Abstract
Free full text

Mitochondria in Cardiovascular Physiology and Disease
Mitochondrial reactive oxygen species: which ROS signals cardioprotection?
Abstract
Mitochondria are the major effectors of cardioprotection by procedures that open the mitochondrial ATP-sensitive potassium channel (mitoKATP), including ischemic and pharmacological preconditioning. MitoKATP opening leads to increased reactive oxygen species (ROS), which then activate a mitoKATP-associated PKCε, which phosphorylates mitoKATP and leaves it in a persistent open state (Costa AD, Garlid KD. Am J Physiol Heart Circ Physiol 295, H874–H882, 2008). The ROS responsible for this effect is not known. The present study focuses on superoxide (O2·−), hydrogen peroxide (H2O2), and hydroxyl radical (HO˙), each of which has been proposed as the signaling ROS. Feedback activation of mitoKATP provides an ideal setting for studying endogenous ROS signaling. Respiring rat heart mitochondria were preincubated with ATP and diazoxide, together with an agent being tested for interference with this process, either by scavenging ROS or by blocking ROS transformations. The mitochondria were then assayed to determine whether or not the persistent phosphorylated open state was achieved. Dimethylsulfoxide (DMSO), dimethylformamide (DMF), deferoxamine, Trolox, and bromoenol lactone each interfered with formation of the ROS-dependent open state. Catalase did not interfere with this step. We also found that DMF blocked cardioprotection by both ischemic preconditioning and diazoxide. The lack of a catalase effect and the inhibitory effects of agents acting downstream of HO˙ excludes H2O2 as the endogenous signaling ROS. Taken together, the results support the conclusion that the ROS message is carried by a downstream product of HO˙ and that it is probably a product of phospholipid oxidation.
reactive oxygen species (ROS) are second messengers of preconditioning (21) and have long been known to be required for cardioprotective signaling (3, 10, 18, 34, 43, 49). The mechanism of increased ROS is reasonably well understood: signaling from the plasma membrane leads to opening of the mitochondrial ATP-sensitive K+ channel (mitoKATP; Ref. 19), and the increased K+ influx into the matrix causes an increase in ROS, which derive during normoxia from complex I of the respiratory chain (1).
The ROS transformations that take place in mitochondria are summarized in Figs. 1 and and2.2. The primary ROS produced by the mitochondrial respiratory chain is superoxide (O2·−), which is formed by single-electron reduction of oxygen. The majority of O2·− undergoes dismutation to hydrogen peroxide (H2O2) both spontaneously and through the action of superoxide dismutases located in the matrix and the intermembrane space (7, 26, 33). Much of the H2O2 generated is reduced by the glutathione and thioredoxin reductase antioxidant systems, which prevent the emission of excess H2O2 to the cytosol (42). H2O2 is also decomposed by catalase, which is present in the matrix of heart mitochondria. Some of the H2O2 leads to formation of hydroxyl radical (HO˙) through the Fenton reaction of H2O2 with transition metal ions (29). The highly reactive HO˙ oxidizes proteins and lipids in diffusion-limited reactions (27).
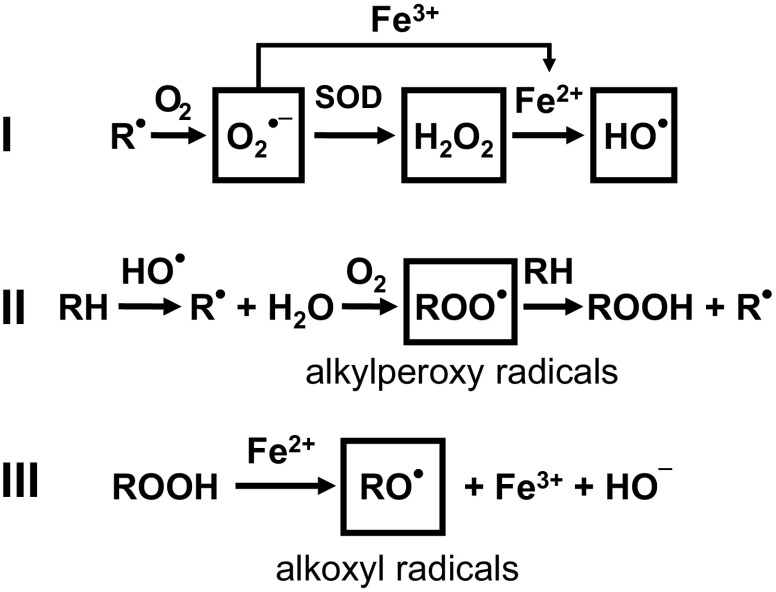
Mitochondrial electron transport chain-derived ROS and formation of alkyl peroxyl alkoxyl radicals. I: superoxide (O2·−) is formed by single electron transfer to molecular oxygen (O2) from a reduced group () in the respiratory chain and converted to hydrogen peroxide (H2O2) by superoxide dismutase (SOD). Hydroxyl radical (HO˙) is formed from H2O2 in the presence of a transition metal ion such as iron (Fe2+/3+; the Fenton reaction). II: subsequent reactions include the formation of alkyl peroxyl radicals (RO
) and alkyl hydroperoxides (ROOH). HO˙ acts on an alkyl side chain (RH) to form a reduced group (
) that leads to the formation of RO
, and these react with RH to produce ROOH. III: alkoxyl radicals (R
) are formed in the decomposition of hydroperoxides (ROOH) by transition metal ions (Fe2+).
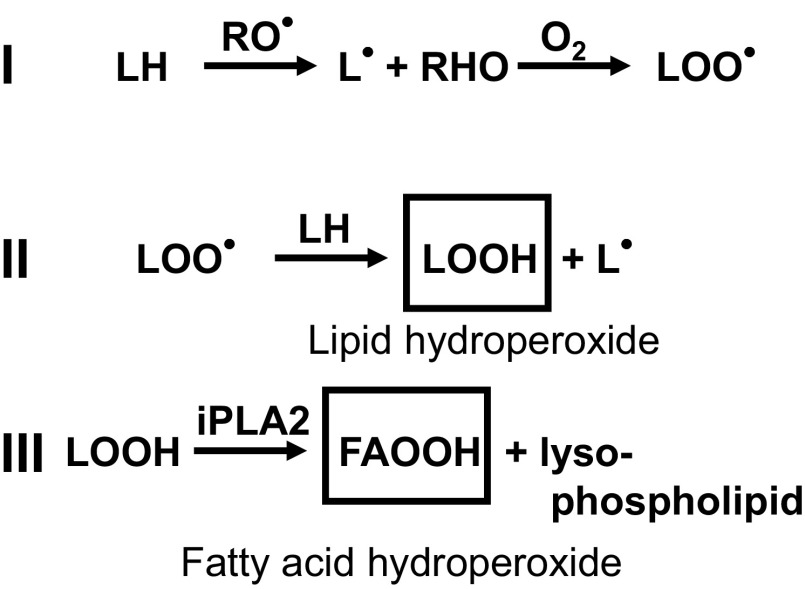
Lipid peroxidation and release of hydroperoxy fatty acids. I and II: alkoxyl (R), hydroxyl (RHO), and alkyl peroxyl radicals (LO
) can initiate the nonenzymatic chain reaction leading to lipid peroxidation (LOOH). III: lipid hydroperoxides (LOOH) can be released by the action of mitochondrial phospholipases, such as Ca2+-independent phospholipases PLA2 (iPLA2), which have been found to modulate the function of mitochondria [see Cedars et al. (8) for a review]. The action by iPLA2 results in a hydroperoxy fatty acid (FAOOH).
