Abstract
Background
p53 accumulation in head and neck squamous cell carcinoma (HNSCC) cells creates a targetable tumor antigen. Adjuvant dendritic cell (DC)-based vaccination against p53 was tested in a phase I clinical trial.Experimental methods
Monocyte-derived DC from 16 patients were loaded with two modified HLA-class I p53 peptides (Arm 1), additional Th tetanus toxoid peptide (Arm 2), or additional Th wild-type (wt) p53-specific peptide (Arm 3). Vaccine DCs (vDC) were delivered to inguinal lymph nodes at three time points. vDC phenotype, circulating p53-specific T cells, and regulatory T cells (Treg) were serially monitored by flow cytometry and cytokine production by Luminex. vDC properties were compared with those of DC1 generated with an alternative maturation regimen.Results
No grade II-IV adverse events were observed. Two-year disease-free survival of 88% was favorable. p53-specific T-cell frequencies were increased postvaccination in 11 of 16 patients (69%), with IFN-γ secretion detected in four of 16 patients. Treg frequencies were consistently decreased (P = 0.006) relative to prevaccination values. The phenotype and function of DC1 were improved relative to vDC.Conclusion
Adjuvant p53-specific vaccination of patients with HNSCC was safe and associated with promising clinical outcome, decreased Treg levels, and modest vaccine-specific immunity. HNSCC patients' DC required stronger maturation stimuli to reverse immune suppression and improve vaccine efficacy.Free full text

Phase I dendritic cell p53 peptide vaccine for head and neck cancer
Abstract
Background
p53 accumulation in head and neck squamous cell carcinoma (HNSCC) cells creates a targetable tumor antigen. Adjuvant dendritic cell (DC)-based vaccination against p53 was tested in a phase I clinical trial.
Methods
Monocyte-derived DC from 16 patients were loaded with two modified HLA-class I p53 peptides (Arm 1); additional T-helper(Th) tetanus toxoid peptide (Arm 2) or additional Th wt p53-specific peptide (Arm 3). Vaccine DC (vDC) were delivered to inguinal lymph nodes at 3 time points. Vaccine (vDC) phenotype, circulating p53-specific T-cells and regulatory T-cells (Treg) were serially monitored by flow cytometry and cytokine production by Luminex. vDC properties were compared to those of DC1 generated with an alternative maturation regimen.
Results
No grade II-IV adverse events were observed. Two-year disease-free survival (DFS) of 88% was favorable. p53-specific T-cell frequencies were increased post vaccination in 11/16 patients (69%), with IFN-γ secretion detected in 4/16 patients. Treg frequencies were consistently decreased (p=0.006) relative to pre-vaccination values. The phenotype and function of DC1 were improved relative to vDC.
Conclusion
Adjuvant p53-specific vaccination of HNSCC patients was safe and associated with promising clinical outcome, decreased Treg levels, and modest vaccine-specific immunity. HNSCC patients’ DC required stronger maturation stimuli to reverse immune suppression and improve vaccine efficacy.
Introduction
Despite considerable recent advances in therapy of HNSCC, survival has not improved >50% for decades, largely due to tumor recurrence, high frequency of second primary tumors, development of distant metastases and resistance of the tumor to chemo-radiotherapy (1). Novel therapeutic strategies are needed, and tumor antigen-specific vaccination has been perceived as a potentially effective approach to improve outcome by mobilizing anti-tumor immunity and reversing immune escape in HNSCC patients. Immune suppressive mechanisms described in these patients are extensive and include impaired dendritic cell (DC) maturation (2, 3), inefficient antigen presentation (4), and an increased frequency as well as suppressive activity of regulatory T cells (Treg) (5). As a result of HNSCC-induced immune suppression, anti-tumor responses of patients are compromised, and a reversal or removal of immune suppression would be expected to restore these responses. Consequently, anti-tumor vaccines offer an opportunity for normalization of immune responses and of the immune balance responsible for the control of tumor progression.
In all anti-tumor vaccines, the selection of a target antigen is one of the most critical issues. The requirements for the selection of a tumor associated antigen (TA) to be used in the vaccine have been defined (6). Such a TA should be selectively expressed in the tumor cells but not normal tissues, naturally processed and presented by APC and immunogenic, i.e., able to induce TA-specific effector and helper T cells. Based on previous studies, p53 meets these criteria: it is the most frequently mutated gene in HNSCC (7) and the mutated protein accumulates in most HNSCC cells (8), allowing for overexpression and processing of wt p53 peptides (8–11). We have previously demonstrated that the presentation of wt p53 peptides on DC induced peptide-specific immune responses in vitro in normal donors and in HNSCC patients (8, 12–14). Similar in vivo studies with wt p53-pulsed DC in animal tumor models have also led to the development of anti-tumor immunity and inhibited tumor growth (11). Also, DC-based wt p53 peptide vaccines have been used for immunotherapy of human ovarian, breast, lung and colon cancers (15).
Here, we report the results of a phase Ib trial of autologous monocyte-derived DC loaded with selected wt p53 peptides. Although the study follows the design used for other wt p53-based DC vaccines (15, 16), it includes several novel aspects. First, to determine whether the addition of T helper (Th) peptides to the vaccine results in the improvements of TA-specific anti-tumor immunity, we included the HLA class II p53 helper peptide (p53110-124 ref. (17)) or tetanus-derived memory peptide (18) in separate vaccine arms. Second, we used two altered (optimized) HLAA*0201 binding p53 peptides (p53149-157 T150L variant, ref. (9) and p53246-272 F270W variant, ref. (19)) to enhance the peptide binding to TCR or to HLA-A2.1 with higher avidity, respectively. Third, serial immune monitoring of all vaccinated patients allowed for the consideration of TA-specific immunity generated in response to the vaccine in the context of clinical outcome. Finally, in vitro generation of DC from the patients’ monocytes in parallel, comparing properties of DC used in the vaccine (vDC) with an alternative maturation protocol (DC1, (20, 21)), allowed for the identification of improvements in the DC maturation that can boost their adjuvant activity in future trials. The study provides novel insights into optimization of the production and monitoring of adjuvant DC-based vaccines for patients with HNSCC and other p53-overexpressing epithelial malignancies.
