murein hydrolases and to ensure that the growth of the sacculus occurs to maintain the specific shape of the bacterium. Genome computer analysis has shown that N. meningitidis has homologs of a majority of the complex members. In addition, by using affinity chromatography, interaction between GNA33 and penicillin-binding protein 2 from N. meningitidis serogroup B has been shown (11), further suggesting that GNA33 may be part of a multienzyme complex, as is the case for MltA in E. coli. Deletion of three lytic transglycosylases in E. coli did not influence growth and division of the mutant E. coli strains. In this study we report that deletion of a protein of N. meningitidis with homology to the E. coli lytic transglycosylase MltA and with a similar in vitro enzymatic activity (11) causes a dramatic effect on growth and cell division. One of the reasons for different phenotypes induced by the deletion of MltA in E. coli and GNA33 in N. meningitidis may be the different cell shape of the bacterium: E. coli cells are rod shaped, while neisseriae are diplococcal. Enzymes shaping the form of the cells may have slightly different roles or be components of different complexes. This observation may represent a first step in the understanding of the mechanisms which regulate cell shapes. The cell division process in gram-negative cocci is essentially uncharacterized. This study suggests that we may learn more by studying the cell shape in cocci. The MinD mutant of Neisseria gonorrhoeae (N. gonorrhoeae MinD is homologous to the MinD protein of E. coli [3, 4]) exhibits a phenotype similar to that of our GNA33 mutant (7). Abnormal cell morphology has been also observed in mutants with mutations in murein hydrolase genes, such as tcp in N. gonorrhoeae (7), Cwl (lyt) in Bacillus subtilis (10), and iap in Listeria (13), but we have not been able to find any sequence homology between these enzymes and GNA33.
The gna33 gene deletion not only perturbs the cell separation process but also seems to affect membrane architecture. By analyzing the culture supernatant of the MC58 mutant strain, we found that several proteins are specifically released in the medium. The five most abundant released proteins, unambiguously identified by MALDI-TOF mass spectrometry, belong to the outer membrane family. Moreover, four of these proteins are major outer membrane proteins of MC58. Further analysis by two-dimensional electrophoresis has allowed the identification of more than 50 membrane proteins (unpublished data). At present we can only speculate on the possible mechanism of selective membrane protein release, which can occur either because of a physical dissociation of membrane material leading to the formation of membrane vesicles or because of selective leakage (secretion) of outer membrane proteins. Shedding of membrane vesicles during the active phase of growth is known to occur in many gram-negative bacteria (12, 22, 23, 24), including N. meningitidis (5). Interestingly, loss of membrane material is significantly increased in the absence of a specific subset of inner membrane, periplasmic, and outer membrane proteins that share the property of strongly interacting with the murein layer and form large complexes which physically link the inner and outer membranes, maintaining envelope integrity. Among these proteins are the Braun's (murein) lipoprotein (Lpp) and the proteins belonging the Tol-Pal system. This system includes the peptidoglycan-associated lipoprotein (PAL), a protein anchored to the outer membrane; the periplasmic protein TolB; and the inner membrane complex formed by the TolQ, TolR, and TolA proteins (15). Any mutation in lpp and the tol-pal system perturbs the outer membrane and causes the formation of OMVs (1, 2, 15). A similar phenotype might be envisaged for the ΔGNA33 mutant, reflecting the close interaction of the GNA33 protein with the murein layer.
The GNA33 knockout mutant was unable to cause bacteremia in the infant rat model even at the highest dose used. This attenuated phenotype is likely to be due to an inability of the mutant strain to multiply in the bloodstream, probably because of higher sensitivity of the bacterial cluster to complement-mediated bacterial lysis. Using insertional mutagenesis, Sun et al. (20) identified several genes essential for pathogenesis. One of the genes identified, NMB1279, is the homolog of the E. coli lytic transglycosylase MltB gene. However, the Neisseria mutant with the deletion in the NMB1279 gene that we generated exhibits a normal diplococcal phenotype and normal growth and does not release outer membrane proteins (data not shown).