The consequences of ROS signaling for mitochondrial physiology are that two mitochondrial protein kinase-Cε (PKCε) are activated by oxidation of their thiol groups (28). Activation of PKCε1, which is associated with mitoKATP at the mitochondrial inner membrane, leads to opening of mitoKATP (13, 25). Activation of PKCε2 leads to inhibition of the mitochondrial permeability transition (MPT; Ref. 12). Progress is being made in these areas and in the molecular identification of mitoKATP (17). However, the ROS responsible for activating these PKCεs in vitro or in vivo is still not known. Because the signaling ROS not only originates in mitochondria but also acts on mitochondria, it should be possible to narrow the search for the signaling ROS by studies on isolated rat heart mitochondria. The objective of these studies was to identify the point in the reaction sequence of ROS transformations at which the ROS signal is formed and, ultimately, to determine the identity of the ROS signal itself.
We previously described feedback activation of mitoKATP, in which mitoKATP opening by a KATP channel opener leads to endogenous ROS formation and a persistent open state of mitoKATP. The phenomenon was shown to involve PKCε1 and an inner membrane phosphorylation event (13). This persistent open state of mitoKATP is thought to be responsible for “memory,” which is seen with all preconditioning (13).
We employed a preincubation protocol to probe the feedback activation system for the identity of the signaling ROS. Diazoxide was used to induce mitoKATP opening, which leads to endogenous ROS formation and activation of PKCε1. This process establishes the prolonged phosphorylation-dependent open state of mitoKATP (13). We then reisolated the mitochondria and probed them for this state by measuring the resulting steady-state mitochondrial matrix volume using the light-scattering technique (5, 11, 14, 20). Agents or conditions that interfered with any step in the generation of the signaling ROS would prevent formation of the phosphorylation dependent open state. Two additional assays were carried out to eliminate agents that interfered either with mitoKATP-dependent K+ uptake or with ROS activation of PKCε1. Together, these procedures allowed identification of agents that specifically interfered with formation of the ROS signal.
The agents that were found to block formation of the ROS signal were dimethylsulfoxide (DMSO) and dimethylformamide (DMF), scavengers of HO˙ and R (2, 32); Trolox, a chain-breaking antioxidant and peroxyl radical scavenger (31); deferoxamine, an iron chelator (29, 41); and bromoenol lactone (BEL), which is an inhibitor of Ca2+-independent phospholipase (iPLA2; Ref. 8). Based on the site of action of these agents, we conclude that the ROS signal arises downstream of HO˙ formation. Because H2O2 is produced upstream of HO˙, these results exclude H2O2 as the signaling ROS. This conclusion is supported by the finding that catalase did not interfere with formation of the phosphorylation-dependent open state of mitoKATP.
We performed experiments on MPT in which diazoxide was used to induce inhibition of MPT via ROS activation of PKCε2 (12). This effect was blocked by DMSO, also implicating a ROS signal downstream from HO˙. Finally, we found that the HO˙ scavenger DMF blocked cardioprotection by ischemic preconditioning and diazoxide. Taken together, each of these results supports the conclusion that the ROS message is carried by a downstream product of hydroxyl radical (HO˙). The actions of BEL suggest a product of phospholipid oxidation.
RESEARCH DESIGN AND METHODS
Langendorff-perfused hearts.
Hearts from male Sprague-Dawley rats (200–240g) were perfused as previously described (15, 22, 37, 39) with Krebs-Henseleit buffer containing the following (in mM): 118 NaCl, 5.9 KCl, 1.75 CaCl2, 1.2 MgSO4, 0.5 EDTA, 25 NaHCO3, 16.7 glucose at 37°C and pH 7.4 and gassed with 95% O2-5% CO2. Treatment protocols are described in Fig. 3. Hearts were stabilized for 25 min with Krebs Henseleit buffer before treatment with buffer containing drugs or agents and then subjected to 25-min global ischemia followed by 2 h of reperfusion and measurement of infarct size. Ischemic preconditioning was established by two cycles of 5-min global ischemia followed by 5-min reperfusion before the index ischemia. Ischemic postconditioning was performed with six cycles of 10-s ischemia plus 10-s reperfusion (45). Hearts were not paced, and mechanical performance was evaluated as the product of heart rate and left ventricular developed pressure and reported as a percentage of rate pressure product at stabilization, t = 0. Infarct size was determined by the method of Ytrehus et al. (48) and reported as a percentage of slice cross section. Experimental protocols complied with the Guiding Principles in the Use and Care of Animals published by the National Institutes of Health and were approved by the Institutional Animal Care and Use Committee at Portland State University.
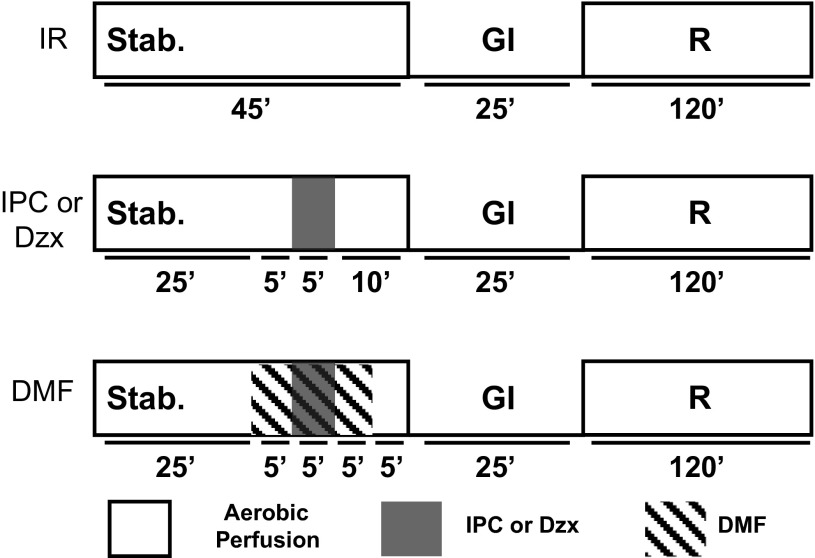
Isolated heart protocols. Hearts were perfused with buffer, as described in research design and methods. After 45 min of stabilization, hearts were subjected to 25 min global ischemia (GI), followed by 2 h of reperfusion (R) and, finally, processing for infarct size estimation. Protocol for ischemic reperfusion (IR) without additional treatment is labeled “IR.” Ischemic preconditioning (IPC) was established by 5-min global ischemia followed by 10-min reperfusion before the index ischemia. Diazoxide (Dzx; 50 μM) was perfused for 5 min, followed by 10-min reperfusion with buffer before the index ischemia. Dimethylformamide (DMF; 1% vol/vol) was administered 5 min before the first IPC ischemia and before Dzx; it was continued for 5 min after these treatments and this was followed by 5-min reperfusion with buffer before the index ischemia.
Mitochondrial isolation.
Male Sprague-Dawley rats (200–240 g) were anesthetized with CO2 and immediately decapitated, and the hearts were removed for mitochondrial isolation exactly as described previously (14). Hearts were washed and finely minced in ice-cold isolation buffer containing 250 mM sucrose, 10 mM HEPES at pH 7.2, and 0.5 mM K+-EGTA. The suspension was diluted threefold with isolation buffer supplemented with 1% fatty acid-free BSA. The time between decapitation and completion of homogenization was kept as brief as possible and was completed within 2 min; mitoKATP activity shows a sharp dependence on the length of this period. The suspension was homogenized with a motorized Teflon pestle and centrifuged for 3 min at 1,500 g. The supernatant was centrifuged for 5 min at 9,000 g, and the resulting pellets were resuspended in isolation buffer lacking BSA and centrifuged for 3 min at 2,300 g. This supernatant was centrifuged for 5 min at 9,000 g. The final mitochondrial pellet was resuspended with isolation buffer to 35–40 mg protein/ml and kept on ice. Where indicated, rat liver mitochondria were isolated using a similar protocol, as previously described (5). Mitochondrial protein concentration was estimated using the Biuret reaction (23).