Materials and Methods
Patient cohort
16 HLA-A2.1+ patients with advanced HNSCC were enrolled in this study. Six patients were treated with surgery alone and 10 patients had received adjuvant chemo-radiotherapy (CRT) after surgery. At the time of vaccination, all patients were free of disease. The patients’ clinical and demographic data are listed in Table I. All patients were treated at the University of Pittsburgh Cancer Institute and signed written informed consent to participate in this trial, which was approved by the Institutional Review Board of the University of Pittsburgh (UPCI 03-156; IRB# 0507062, NCT00798655), under FDA IND #12273 (sponsor RLF).
Table I
Clinical and demographic data.
ID | Arm | Age | TNM | Location | Status (months) |
---|---|---|---|---|---|
1 | 1 | 78 | T2 N2c | base of tongue | alive (30) |
2 | 1 | 66 | T3 N1 | oropharynx | alive (29) |
3 | 1 | 55 | T3 N2b | base of tongue (r) | alive (41) |
4 | 1 | 27 | T2 N1 | base of tongue | alive (06) |
5 | 1 | 58 | T2 N2b | lateral tongue | DOD (17) |
6 | 1 | 55 | T4 N2b | base of tongue (r) | alive (26) |
| |||||
7 | 2 | 68 | T4 N2b | hypopharynx | alive (27) |
8 | 2 | 60 | T1 N0 | tonsil | alive (42) |
9 | 2 | 42 | Tx N2a | CUP | alive (38) |
10 | 2 | 57 | Tx N2a | CUP | alive (39) |
| |||||
11 | 3 | 57 | T4 N2c | pan-pharynx | DOD (25) |
12 | 3 | 56 | Tx N2a | CUP | alive (26) |
13 | 3 | 77 | T3 N0 | maxilla (r) | DOD (16) |
14 | 3 | 59 | T4 N2b | base of tongue | alive (39) |
15 | 3 | 62 | T2 N0 | hypopharynx | alive (33) |
16 | 3 | 54 | T4 N2b | larynx | alive (29) |
CUP – cancer of unknown primary, DOD – death of disease (r) recurrent disease before vaccination
Immunohistochemistry of tumors for p53
Staining was performed with p53 monoclonal primary antibody (D011, 1:400 dilution) and secondary antibody ‘Dual Envision Polymer,’ using Antigen Retrieval-Citrate pH6 buffer (Dako, USA) and DAB chromogen (Dbiosys, CA, USA). p53 protein accumulation was scored as positive when >10% of tumor cells were stained positive as observed by the study pathologist.
Generation of DC
Monocytes were isolated from autologous leukapheresis products by the Elutra System (Caridian BCT, CO). The monocyte-rich fraction #5 was collected and analyzed for cell viability, cell counts and the cell phenotype by flow cytometry. Following 6-day culture of separated monocytes in Cellgenix DC medium containing GM-CFS (1,000IU/mL) and IL-4 (10ng/mL, both Cellgenix, Germany), immature DC (iDC) were harvested and counted. Aliquots containing 10,000 harvested iDC were re-plated in T-75 culture flasks in medium containing GM-CFS and IL-4 as above, and matured by the addition of a pro-inflammatory cytokine cocktail for 48h.
DC used for vaccination of patients (vDC) were matured in a cytokine cocktail containing IL-1β (10ng/mL), IL-6 (10ng/mL), TNF-α (10ng/mL) and PGE2 (1ug/mL), The maturation cytokine cocktail for DC1, which were generated in parallel with vDC but were not used for vaccination, contained IL-1β (10ng/mL), TNF-α (50ng/mL), IFN-α (3,000IU/mL), IFN-γ (1,000IU/mL) and Poly I:C (20ug/mL). Supernatants of both DC preparations were collected for cytokine measurements (IL-10, IL-12, VEGF) by Luminex™ according to the manufacturer’s protocol (SinglePlex Bead Kit, Invitrogen). DC1 were used for in vitro studies only. Cytokines were purchased from the following manufacturers: IL-1β, IL-4, IL-6, and TNF-α (R&D Systems, Minneapolis, MN); IFN-α (Schering, Kenilworth, NJ); IFN-γ (Intermune, Brisbane, CA); GM-CSF (Immunex, Seattle, WA); PGE2 and Poly I:C (Sigma-Aldrich, St. Louis, MO). All DC cultures were tested negative for endotoxin and mycoplasma contamination.
Patient randomization and administration of vaccines
Patients were randomized into three treatment arms as shown in Table II (supplementary data) and Figure S1. The following peptides were used for DC loading: modified HLA-A2 binding wt p53149-157 [S(T->L)PPPGTRV], with T150L substitution, ref. (9) and wt p53264-272 [LLGRNS(F->W)EV, with F270W substitution (22). In Arm 2, the modified, pan-DR binding T-helper epitope from tetanus toxoid (AQYIKANSKFIGIGL, (18, 23–25)) was used. HLA DR4− patients were randomized to Arm 1 (no helper peptide) or Arm 2 (tetanus helper peptide). All HLA-DR4+ patients were assigned to Arm 3 due to DR4+ restriction of the Th p53110-124 peptide sequence (26).
Fresh or cryopreserved mDC were loaded with the respective combinations of class I / class II peptides (10uM each) for 18h. Autologous DC (100 uL) were injected into an inguinal lymph node (LN) under ultrasound guidance biweekly for three time points. The vaccination schedule and the injection site were selected based on previously reported successful experience at our institution (27, 28). LN in the head and neck region were avoided, as they could have been affected by the proximity of tumor cells.
Collection of peripheral blood mononuclear cells
Blood samples of study patients (40 – 50mL) were collected at the following time points: (a) baseline; (b) one day before the second vaccination; (c) one week and (d) one month after the third vaccination. Blood was drawn into heparinized tubes and centrifuged on Ficoll-Hypaque gradients (GE Healthcare Bioscience, UK). Peripheral blood mononuclear cells (PBMC) were recovered, washed and used for experiments or cryopreserved in RPMI medium (Invitrogen) containing fetal bovine serum (FBS, 40%) and DMSO (10%).