In conclusion, we have shown that GNA33, a highly conserved lipoprotein from N. meningitidis, is required for maintaining proper cell division, morphology, membrane architecture, and virulence of meningococci. The inability of the GNA33 mutant to cause bacteremia in infant rats suggests that GNA33 may be a good candidate for antimicrobial drugs.
Acknowledgments
We thank Renzo Nogarotto for helpful discussions, Davide Mercati for technical assistance, Catherine Mallia for editing, and Giorgi Corsi for artwork.
J. Adu-Bobie was a recipient of a Marie Curie Fellowship from the European Union.
Notes
Editor: J. N. Weiser
REFERENCES
Articles from Infection and Immunity are provided here courtesy of American Society for Microbiology (ASM)
Full text links
Read article at publisher's site: https://doi.org/10.1128/iai.72.4.1914-1919.2004
Read article for free, from open access legal sources, via Unpaywall:
https://europepmc.org/articles/pmc375154?pdf=render
Citations & impact
Impact metrics
Citations of article over time
Alternative metrics
Smart citations by scite.ai
Explore citation contexts and check if this article has been
supported or disputed.
https://scite.ai/reports/10.1128/iai.72.4.1914-1919.2004
Article citations
An MltA-Like Lytic Transglycosylase Secreted by Bdellovibrio bacteriovorus Cleaves the Prey Septum during Predatory Invasion.
J Bacteriol, 205(4):e0047522, 03 Apr 2023
Cited by: 2 articles | PMID: 37010281 | PMCID: PMC10127604
Towards a Four-Component GMMA-Based Vaccine against Shigella.
Vaccines (Basel), 10(2):328, 18 Feb 2022
Cited by: 29 articles | PMID: 35214786 | PMCID: PMC8880054
Review Free full text in Europe PMC
The AmiC/NlpD Pathway Dominates Peptidoglycan Breakdown in Neisseria meningitidis and Affects Cell Separation, NOD1 Agonist Production, and Infection.
Infect Immun, 90(3):e0048521, 14 Feb 2022
Cited by: 3 articles | PMID: 35225652 | PMCID: PMC8929373
Outer Membrane Vesicle Induction and Isolation for Vaccine Development.
Front Microbiol, 12:629090, 04 Feb 2021
Cited by: 35 articles | PMID: 33613498 | PMCID: PMC7889600
Review Free full text in Europe PMC
Identification of Membrane-Bound Lytic Murein Transglycosylase A (MltA) as a Growth Factor for Francisella novicida in a Silkworm Infection Model.
Front Cell Infect Microbiol, 10:581864, 22 Jan 2021
Cited by: 5 articles | PMID: 33553001 | PMCID: PMC7862118
Go to all (39) article citations
Similar Articles
To arrive at the top five similar articles we use a word-weighted algorithm to compare words from the Title and Abstract of each citation.
GNA33 from Neisseria meningitidis serogroup B encodes a membrane-bound lytic transglycosylase (MltA).
Eur J Biochem, 269(15):3722-3731, 01 Aug 2002
Cited by: 26 articles | PMID: 12153569
A novel mimetic antigen eliciting protective antibody to Neisseria meningitidis.
J Immunol, 167(11):6487-6496, 01 Dec 2001
Cited by: 28 articles | PMID: 11714816
Role of transferrin receptor from a Neisseria meningitidis tbpB isotype II strain in human transferrin binding and virulence.
Infect Immun, 72(6):3461-3470, 01 Jun 2004
Cited by: 22 articles | PMID: 15155653 | PMCID: PMC415691
[Neisseria meningitidis. The pathophysiological role of lipopolysaccharides in association with meningococcal disease and septic shock].
Ugeskr Laeger, 170(6):421-426, 01 Feb 2008
Cited by: 0 articles | PMID: 18252172
Review
Funding
Funders who supported this work.
NIAID NIH HHS (2)
Grant ID: R01 AI046464
Grant ID: R01 AI046464-01A1