Measurements of mitoKATP activity.
MitoKATP opening causes mitochondrial swelling due to respiration-driven uptake of K+ salts and water. These volume changes were followed by light scattering, as previously described (5, 11, 14, 20). Light-scattering changes of 0.1 mg/ml mitochondrial suspensions were followed at 520 nm and 30°C. Mitochondria were suspended in a buffered salt assay medium containing K+ salts of Cl− (120 mM), HEPES (10 mM), EGTA (0.1 mM), succinate (10 mM), MgCl2 (0.5 mM), phosphate (5 mM), ATP (200 μM), rotenone (2.5 μM), and oligomycin (1 μg/ml) at pH 7.2. Osmolality ranged between 275 and 280 mosmol/kgH2O. Data summarized in bar graphs as “mitoKATP activity (%)” were calculated from
where A−1(x) is the observed inverse absorbance at 120 s under the given experimental condition, and A−1 (ATP) and A−1(0) are values independently measured in the presence and absence of ATP, respectively.
Preincubation protocol.
A preincubation protocol was used to trigger activation of PKCε by endogenous ROS. As illustrated in Fig. 4, mitochondria were incubated in the light-scattering assay medium (described above) for 3 min at 30°C with 30 μM diazoxide. The preincubation medium was supplemented with various agents to explore the mechanisms of endogenous ROS signaling. Each sample was equally divided and preincubated in the presence or absence of diazoxide. The treated mitochondria were then isolated from the incubation medium and resuspended in a small volume (15 μl) of ice-cold sucrose buffer containing 250 mM sucrose and 10 mM HEPES at pH 7.2. MitoKATP activity of the treated mitochondria was then assayed using light scattering. When preincubated without diazoxide, the mitochondria behaved normally in the subsequent assay. That is, mitoKATP activity was inhibited by ATP, and inhibition was released by diazoxide. When preincubated with diazoxide, mitoKATP activity in the subsequent assay was not inhibited by ATP, and diazoxide had no effect. Thus, these conditions cause mitoKATP to remain open due to its phosphorylation by PKCε (13). Any agent that blocks any step in the process will block feedback activation of mitoKATP.
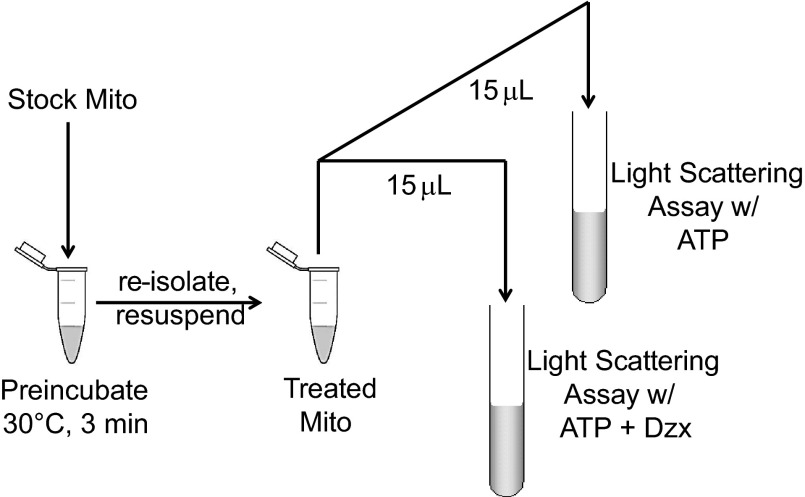
Preincubation protocol. Isolated rat heart mitochondria suspended in 250 mM sucrose and 10 mM HEPES at 30 mg/ml (Stock Mito) was incubated at 30°C for 3 min in assay medium containing 200 μM ATP and 30 μM Dzx, in a total volume of 0.5 ml. Treated mitochondria were reisolated and resuspended, and mitochondrial ATP-sensitive K+ channel (mitoKATP) activity was measured using the light-scattering assay, with a final protein concentration of 0.1 mg/ml. Half of the treated mitochondria were assayed with ATP and the other half with ATP and Dzx. Incubation was supplemented with various agents including 5-hydroxydecanoate (5-HD; 300 μM), εV1–2 (0.5 μM), catalase (250 U/ml), DMSO (1% vol/vol), DMF (1% vol/vol), Trolox (100 μM), deferoxamine (1 mM), N-2-mercaptopropionylglycine (MPG; 1 mM), or bromoenol lactone (BEL; 10 μM).
Measurements of MPT.
MPT activity was monitored by light scattering in medium containing 200 μM ATP and lacking EGTA. MPT opening was synchronized by sequential additions of CaCl2 (100 μM free Ca2+), ruthenium red (0.1 μM, to block further Ca2+ uptake), and carbonyl cyanide m-chlorophenyl hydrazine (250 nM), at 20, 40, and 60 s, respectively (12). These experiments used mitochondria from livers taken from the animals supplying the heart.
Statistical analysis.
Data are presented as means ± SD. One-way ANOVA followed by Tukey's post hoc test or Student's t-test of the means was used as required to analyze the data using Microcal Origin software (Northampton, MA). A value of P < 0.05 was considered statistically significant.
Chemicals.
Peroxynitrite was acquired from Cayman Chemical. All other chemicals were obtained from Sigma Chemical (St. Louis, MO). N-2-mercaptopropionylglycine (MPG) was always freshly prepared and adjusted to neutral pH by Tris base. Diazoxide and εV1–2 were prepared in DMSO, and BEL was always freshly prepared in DMSO. Uric acid, Trolox, deferoxamine, catalase, 5-hydroxydecanoate (5-HD), and ruthenium red were prepared in distilled water.
RESULTS
Endogenous ROS signaling and PKCε1-dependent feedback activation of mitoKATP.
Feedback activation of mitoKATP can be divided into three steps as described in Fig. 5. In step 1, K+ channel openers such as diazoxide open mitoKATP (21), and the resulting K+ influx and matrix alkalinization leads to increased generation of superoxide (1). Step 2 encompasses the ROS transformations that result in formation of the signaling ROS, i.e., the ROS that activates PKCε1. In step 3, the activated PKCε1 establishes the phosphorylation dependent open state of mitoKATP (13). The rationale for this division is that we can study the effects of agents on the overall cycle and, independently, on steps 1 and 3. In this way, we can identify agents that interfere specifically with formation of the signaling ROS (step 2).