Antibodies and flow cytometry
The following anti-human monoclonal antibodies (mAb) were used for staining CD3-FITC, CD4-PC5, CD8-PC5, CD14-ECD, CD80-FITC, CD86-PE, DR-ECD, CD83-PC5, CD1a-PC5 (Beckman Coulter), HLA-A2-PE (Biolegend), CCR7-FITC (R&D Systems), CD39-FITC, (eBioscience), CD25-PE (Miltenyi), including their respective isotypes, which served as negative controls for surface as well as intracellular staining. All Abs were pre-titrated using PBMC to determine the optimal staining dilution for each. For HLA-typing and determination of APM components we used BB7.2 δ-specific mAb SY-4, LMP 2-specific mAb SY-1, TAP1-specific mAb, TAP2-specific mAb NOB-2, tapasin-specific mAb TO-3 which were generated by Dr. Soldano Ferrone (Harvard U) and generously donated for these studies (3).
Cryopreserved cells were thawed and washed in RPMI medium. For flow cytometry, cells were incubated with mAbs specific for each surface marker in 50μL PBS for 30 min at RT. Flow cytometry was performed using an EPICS XL-MCL flow cytometer equipped with Expo32 software (all Beckman Coulter). The acquisition and analysis gates were restricted to the lymphocyte gate based on characteristic properties of the cells in the forward and side scatter. At least 1 × 105 events were acquired for analysis and gates were restricted to the CD4+ and CD8+ T cell subsets.
The frequency of regulatory T cells (Treg) was measured by multicolor flow cytometry gating on CD4+CD25+CD39+ T cells as previously described (29). The absolute numbers of Treg were calculated using total white cell blood counts determined for each patient. Normal control (NC) values for the frequency and absolute Treg numbers were available in our laboratory having been determined previously.
P53 peptides and measurement of p53-specific CTL
The frequency of CTL specific for wt p53 epitopes was determined by tetramer flow cytometry. Tetramers specific for the wt p53149-157 sequences (STPPPGTRV) and wt p53264-272 sequences (LLGRNSFEV) were obtained from the NIH tetramer facility (Atlanta, GA). The specificity of the p53 tetramers was confirmed by staining against a CTL line specific for p53 as well as irrelevant CTL. The gate for tetramer+ events was defined by PBMC stained with antibodies (CD3/CD8/CD14) in the absence of tetramer, and the lower limit of detection (LLD) for p53 peptide-specific tetramers was established at the reciprocal frequency of 1/7,805 (0.013%) by staining T cells of ten HLA-A2.1neg donors (13, 30). Approximately 1 × 106 events were acquired, including >50,000 gated CD8+ T cells, and positive events were defined as CD3+CD8+CD14negtetramer+.
ELISPOT Assays
Reactivity of PBMC against p53-peptides was tested by IFN-γ ELISPOT assay as previously described (8). Patients’ PBMC (1×105/well) were stimulated using T2 cells loaded with wt p53 peptides or tetanus peptide for 18h, in vitro. All experiments used negative and positive controls to confirm CTL specificity, consisting of T2 cells without peptide or PMA/ionomycin-treated CTL for maximum spots, respectively. The ELISPOT assay was performed in 96-well plates (Nunc, Denmark). The capture and detection Abs and AEC substrate reagent were purchased from BD Biosciences (Human IFN-γ ELISPOT pair, AEC substrate reagent set). Spot numbers and sizes were determined with computer-assisted video image analysis (Cellular Technologies). Background, as determined by unstimulated samples, was subtracted in all cases.
APM component expression in DC
APM components TAP1, TAP2, LMP2 and tapasin were determined by flow cytometry (31, 32). Cells were fixed in 2% paraformaldehyde for 10min at RT and permeabilized in 100% methanol for > 24h at −20°C. Cells were then washed and incubated with primary APM component-specific mAb and FITC-conjugated secondary anti-mouse mAb for 30min at RT, respectively. A minimum of 100,000 events were acquired and the cut-off level was set at MFI (5) based on IgG2a isotype control.
Quantitation of tumor infiltrating lymphocytes (TIL)
H&E stained sections of 11 vaccinees’ blinded tumor sections were reviewed, and the intra-tumoral infiltrating lymphocytes (TIL) were enumerated in five high power fields (400×). Tumor cells comprised at least 60% of each field. The extent of TIL was determined by the average value of the TIL numbers in the five counted fields. As a relative measure of intratumoral inflammation, lymphocytic tumoral infiltration was considered mild, moderate, or prominent, if the TIL number was ≤ 10, 11–20, ≥20, respectively. Classification of patient clinical outcome and immune responses after vaccination were evaluated for each group to identify patterns associated with extent of TIL present.
Statistics
All averages were calculated as means. Paired samples were evaluated by Wilcoxon paired signed-rank test, and correlations were calculated by the Spearman test using SPSS software (IBM, version 19). The permutation test was used for significance of T cell reactivity. Comparison of three vaccination arms was performed by Kruskal-Wallis and consecutive Mann-Whitney-test. Maturation cocktails were compared for 8 different vaccine phenotypes, 4 APM components and 3 cytokines by Friedman’s test and with adjustment for false discovery for surviving signed rank tests of vDC vs DC1. Kaplan-Meier estimates and the log rank test were applied for evaluation of disease-free survival.
Results
Clinical information of vaccinated patients
Demographics of the wt p53 peptide-based DC vaccinated cohort are shown in Table 1. The vaccine was well tolerated by all patients, with skin rash at injection sites and circumscribed hematomas after leukapheresis in 2/16 patients, but no grade II-IV adverse events occurred. No differences were observed between vaccine arms. All patients were followed every 3 months using clinical examination and periodic PET/CT scans for 3 years.