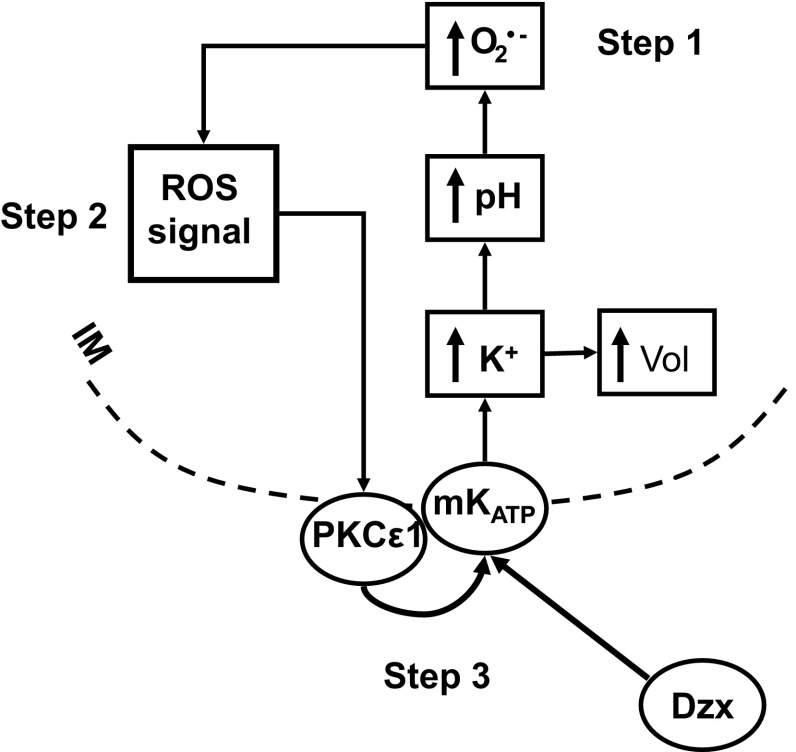
Reactive oxygen species (ROS)-dependent feedback activation of mitoKATP. Step 1 of the process encompasses the sequence from opening to superoxide (O2·−) formation. MitoKATP is opened by KATP channel openers such as Dzx (21). Consequent uptake of K+ and anions leads to increased matrix volume (↑Vol), which is the basis of the light-scattering assay for mitoKATP activity (14). The cytosolic concentration difference between [K+] and [phosphate] means that more K+ than phosphate will be taken up, leading to matrix alkalinization (↑pH; Ref. 14). Matrix alkalinization, in turn, inhibits complex I, leading to increased production of superoxide (O2·−; Ref. 1). Step 2 encompasses the many ROS transformations that take place and lead to the signaling ROS, which activates protein kinase-Cε1 (PKCε1). In step 3, the activated PKCε1 phosphorylates a protein, possibly mitoKATP itself, which leads to the phosphorylated open state of mitoKATP (13). In parallel (not represented here), the ROS signal activates PKCε2, leading to inhibition of mitochondrial permeability transition (MPT) opening (12).
Figure 6 contains results of control experiments using the preincubation protocol described in research design and methods. When mitochondria were preincubated with diazoxide and ATP, they exhibited maximum volume change in the normal assay medium containing ATP and no further effect was seen with the addition of diazoxide (1st 2 bars of Fig. 6). This result is characteristic of conditions that allow mitoKATP to achieve the phosphorylation-dependent open state. When step 1 was blocked by omission of diazoxide (2nd pair of bars) or inclusion of 5-HD (3rd pair of bars) in the preincubation, mitoKATP behaved as if there were no preincubation; that is, mitoKATP activity was inhibited by ATP and opened by diazoxide. When step 3 was blocked by inclusion of the specific PKCε inhibitor εV1–2 in the preincubation (last pair of bars), feedback activation was also prevented and mitoKATP responded normally to ATP and diazoxide.
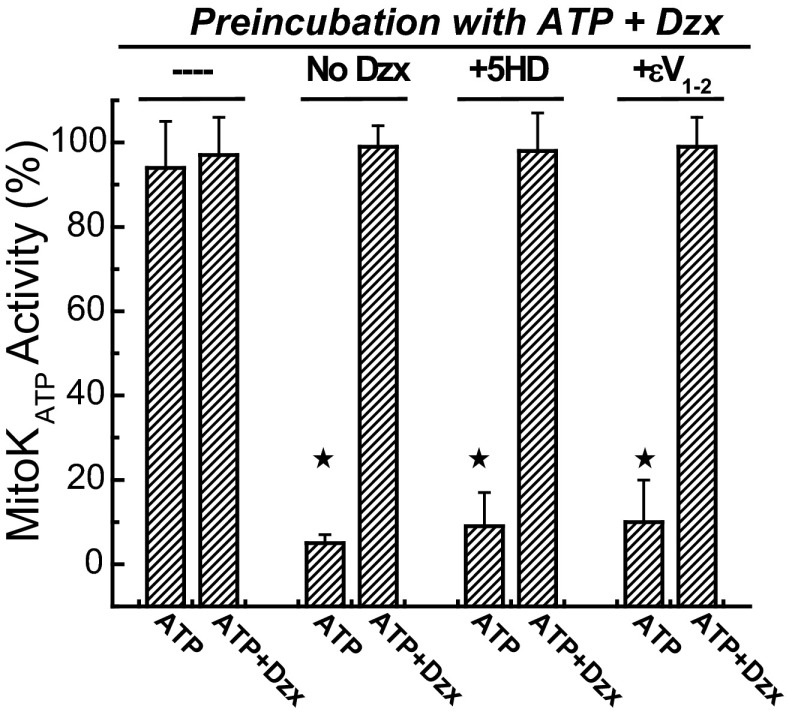
Feedback activation of mitoKATP is blocked by 5-HD and εV1–2. Shown are the effects of various agents on mitoKATP activity after preincubation with ATP (200 μM) plus Dzx (30 μM). Mitochondria were preincubated with the agents indicated at top and then assayed in K+ medium with ATP or ATP + Dzx as indicated below (described in research design and methods). With no further additions to the preincubation (-“—”), mitoKATP remains in the phosphorylated open state with full activity, and diazoxide (ATP + Dzx) has no further effect in the subsequent light-scattering assay. When mitoKATP opening during preincubation was prevented by omission of diazoxide (No Dzx) or inclusion of the mitoKATP blocker 5-HD (300 μM; +5-HD), the phosphorylation-dependent open state was blocked. When feedback activation of PKCε1 was prevented by inclusion of εV1–2 (0.5 μM; +εV1–2), the open state was also blocked. Data are means of mitoKATP activity ± SD of at least 3 independent experiments. P < 0.05.
Figure 7 contains results of preincubation experiments in which we evaluated agents that may block ROS signaling (Step 2) in the feedback loop shown in Fig. 5. DMSO, DMF, and MPG have all been described as reactants with HO˙, R (alkoxyl radicals), and RO
(alkylperoxyl radicals; Refs. 2, 32), each of which may play a role in endogenous ROS signaling. Trolox, a vitamin E analog, acts as a chain-breaking antioxidant and peroxyl radical scavenger (31). Figure 7A demonstrates that each of these agents prevented formation of the phosphorylation-dependent open state, suggesting that HO˙ is involved in the generation of the signaling ROS.