Disease-free survival and tumor immunohistochemistry
Among the 16 vaccinated patients, 3 died of disease (DOD) at 16, 17 and 25 months; 13 patients remain alive with no evidence of disease (NED) the median follow-up of 32 months (range 6 – 42 months). Thus, two-year disease-free survival (DFS) was 88% and three-year DFS was 80% (Figure 1A). This DFS rate was favorable, as compared to the DFS of a similar, previously untreated stage III/IV clinical trial cohort treated with chemoradiation at our institution, as published previously (70%, 95% Confidence Interval 53% – 82% %, ref. (33)). Tumor samples obtained from the patients who underwent surgery (n=10) prior to vaccination were examined for expression of p53 in tumor cells. By IHC, 8 tumors were p53+ by IHC (Figure 1B) and 8 were p53neg. No significant difference in DFS between patients who had p53+ versus p53neg tumors was observed. HPV-16 status was available for 7 patients, of whom 4 tumors were HPV-16+ using p16 IHC and in situ hybridization (33). As expected HPV status strongly predicted clinical outcome even within this small patient group (Figure 1C).
Characteristics of vDC
Monocyte-derived matured DC generated for vaccine therapy (vDC) in the HNSCC patients showed significant up-regulation of surface markers HLA-DR, CD86, CD80 and CD83 relative to autologous mononuclear cells or immature (i)DC (Figure 2A). Phenotyping was performed after peptide loading, and there were no differences in expression of surface markers between DC pulsed with class I or class II peptides; therefore, their phenotypic results are grouped in Figure 2A. After DC-1 maturation, up-regulation of CD80, CD86, and HLA-A2 was stronger, while up-regulation of CD83 and CCR7 was weaker compared to vDC. Down-regulation of CD1a was greater in DC1 than in vDC (all adjusted signed rank p < .01).
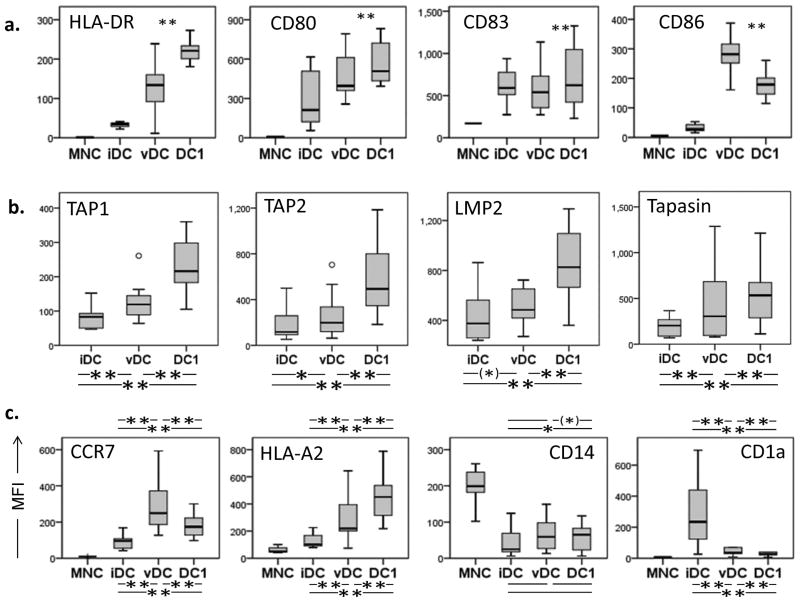
(a) Monocyte- derived immature DC (iDC) were loaded with wt p53 peptides and matured in a conventional cytokine cocktail prior to vaccination or in an alternative cytokine cocktail generating DC1. Mature DC for vaccination (vDC) and DC-1 were phenotyped for the maturation markers. Box plots for all patients’ vDC show quartiles for 25, 50, 75 as boxes, and values for 0% and 100% as whiskers. (b) Intracellular expression of antigen-presenting machinery (APM) components TAP1, TAP2, LMP2 and tapasin was determined in iDC and vDC of all study patients (n=16). Intracellular expression of antigen-presenting machinery (APM) components was determined in vDC and DC1 of all study patients (n=16). *p < 0.05, **p < 0.01. (c) Immature DC (iDC) were generated from monocytes (MNC) obtained from study patients with HNSCC. iDC were matured with two different maturation cocktails (vDC and DC1). MNC, iDC, vDC and DC1 were phenotyped for additional maturation markers. Wilcoxon paired signed-rank test **p < 0.005, *p < 0.05, (*) p < 0.1.
APM components were also measured, and TAP1/2, LMP2 and tapasin were modestly upregulated in vDC as compared to iDC, similar to our previous findings (31) (Figure 2B). Expression of antigen processing machinery (APM) components (TAP1, TAP2, LMP2 and tapasin) was measured in iDC, vDC and DC1. Although we observed an increased expression of APM components in vDC and DC1 relative to iDC (p < 0.05), upregulation of APM components was again significantly stronger in DC1 than in vDC (p < 0.05, Figure 2B). Significant differences were seen in the expression of additional surface maturation markers between DC matured in these two cocktails as shown in Figure 2C.
Peptide-specific CTL responses to the vaccine
Immune monitoring at baseline and at three post-vaccination time points included tetramer analysis for the frequency of wt p53-specific CTL (Figure 3A). Peptide-specific immune response was considered to be positive when the following criteria were met: (1) the frequency of p53-specific CTL was above LLD of 0.013%, as established previously (34); (2) the frequency of CTL for both peptide sequences (wt p53149-157 and wt p53262-274) at any two post-vaccination time points was increased as compared to baseline; and (3) the increase in tetramer-positive events was greater than 0.02%. Applying all three criteria, 69% (11/16) of patients showed a positive tetramer response. Specifically, 6/16 patients (38%) had a positive tetramer response for both peptide sequences (“full response”) and 5/16 patients (31%) had a response to one of the two peptides (“partial response”) as shown in Figure 3B. Five patients (31%) were nonresponsive, and although 3/5 of these patients were in Arm 3, no significant difference in tetramer responses could be attributed to the vaccination arms (p=0.46). Patients in Arm 1 had the most favorable CTL response but without reaching statistical significance. Patients who had tetramer responses were stratified against those with no evident or weak tetramer responses to determine whether wt p53 peptide-specific reactivity predicted DFS. As shown in Figure 3C, peptide-specific immune responses after vaccination did not differentiate patients based on DFS.