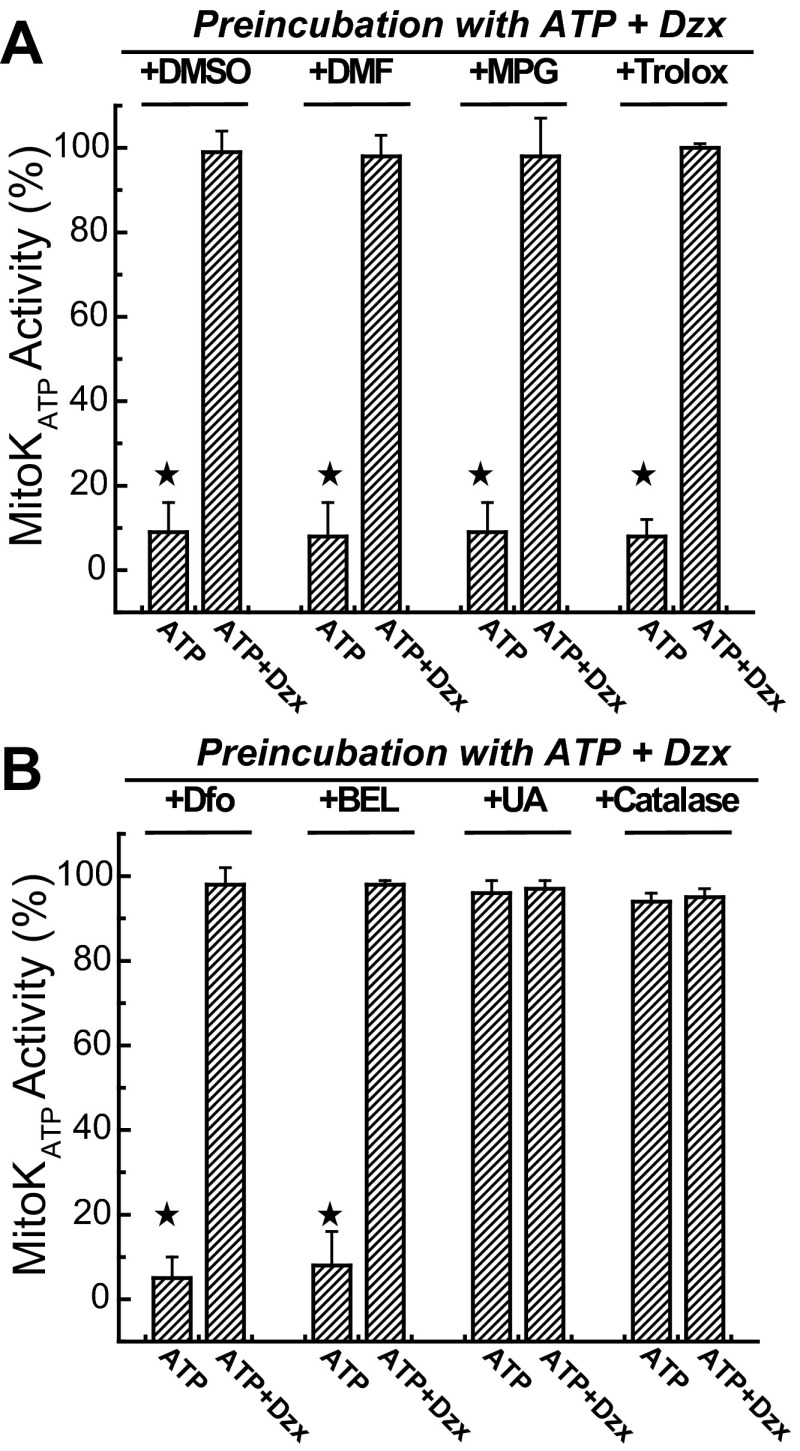
Effects of agents that may block formation of the signaling ROS on feedback activation of mitoKATP. A: effects of various ROS and radical scavenging agents and antioxidants on mitoKATP activity after preincubation with ATP plus Dzx in the presence of DMSO (1% vol/vol), DMF (1% vol/vol), MPG (1 mM), and Trolox (100 μM). Each of these agents blocked formation of the phosphorylation-dependent open state of mitoKATP. In B, deferoxamine (Dfo; 1 mM) and BEL (10 μM) also blocked this state, but uric acid (100 μM; +UA; peroxynitrite scavenger) and catalase (250 U/ml; H2O2 scavenger) did not interfere with feedback activation of mitoKATP. Mitochondria were preincubated with the agents indicated in the figure, then assayed in K+ medium, as described in research design and methods. Data are means of mitoKATP activity ± SD of at least 3 independent experiments. P < 0.05.
Deferoxamine is a chelator of iron (29, 41) and acts as a preventive antioxidant by reducing the rate of iron-dependent hydroxyl and peroxyl radical chain initiation. BEL (racemic bromoenol lactone) is an inhibitor of Ca2+-independent phospholipase (iPLA2; Ref. 8), and it prevents the release of oxidized phospholipids such as hydroperoxy fatty acids (FAOOH) from the bilayer, a possible downstream oxidation product in the reaction sequence. Figure 7B shows that both deferoxamine and BEL blocked feedback activation of mitoKATP, further suggesting that hydroxyl radicals and their downstream oxidation products are involved in the generation of the signaling ROS. When uric acid, a peroxynitrite scavenger (44), was included in the preincubation, the phosphorylation-dependent open state was preserved, indicating that peroxynitrite is not involved with any of the steps and, therefore, is not the signaling ROS. Catalase is an enzyme that catalyzes the decomposition of H2O2, and catalase was also unable to block this process, suggesting that H2O2 is not the signaling ROS.
The mechanisms of action of these agents suggests that they are acting on step 2, the formation of the ROS signal; however, it is necessary to demonstrate that they are not acting elsewhere in the loop, i.e., on step 1 or step 3. Accordingly, we tested each of the agents for their effect on mitoKATP activity in the straight light-scattering assay with no preincubation and found that none of them interfered with diazoxide opening of mitoKATP (data not shown). Note that this assay also detects respiratory inhibition or uncoupling, which inhibit K+ uptake by reducing the driving force.
To evaluate their effect on step 3 (induction of the phosphorylated open state by PKCε1), we examined whether the agents were able to block mitoKATP opening induced by H2O2, which works by activating PKCε1 (13). Figure 8 contains representative light-scattering traces using mitochondria without preincubation. Here, we find that Trolox had no effect. MPG did block H2O2-induced mitoKATP opening, which means that we cannot distinguish whether MPG acts on step 2 or step 3 from these experiments. The results of the step 3 assays are summarized in Fig. 9 and show that deferoxamine, DMSO, DMF, Trolox, and BEL did not inhibit H2O2-induced mitoKATP opening (step 3). Because they also did not interfere with diazoxide opening of mitoKATP (step 1), we infer that these agents are acting to prevent formation of the endogenous ROS signal that activates PKCε1 (step 2).
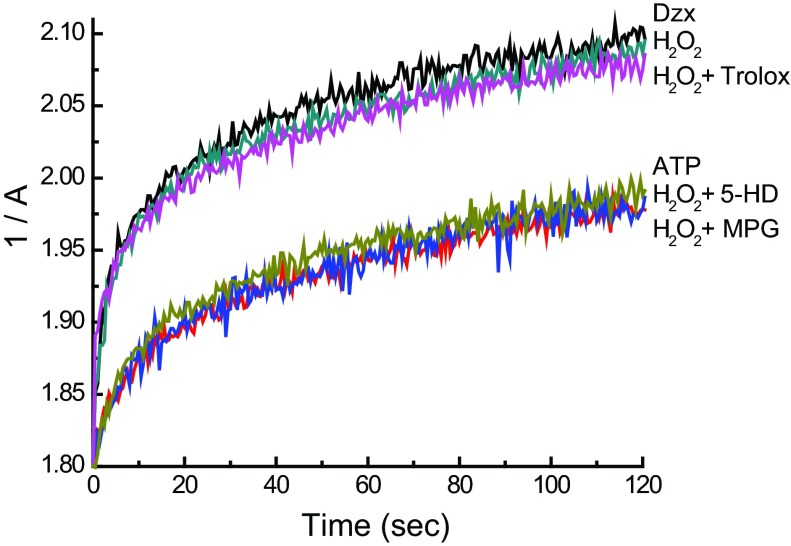
Differential effects of MPG and Trolox on H2O2-dependent mitoKATP opening. Shown are representative light-scattering traces (1/A vs. time) of rat heart mitochondria respiring on succinate in K+ medium. Mitochondria were suspended at 0.1 mg/ml and assayed as described in research design and methods. ATP (200 μM) was present in all experiments. Where indicated, Trolox, MPG, 5-HD, or no additions (ATP) were present in the assay medium prior to the addition of mitochondria. Mitochondrial swelling was initiated by the addition of hydrogen peroxide (H2O2; 2 μM) or Dzx 2 s after the addition of mitochondria to trigger PKCε1-dependent mitoKATP opening.
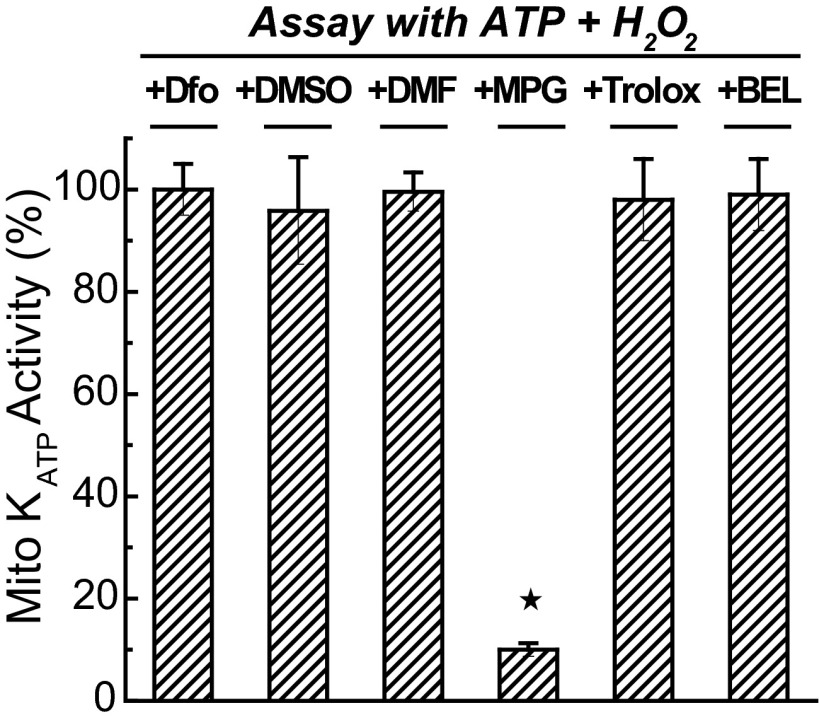
Effects of agents on activation of PKCε1 by H2O2. Agents that were utilized in the preincubation experiments of Fig. 6 were examined for their effects on H2O2-induced PKCε-dependent mitoKATP opening in the straight light-scattering assay described in research design and methods. MPG was the only agent that interfered with this step in the process. Deferoxamine, DMSO, DMF, Trolox, and BEL had no effect on H2O2 activation. Data are means of mitoKATP activity ± SD of at least 3 independent experiments. P < 0.05.
These findings may be summarized as follows. 1) Deferoxamine, DMSO, DMF, Trolox, and BEL each prevent formation of the endogenous ROS signal. These results imply that the ROS signal arises downstream from O. 2) H2O2 is produced upstream of O
, and catalase, the H2O2 scavenger, had no effect on the formation of the phosphorylation-dependent open state. Therefore, H2O2 can be excluded as the signaling ROS. 3) The action of MPG is independent of ROS scavenging. Like all thiols, MPG can scavenge O
, but O
is not involved in the pathway responsible for the results in Fig. 8. Moreover, MPG has little or no H2O2 scavenging ability (6, 30). We propose that this action of MPG is due to its strong thiol reductant properties, holding PKCε in a reduced state and thereby preventing its activation.
Cardioprotection by ischemic preconditioning and diazoxide is blocked by DMF.
The implication of the previous mitoKATP experiments is that intercepting the HO˙-dependent reactions will block all modes of ROS-dependent cardioprotection. To examine this question, we perfused the heart with 1% DMF, the HO˙-scavenger, following the protocols described in Fig. 3. As demonstrated in Fig. 10, DMF inhibited the improvement in postischemic functional recovery (Fig. 10A) and blocked the infarct-size reduction (Fig. 10B) normally observed with ischemic preconditioning and diazoxide. DMF alone had no effect on infarct size in these conditions (Fig. 10B). Again, the effect of DMF on cardioprotective signaling implicates a downstream oxidation product of HO˙ as the signaling ROS.
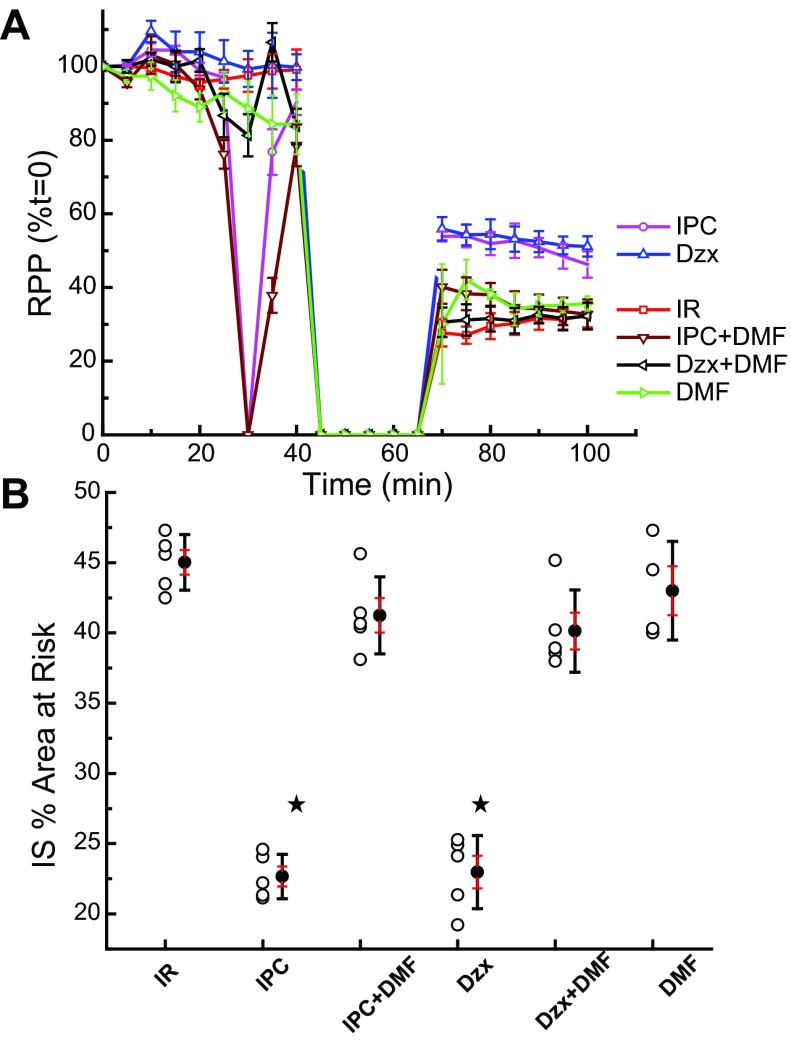
DMF prevents protection of the perfused heart by Dzx and IPC. A: shown are measurements of rate-pressure product [RPP (%t = 0)] with time from perfusion of the ex vivo heart. Dzx (50 μM) and IPC improved functional recovery, and this effect was blocked by dimethylformamide (Dzx + DMF and IPC + DMF), a free radical scavenger. DMF alone had no effect. B: infarct size as a percent of area at risk (IS % Area at Risk) is plotted for the various treatments. Treatment with Dzx and IPC reduced infarct size, and this effect was blocked by DMF. Data are means of IS %area at risk ± SD of at least 3 independent experiments. P < 0.05.
Endogenous ROS signaling and mitoKATP-dependent MPT inhibition.