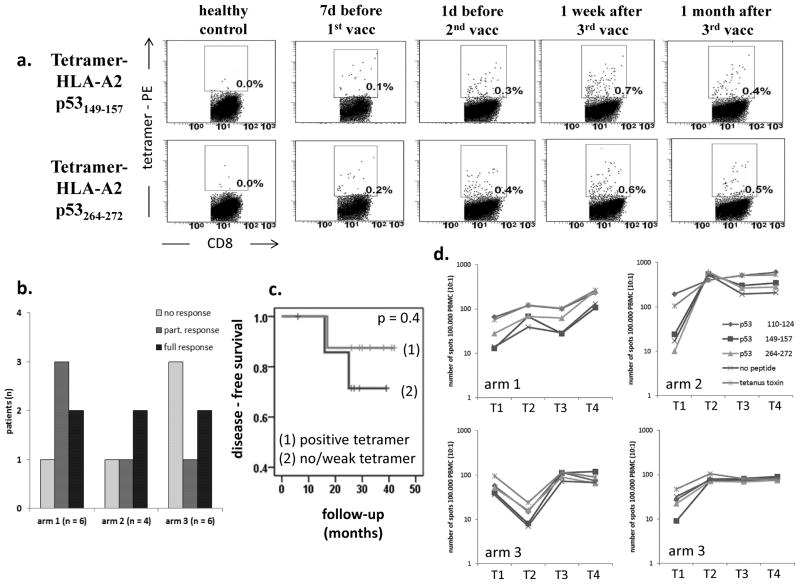
(a) The frequency of CTL specific for wt p53149-157 or wt p53262-274 sequences in patients’ peripheral blood was determined by tetramer-flow cytometry at baseline and at three follow-up time points, as indicated. Dot plots are from one representative HNSCC patient and one normal control (NC). Boxes indicate tetramer-positive CD8+ T cells. (b) Numbers of patients in each treatment arm with positive, partial or no tetramer response. 6/16 patients (38%) showed a positive immune response; 5/16 patients (31%) showed a partial response with an elevated CTL frequency for only one peptide; and 5/16 patients (31%) showed no peptide-specific immune response to both wt p53 peptides. Patients in treatment arm 1 had the most favorable immune response, without reaching significance (p > 0.2). (c) DFS by the positivity of tetramer responses; patients are classified as ‘positive tetramer response’ or ‘no/weak tetramer response’. Overall DFS was similar for each tetramer response group (log rank p = .754). (d) Representative IFN-γ ELISPOT data at four different time points: T1: baseline, T2: one day before 2nd vaccine, T3: one week after 3rd vaccine, and T4: one month after 3rd vaccine. CTL reactive against wt p53 peptides, tetanus toxoid or no peptide were measured in PBMC stimulated with peptide-loaded T2 cells. Longer term follow up data are shown for four patients with a positive immune response.
ELISPOT assays
The presence of IFN-γ producing CTL in patients’ PBMC upon stimulation with T2 cell pulsed with the wt p53 peptides was measured in ELISPOT assays. Serial assays were performed at all 4 time points. Representative data for single tetramer-positive patients (one in each Arm) are shown in Figure 3D. The data show variably increased numbers of spots at the post-vaccination time point vs. background values for four selected patients who were tetramer responders. These were the only patients among 16 tested with positive ELISPOT results. Interestingly, positive IFN-γ responses to the wt p53 peptides as well as to the tetanus peptide were seen, suggesting epitope spreading and/or non-peptide specific activation of immune cells in these 4 patients.
Regulatory T cells (Treg) levels after vaccination
In this study, Treg were evaluated by flow cytometry and defined as CD4+CD25+CD39+ T cells, as shown in Figure 4A. We have previously reported that these Treg cells were highly FoxP3+ and mediated suppression of autologous CD4+ T cell proliferation after in vitro SEB stimulation (35). The frequency of CD4+CD25+CD39+ Treg was determined at baseline and after administration of the third vaccine (days 35 – 56). As expected, the frequency of Treg was increased at baseline in HNSCC patients (8.1 ± 3.5%) in comparison to normal controls (NC, 3.4 ± 2.1%, n=40, p< 0.001). Notably, after vaccination, the frequency of Treg (Figure 4B) as well as the absolute number of Treg (Figure 4C) were significantly decreased (p<0.006 and p<0.005, respectively). The Treg frequency was decreased in 12/15 patients evaluated and the absolute number was decreased in 15/16 patients. After vaccination, the mean absolute number of Treg was lower than that present in the circulation of NC (28 ± 6 per μL; n=20; p<0.01). In some patients, a decrease in the Treg frequency after vaccination was accentuated by an increase in total CD4+ T cell frequencies. In the patient cohort as a whole, the frequency of Treg in the peripheral blood had a strong inverse correlation with the absolute number of CD4+ T cells (p<0.01, Figure 4D).
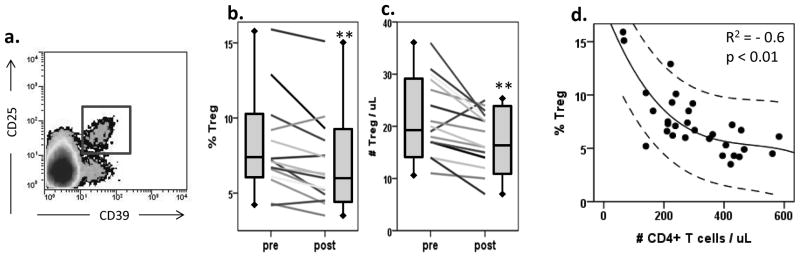
(a) Treg were defined as CD4+CD39+CD25+ cells by flow cytometry, which are regularly identified as a defined population. (b) Percentages of CD4+CD39+CD25+ Treg in the peripheral blood of patients (n=16) before and after vaccination. Wilcoxon paired signed-rank test **p = 0.006. (c) Absolute numbers of CD4+CD39+CD25+ Treg in the peripheral blood of patients (n=16) before and after vaccination. Wilcoxon paired signed-rank test **p = 0.005. (d) The frequency of Treg in the peripheral circulation inversely correlates to the absolute number of CD4+ T cells (R2 = −0.6, p < 0.01). Upper and lower lines denote 95% confidence interval.