MPT synchronization, described in research design and methods, was employed to determine the role of HO˙, R, and/or RO
in diazoxide induced MPT inhibition, which depends on ROS activation of a second mitochondrial PKCε, PKCε2 (12). Results of a representative experiment are contained in Fig. 11. CsA completely blocked MPT activity, and diazoxide inhibited MPT opening by ~60%, which is the customary extent observed in these experiments (13). This inhibition was reversed by 5-HD. MPG, DMSO, and DMF (not shown) also reversed MPT inhibition by diazoxide. These results also imply that the signaling ROS that activates PKCε2 must be a downstream oxidation product of HO˙.
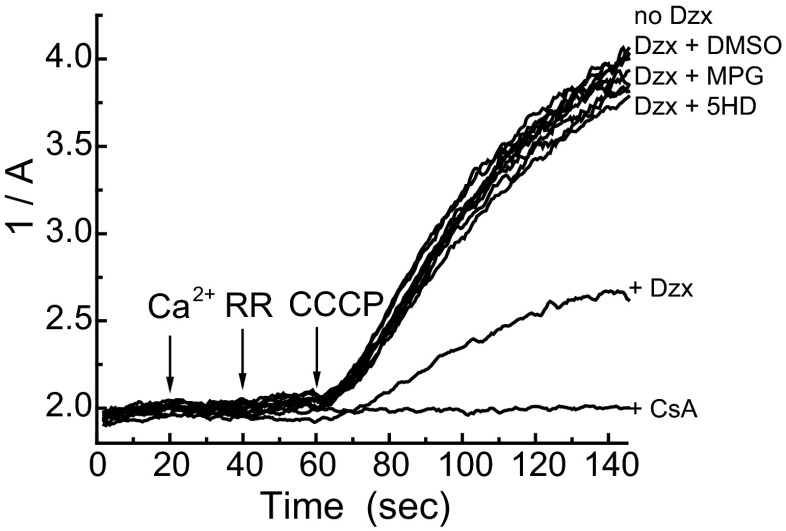
Effects of free radical reactants on mitoKATP-dependent MPT inhibition. Shown are light-scattering traces (1/A) of rat liver mitochondria respiring on succinate in K+ medium and assayed as described in research design and methods. Free calcium (100 μM) (Ca2+), ruthenium red (RR; 0.1 μM), and carbonyl cyanide m-chlorophenyl hydrazone (CCCP; 0.25 μM) were added sequentially at 20-s intervals, as shown. MPT inhibition by Dzx (30 μM) was blocked by 5-HD (300 μM; mitoKATP blocker), MPG (1 mM; thiol reductant), and DMSO (1%, 14 M; HO˙/R reactant). Data presented are representative of 3 independent experiments with P < 0.05.
DISCUSSION
The major findings of the present study are 1) that the signaling ROS responsible for activating mitochondrial PKCεs is a downstream oxidation product of hydroxyl radical, not superoxide or H2O2; and 2) that the main action of submillimolar MPG on mitochondria and perfused heart is inactivation of PKCε by thiol reduction rather than ROS scavenging.
MitoKATP governs the ROS signaling that is essential for cardioprotection (18). MitoKATP opening has been found to increase levels of ROS in perfused heart (36), in cardiomyocytes (35), and in the mitochondrial matrix (1). The ionophore valinomycin, at a concentration yielding the same K+ flux as diazoxide, caused the same ROS increase as diazoxide, so the mitoKATP-dependent increase in ROS is due specifically to K+ influx into the matrix (1). The primary mitochondrial targets of ROS signaling are PKCε1, which activates mitoKATP, and PKCε2, which inhibits MPT (reviewed in Ref. 19). Mitochondrial PKCε1 and mitoKATP copurify and coreconstitute to form a functioning signaling module in proteoliposomes (25). Thus mitoKATP in either mitochondria or proteoliposomes can be opened by all PKCε activators, including phorbol esters, the specific peptide activator, ΨεRACK, and a variety of thiol oxidizing agents.
Our objective is to identify the mitoKATP-dependent endogenous ROS that activates PKCε1 to leave mitoKATP in a phosphorylated open state. Superoxide is the first ROS that increases due to increased K+ uptake into the matrix (see Fig. 1) and has been proposed to be the ROS signal (38). Xanthine/xanthine oxidase (X/XO) induced mitoKATP opening in isolated mitochondria; however, superoxide rapidly dismutates to H2O2 in aqueous solution, and Costa et and Garlid (13) showed that X/XO did not open mitoKATP in the presence of catalase. Therefore, the observed effect of X/XO was mediated by H2O2, and superoxide can be excluded as a PKCε activator or mitoKATP opener. Queliconi et al. (38) repeated the X/XO experiment and obtained the same results; however, they failed to control for H2O2 production and erroneously concluded that superoxide opens mitoKATP. PKCε is activated by nitric oxide (NO; Ref. 4) and H2O2 (28), which oxidize the thiols in the zinc finger of PKCε, leading to its activation. Consistent with this biochemistry, H2O2 and NO cause mitoKATP opening (13, 25) that is blocked by the PKCε peptide inhibitor εV1–2. The absence of an effect of H2O2 and NO in the presence of εV1–2 demonstrates that these agents have no direct effect on mitoKATP. Again, Queliconi et al. (38) repeated these experiments and obtained the same result; however, they failed to control for PKCε involvement and erroneously concluded that H2O2 and NO open mitoKATP directly.
Liu et al. (30) concluded that hydroxyl radical (HO˙) is responsible for ROS signaling in the heart, but this conclusion was based on experiments showing that MPG blocks ROS signaling and the assumption that this block was due to HO˙ scavenging. This assumption may be incorrect. The data in Fig. 8 show that MPG blocks H2O2-induced mitoKATP opening. HO˙ is not involved in this process, and MPG has little or no H2O2 scavenging ability (6, 30). Moreover, eliminating HO˙ requires very high concentrations of scavenger due to the transitory nature of HO˙, and it is unlikely that submillimolar concentrations of MPG would be effective. Thus most scavengers “will never be present at levels remotely approaching those of endogenous molecules that react at diffusion-controlled rates with HO˙” (24). Therefore, we infer that MPG prevents signaling, not by scavenging ROS, but rather by virtue of its powerful thiol reduction properties, keeping PKCε in a reduced, inactive state. Conclusions drawn from experiments in which MPG blocks ROS signaling, including those of Liu et al. (30), should be reevaluated in view of this new finding related to the mechanism of action of MPG.
To investigate the location giving rise to the endogenous ROS signal, we employed the preincubation protocol described in research design and methods and results. This protocol leads to feedback activation of mitoKATP, which depends on endogenous ROS signaling to PKCε1 (13). The results of Figs. 4–7 provide important clues to the identity of the ROS messenger. They are best understood in the context of Figs. 1 and and2.2. The antioxidants deferoxamine and Trolox each prevented mitoKATP opening. Deferoxamine is an iron chelator, and Trolox, a water soluble derivative of vitamin E, is a peroxyl radical scavenger and lipid peroxidation chain-breaking antioxidant. These data indicate that the redox-sensitive opening of mitoKATP is dependent on both iron and peroxyl radicals and are consistent with transition metal-catalyzed RO-dependent signaling. These steps occur downstream of HO˙ production and strongly suggest that HO˙ is the precursor of the signaling molecule. This is supported by the finding that inclusion of DMSO or DMF, which react with HO˙, R
, and/or RO
(2), in the preincubation prevented mitoKATP opening.
Many workers have proposed that H2O2 is the messenger of ROS-dependent mitoKATP opening (1, 46). H2O2 would appear to be a good candidate, not the least because it is effective in activating mitochondrial PKCε (11, 13, 25, 50) and in protecting ischemic heart (47, 49) and hypoxic cardiomyocytes (16). However, a major finding of the present study is that H2O2 can be excluded as the signaling ROS in mitochondria and that the signaling ROS originates downstream of hydroxyl radical. The evidence is 1) the ROS signal arises downstream from O radical, whereas H2O2 is produced upstream of O
radical (Fig. 1); and 2) catalase, the H2O2 scavenger, had no effect on the formation of the phosphorylation-dependent open state, whereas DMF and DMSO, which have neither H2O2 scavenging nor thiol reductant properties, do block PKCε-dependent mitoKATP activation. Circumstantial evidence also argues against H2O2 as the signaling ROS: H2O2 is produced in the matrix, whereas PKCε1 faces the intermembrane space. The matrix of heart mitochondria contains glutathione, thioredoxin reductase, and catalase, which may prevent sufficient H2O2 from reaching its target (42).
The conclusion that the ROS message is formed downstream from HO˙ is further supported by the effects of DMSO and DMF on endogenous ROS-dependent MPT inhibition (Fig. 10). Finally, the finding that DMF prevents cardioprotection by both ischemic preconditioning and diazoxide (Fig. 11) supports extension of the conclusion to the ex vivo and in vivo heart. That HO˙ is necessary for cardioprotective signaling should not be taken to mean that HO˙ itself is the signaling ROS. HO˙ is too reactive for signaling, with a half-life that is diffusion limited at ~1 ns (24). HO˙ will react with its nearest neighbors almost immediately after it is formed, allowing for the formation of downstream oxidation products that are most likely responsible for signaling.
Finally, the finding that BEL interrupted the ROS signal (Fig. 7) implicates hydroperoxy fatty acids (FAOOH) as the ROS signal. A full investigation of the effects of fatty acids and hydroperoxy fatty acids on mitoKATP and on ischemia-reperfusion injury will be presented in a subsequent communication.
Limitations.
1) The conclusions are based largely on in vitro experiments carried out on isolated mitochondria. The rationale for this approach is that the signaling ROS not only originates in mitochondria but also acts on mitochondria. We have no reason to believe that the ROS transformation reaction sequence is any different in vivo. 2) We stress that the conclusions relate specifically to ROS signaling secondary to mitoKATP opening. The reverse sequence, mitoKATP opening caused by ROS, may also be physiologically important. For example, it is possible that the increased ROS that occurs during ischemia opens mitoKATP, and this ROS may arise from a different location, such as complex III. The identity of the ROS signal in this setting has not yet been addressed. 3) BEL has been shown to inhibit activation of store-activated Ca2+ channels; however, this is mediated by inhibition of iPLA2 and is therefore a pharmacological effect (40). It has recently been shown that BEL inhibits voltage-gated Ca2+ and transient receptor potential canonical channels independently of iPLA2 (9). We consider it unlikely that these effects play a role in the effect of BEL on isolated mitochondria.
Conclusions and potential conceptual/pragmatic values of these findings.
We conclude that the signaling ROS responsible for activating mitochondrial PKCεs is a downstream oxidation product of hydroxyl radical and that superoxide and H2O2 are not the signaling ROS. We further conclude that the main action of submillimolar MPG on mitochondria and perfused heart is not ROS scavenging but rather thiol reduction, causing inactivation of PKCε and other ROS-dependent kinases. MitoKATP-dependent ROS signaling is a central process in cardioprotection and cellular signaling generally. Determining the identity of the mitochondrial signaling ROS is important for future research in these areas.
GRANTS
This work was supported by National Heart, Lung, and Blood Institute Grants HL-35673; and HL-067842 (to K. D. Garlid) and Czech Ministry of Education Grant ME-09018 (to M. Jaburek).
DISCLOSURES
No conflicts of interest, financial or otherwise, are declared by the author(s).
AUTHOR CONTRIBUTIONS
Author contributions: A.O.G., M.J., and K.D.G. conception and design of research; A.O.G., M.J., and J.P.J. performed experiments; A.O.G., M.J., and J.P.J. analyzed data; A.O.G., M.J., J.P.J., and K.D.G. interpreted results of experiments; A.O.G., M.J., J.P.J., and K.D.G. prepared figures; A.O.G., M.J., and K.D.G. drafted manuscript; A.O.G., M.J., and K.D.G. edited and revised manuscript; A.O.G., M.J., J.P.J., and K.D.G. approved final version of manuscript.
ACKNOWLEDGMENTS
We express gratitude for the excellent technical and administrative assistance of Craig Semrad, Caitlin E. Pesout, and Beau Sober.
REFERENCES
Articles from American Journal of Physiology - Heart and Circulatory Physiology are provided here courtesy of American Physiological Society
Full text links
Read article at publisher's site: https://doi.org/10.1152/ajpheart.00858.2012
Read article for free, from open access legal sources, via Unpaywall:
https://europepmc.org/articles/pmc3798754
Citations & impact
Impact metrics
Article citations
The multifaceted role of mitochondria in cardiac function: insights and approaches.
Cell Commun Signal, 22(1):525, 29 Oct 2024
Cited by: 0 articles | PMID: 39472951 | PMCID: PMC11523909
Review Free full text in Europe PMC
Mitochondrial Volume Regulation and Swelling Mechanisms in Cardiomyocytes.
Antioxidants (Basel), 12(8):1517, 28 Jul 2023
Cited by: 5 articles | PMID: 37627512 | PMCID: PMC10451443
Review Free full text in Europe PMC
Mitochondrial Cristae Morphology Reflecting Metabolism, Superoxide Formation, Redox Homeostasis, and Pathology.
Antioxid Redox Signal, 39(10-12):635-683, 11 Apr 2023
Cited by: 15 articles | PMID: 36793196 | PMCID: PMC10615093
Review Free full text in Europe PMC
Specific Features of Mitochondrial Dysfunction under Conditions of Ferroptosis Induced by t-Butylhydroperoxide and Iron: Protective Role of the Inhibitors of Lipid Peroxidation and Mitochondrial Permeability Transition Pore Opening.
Membranes (Basel), 13(4):372, 24 Mar 2023
Cited by: 2 articles | PMID: 37103799 | PMCID: PMC10145271
Endothelial Function and Hypoxic-Hyperoxic Preconditioning in Coronary Surgery with a Cardiopulmonary Bypass: Randomized Clinical Trial.
Biomedicines, 11(4):1044, 28 Mar 2023
Cited by: 0 articles | PMID: 37189663 | PMCID: PMC10136033
Go to all (37) article citations
Similar Articles
To arrive at the top five similar articles we use a word-weighted algorithm to compare words from the Title and Abstract of each citation.
Intramitochondrial signaling: interactions among mitoKATP, PKCepsilon, ROS, and MPT.
Am J Physiol Heart Circ Physiol, 295(2):H874-82, 27 Jun 2008
Cited by: 141 articles | PMID: 18586884 | PMCID: PMC2519212
Ischemic preconditioning requires increases in reactive oxygen release independent of mitochondrial K+ channel activity.
Free Radic Biol Med, 40(3):469-479, 09 Nov 2005
Cited by: 37 articles | PMID: 16443162
GSK-3β at the crossroads in the signalling of heart preconditioning: implication of mTOR and Wnt pathways.
Cardiovasc Res, 90(1):49-56, 13 Jan 2011
Cited by: 76 articles | PMID: 21233250
Mitochondria "THE" target of myocardial conditioning.
Am J Physiol Heart Circ Physiol, 315(5):H1215-H1231, 13 Jul 2018
Cited by: 48 articles | PMID: 30004243
Review
Funding
Funders who supported this work.
NHLBI NIH HHS (2)
Grant ID: HL-067842
Grant ID: HL-35673