In order to determine whether the overall decrease in Treg number is due to vaccination-independent non-specific contribution of tumor-infiltrating lymphocytes (TIL), we analyzed 11available pre-vaccine tumor specimens histologically. The surgical resection preceded vaccination by 2 mo – 23 mo, and these tumor specimens showed varied extent of TIL levels. Four tumors showed prominent (>20TIL/HPF), two tumors showed moderate (10–20 TIL/HPF), and four tumors showed mild TIL content (<10 TIL/HPF) within the cancer cell islands. Of the latter four patients with minimal TIL content, two had died (of three total deaths in the trial cohort). No correlation was found between time of resection and clinical or immunologic parameters.
Functional studies with HNSCC patients’ DC generated by an alternative protocol
The functional characteristics of DC matured by different maturation regimens were also determined. To test this, immature DC (iDC) generated from cryopreserved CD14+ monocytes of 13/16 patients were matured by an alternative cytokine protocol. Thus, iDC were matured for 48h in the presence of the DC1 cocktail of cytokines (21), containing IFNs and polyI:C, as described in Materials and Methods. In parallel, using the same iDC, maturation was performed with the cytokine cocktail utilized for maturation of the vDC administered to patients. The peptide-pulsed vDC and DC1 were also tested for cytokine secretion (IL-10, IL-12, VEGF) by Luminex™. As shown in Figure 5A, vDC produced more IL-10, IL-12 and VEGF than iDC; however, the ratios of IL-10/IL-12 were not different in vDC loaded with combinations of peptides and used in Arms 1 to 3 (Figure 5B). As shown in Figure 5A, DC1 produced more IL-12 and less IL-10 than did vDC, and only vDC produced VEGF. The data suggest that vDC have an immunosuppressive functional profile relative to DC1 which mediate stronger adjuvant effects. In Figure 5C, a significant negative correlation between IL-10 and IL-12 levels in DC1 is shown as well as a positive correlation between VEGF and IL-10 in vDC, further illustrating the cytokine profile associated with vDC.
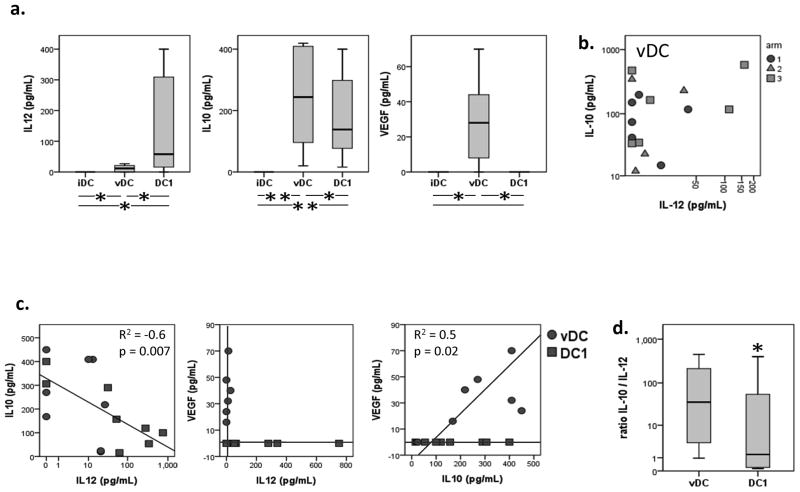
(a) Production of cytokines IL-10 and IL-12 was measured by LuminexTM in CD40L-stimulated vDC (b) and DC1. (c) Production of IL-10 and IL-12 was inversely correlated in all DC (R2 = −0.6, p = 0.007) and production of IL-10 and VEGF was positively correlated in vDC (panel (d), R2 = 0.5, p = 0.02). Wilcoxon paired signed-rank test *p < 0.05, **p < 0.01.
Discussion
Therapeutic vaccination strategies in HNSCC have been limited to the use of virus-modified tumor cells (1) or heat shock protein HSP65 (2), and both these approaches have reported considerable toxicity and low vaccine efficacy. The present clinical vaccination trial is, to the best of our knowledge, the largest tumor-peptide specific DC-based vaccination trial in HNSCC reported to date, and it is the first to incorporate a T helper (Th) peptide derived from the same TA, p53, while using modified/optimized HLA class I binding peptides to generate CTL. It has been previously shown that the induction of tumor-specific CTL required cross-presentation of the relevant helper peptides via class II molecules on mDC (17, 22). Activated CD4+ T helper cells up-regulate expression of surface CD40 and produce Th1 cytokines (IFN-γ, IL-2), which have positive effects on DC maturation as well as CTL function (36). The class II wt p53 epitope (p53110-124) we incorporated in the vaccine was previously identified as a potent stimulator of p53 class I presentation on DC.(17) This phase I DC-based vaccination trial targeting p53 tested the hypothesis that transfer of wt p53 peptide-loaded DC to HNSCC patients could be therapeutically beneficial and that the vaccine-induced benefits could be related to its ability to induce anti-tumor immune responses.
Clinical outcome of this vaccination therapy following previous surgery or chemoradiation suggested that 2-year DFS of the patients may have been increased, as compared to historical controls comprising a cohort of similarly staged, unvaccinated patients. DFS is approximately 50–70% for advanced HNSCC (stage III and IV) patients after conventional treatment.(37–39) In single-arm phase II adjuvant trials, experimental therapy is generally considered worthy of future study, if 2-year DFS is increased from 50% to 70%.(40) In the present phase I trial, 2-year PFS was 88%, providing a rationale for a future confirmatory phase II vaccine trial in HNSCC. Interestingly, DFS did not correlate with the presence or expression levels of p53 in the tumor cells or with positive tetramer staining of wt p53 peptide-specific CD8+ T cells in the patients’ peripheral blood after vaccination, suggesting that reduction of the poor prognostic influence of Treg may provide a global beneficial impact and serve as a biomarker for correlation with clinical outcome in a prospective phase II trial. Indeed, a strong and functional lymphocytic response is beneficial for patients’ clinical response. In this phase I trial, two of the three patients who died of disease had only mild TIL within the specimen, suggesting the significance of TIL in anti-tumor immunity. However, the time lapse between surgery and vaccination in this trial expanded from a few months to a couple of years, making the interpretation of the impact of the baseline TIL on patients’ survival of interest for further trials involving larger patient numbers, thus permitting statistical analyses..
The observed limited and weak post-vaccination anti-wt p53 peptide-specific immunologic responses in patients with advanced HNSCC could be explained in several different ways. Foremost among them is the well-known immunosuppressive influence of the tumor microenvironment in HNSCC patients (41). HNSCC is characterized by a potent tumor-induced immune suppression mediated by Treg, MDSC, tumor-derived factors and exosomes. Tumor-induced suppression involves all components of the host immune system, including DC, T helper cells, CTL, NK and B cells, and is especially evident in advanced disease. It is, therefore, likely that the vaccine consisting of matured DC loaded with immunogenic cytotoxic and helper wt p53 peptides was unable to overcome extensive immune suppression present in HNSCC patients and induce strong anti-tumor immune responses.
Nevertheless, the vaccine was apparently able to induce changes in immunoregulatory mechanisms. One consistent finding in nearly all vaccinated patients was a significant reduction in the frequency and absolute number of circulating Treg after vaccination. This decline in Treg was not restricted to the protocol arms using a class II-restricted peptide in the vaccine but occurred in nearly all vaccinated patients. In addition, the overall decrease in Treg frequency and absolute number in almost every patient in this cohort appeared to be a specific result from vaccination and unrelated to the intra-tumoral inflammation status, given the fact that the examined tumor specimens displayed a wide range of TIL levels, from as few as 6 TIL per high power field to more than 50 TIL per high power field.
As Treg are known to suppress effective anti-tumor immune responses and impair patient prognosis (42, 43), their normalization post-vaccine could be expected to restore or upregulate anti-tumor immunity. However, despite consistently seen decline in the Treg frequency and absolute numbers after vaccination, anti-wt p53-specific responses measured by tetramer analysis or ELISPOT assays were few and weak. The role of Treg in human cancer is still controversial, and our finding implying that Treg normalization by the vaccine is not sufficient for restoration of anti-tumor immunity further highlights the uncertain role these cells seem to play in cancer progression. In contrast to our study, many DC-based vaccines have been reported to increase the frequency of circulating Treg relative to that before vaccination. This discrepancy could be in part explained by the fact that reliable surface markers controversial for human Treg. By using CD39, an ectonucleotidase, in addition to CD25 as surface markers for Treg (29), we can reliably distinguish activated CD4+CD39neg conventional T cells from CD4+CD39+ CD25+ Treg and thus increase the specificity of Treg phenotyping. It is perhaps important to note that a decrease in CD39+ Treg implies that the vaccine also had an impact on the adenosine pathway, which is known to be involved in down-regulation of immune cell functions. We acknowledge that additional immunosuppressive subsets exist in HNSCC patients, including MDSC, and with a small number of available residual specimens we were unable to detect a consistent vaccine effect on MDSC frequency in PBMC (data not shown). Further vaccine trials will clearly need to incorporate studies and disruption of these cells to permit full efficacy of vaccination, in addition to Treg reduction.
One of the key objectives of DC-based vaccination is to restore suppressed anti-tumor immunity by providing effective antigen presentation and strong adjuvant activity (31, 32). In HNSCC patients, DC are not fully competent for T cell priming, likely due to their incomplete maturation (19, 21). Thus, DC generation and maturation for vaccine production is a critical step. It is clear that vDC used for therapy in this trial were not functionally optimal despite a normal surface expression of co-stimulatory and HLA molecules as evaluated by flow cytometry. Importantly, vDC produced little IL-12 and an excess of immunoinhibitory factors, IL-10 and VEGF; they did not up-regulate expression of crucial APM components upon maturation in the presence of the conventional cytokine cocktail. The ex vivo comparison of vDC with DC1 matured in parallel from iDC of the same HNSCC patients by an alternative cytokine cocktail convincingly demonstrates that superior DC can be generated from monocytes derived from the blood of HNSCC patients using alternative maturation cocktails. Further, it has been determined that phenotypic and functional characteristics of vDC generated from patients with cancer differ from those of DC generated from normal donors (16). Since the vaccination outcome might depend on the quality of injected vDC, it is necessary to ensure that vDC are mature and functionally effective.
Given the fact that DC generated from HNSCC patients preferentially produced IL-10 and had only mild adjuvant effects in vivo, we considered a possibility that vDC were not optimally matured. To test this hypothesis, immature DC (iDC) generated from cryopreserved CD14+ monocytes of 13/16 patients were matured by an alternative cytokine protocol. Thus, iDC were matured for 48h in the presence of the DC1 cocktail of cytokines (21), containing IFNs and polyI:C, as described in Materials and Methods. In parallel, using the same iDC, maturation was performed with the cytokine cocktail utilized for maturation of the vDC administered to patients (Figures 2 and and5).5). Our studies suggest that DC generated for clinical applications should be critically monitored not only for surface maturation markers, but especially for intracellular APM component expression and for their cytokine production profile. For example, APM component TAP1 might serve as a good measure of DC maturation as shown by our recently published data (31).
In addition to vDC quality, the potential of any vaccine to induce anti-tumor immune responses depends on the selected target TAA. The selection of wt p53 as a vaccination target is based on recent data indicating that p53 mutation is the dominant genetic event in HNSCC (>60% mutated) and that p53 frequently accumulates in tumor cells, making the wt p53 peptides the most ubiquitous TAAs (44). The self-antigen, p53, consists of many low- to moderate-affinity wt peptide sequences, some of which have been shown to be immunogenic (14, 15, 17, 32). Vaccination with the modified (HLA-A2 binding) wt p53 peptides plus T helper peptides is designed to stimulate both CD8+ and CD4+ T cells and thus increase the immunogenic effects of the vaccine. Also, the delivery of the combination of wt p53 cytotoxic and helper peptides on matured autologous DC is expected to improve antigen presentation to T cells in the context of strong adjuvant effects needed for improving the vaccine efficacy. However, since the wt p53 peptides are expressed at low levels in normal tissues, vaccines targeting p53 peptides may have to overcome a high level of immunologic tolerance to self (45). Therefore, the three vaccine administrations used in the present study might be insufficient for induction of a detectable and persistent response. Other p53-peptide based vaccination studies have applied up to ten courses of DC-vaccination in order to induce peptide specific immune responses (46). In light of the absence of serious side effects in this study, the number of vaccination courses could be increased, enhancing the likelihood of a positive immune response against wt p53-peptides.
It should be emphasized that the HNSCC patients enrolled in this trial were treated with either surgery or chemoradiation prior to receiving the vaccine. To date, the optimal sequence, timing or combination of various therapeutic cancer regimens is still unclear. Chemotherapeutic drugs, e.g. cisplatin, can increase susceptibility of tumor cells to CTL mediated killing. On the other hand, chemotherapy reduces the T cell pool available for DC-based vaccination and expands the frequency of chemoresistant Treg. Therefore, it may be an advantage to begin tumor-specific vaccination before chemoradiation therapies. Alternatively, chemotherapeutic regimens or targeted therapies which expand immune responses, such as taxanes or cetuximab (Erbitux™) (33), might be preferentially selected for combinatorial immunotherapy.
Supplementary Material
1
2
Figure S1:Study patients with advances HNSCC were assigned to three different treatment arms. ‘Arm 1’ was vaccinated with dendritic cells (DC) loaded with p53-specific peptides (class I) for sequences 149-157 (modified T150L) and 264-272 (modified F270W). In treatment ‘arm 2’ DC were additionally loaded with tetanus peptide (class II). In treatment ‘arm 3’ DC were additionally loaded with p53-specific peptides (class II) for sequence 110-124.
3
Figure S2:Representative examples of flow cytometric gating and quantification of CD4, CD25, CD39, and FOXP3 on Treg subsets.
References
Full text links
Read article at publisher's site: https://doi.org/10.1158/1078-0432.ccr-13-2617
Read article for free, from open access legal sources, via Unpaywall:
https://clincancerres.aacrjournals.org/content/clincanres/20/9/2433.full.pdf
Citations & impact
Impact metrics
Citations of article over time
Alternative metrics
Smart citations by scite.ai
Explore citation contexts and check if this article has been
supported or disputed.
https://scite.ai/reports/10.1158/1078-0432.ccr-13-2617
Article citations
Primary cilia-associated signalling in squamous cell carcinoma of head and neck region.
Front Oncol, 14:1413255, 21 Aug 2024
Cited by: 0 articles | PMID: 39234399 | PMCID: PMC11372790
Review Free full text in Europe PMC
A pseudotyped adenovirus serotype 5 vector with serotype 49 fiber knob is an effective vector for vaccine and gene therapy applications.
Mol Ther Methods Clin Dev, 32(3):101308, 30 Jul 2024
Cited by: 0 articles | PMID: 39206304 | PMCID: PMC11357811
Autoantibodies in cancer: a systematic review of their clinical role in the most prevalent cancers.
Front Immunol, 15:1455602, 21 Aug 2024
Cited by: 0 articles | PMID: 39234247 | PMCID: PMC11371560
Review Free full text in Europe PMC
Head and neck cancer: pathogenesis and targeted therapy.
MedComm (2020), 5(9):e702, 21 Aug 2024
Cited by: 1 article | PMID: 39170944 | PMCID: PMC11338281
Review Free full text in Europe PMC
Dysfunction of dendritic cells in tumor microenvironment and immunotherapy.
Cancer Commun (Lond), 44(9):1047-1070, 25 Jul 2024
Cited by: 1 article | PMID: 39051512 | PMCID: PMC11492303
Review Free full text in Europe PMC
Go to all (84) article citations
Data
Data behind the article
This data has been text mined from the article, or deposited into data resources.
BioStudies: supplemental material and supporting data
Clinical Trials
- (1 citation) ClinicalTrials.gov - NCT00798655
Similar Articles
To arrive at the top five similar articles we use a word-weighted algorithm to compare words from the Title and Abstract of each citation.
p53 as an immunotherapeutic target in head and neck cancer.
Adv Otorhinolaryngol, 62:151-160, 01 Jan 2005
Cited by: 8 articles | PMID: 15608425
Phenotype of p53 wild-type epitope-specific T cells in the circulation of patients with head and neck cancer.
Sci Rep, 8(1):10716, 16 Jul 2018
Cited by: 7 articles | PMID: 30013227 | PMCID: PMC6048165
Generation of T cells specific for the wild-type sequence p53(264-272) peptide in cancer patients: implications for immunoselection of epitope loss variants.
J Immunol, 165(10):5938-5944, 01 Nov 2000
Cited by: 45 articles | PMID: 11067956
Dendritic cell gene therapy.
Surg Oncol Clin N Am, 11(3):645-660, 01 Jul 2002
Cited by: 19 articles | PMID: 12487060
Review
Funding
Funders who supported this work.
NCATS NIH HHS (1)
Grant ID: UL1 TR000005
NCI NIH HHS (7)
Grant ID: 1R01 CA110249
Grant ID: R01 CA110249
Grant ID: P30CA047904
Grant ID: P50 CA097190
Grant ID: R01 CA168628
Grant ID: P01 CA109688
Grant ID: 1P50 CA097190
NIDCR NIH HHS (2)
Grant ID: R00 DE024173
Grant ID: K99 DE024173