Abstract
Free full text

At the Bench: Helicobacter pylori, dysregulated host responses, DNA damage, and gastric cancer
Abstract
Helicobacter pylori infection is the strongest known risk factor for the development of gastric cancer. Given that ~50% of the global population is infected with this pathogen, there is great impetus to elucidate underlying causes that mediate progression from infection to cancer. Recent evidence suggests that H. pylori-induced chronic inflammation and oxidative stress create an environment conducive to DNA damage and tissue injury. DNA damage leads to genetic instability and eventually, neoplastic transformation. Pathogen-encoded virulence factors induce a robust but futile immune response and alter host pathways that lower the threshold for carcinogenesis, including DNA damage repair, polyamine synthesis and catabolism, antioxidant responses, and cytokine production. Collectively, such dysregulation creates a protumorigenic microenvironment within the stomach. This review seeks to address each of these aspects of H. pylori infection and to call attention to areas of particular interest within this field of research. This review also seeks to prioritize areas of translational research related to H. pylori-induced gastric cancer based on insights garnered from basic research in this field. See related review by Dalal and Moss, At the Bedside: H. pylori, dysregulated host responses, DNA damage, and gastric cancer.
Introduction
Gastric cancer is the second leading cause of cancer-related deaths worldwide [1]. The single greatest risk factor for the development of this malignancy is infection with H. pylori, which is a Gram-negative, microaerophilic bacterium that selectively colonizes the human stomach [2]. H. pylori infects ~50% of the global population and leads to gastritis, peptic ulceration, gastric adenocarcinoma, and mucosa-associated lymphoid tissue lymphoma [1, 3]. As H. pylori confers an attributable risk of 75% for gastric carcinogenesis, the World Health Organization has classified this organism as a type I carcinogen [4]. Risk factors for the development of gastric cancer include infection with cagA-positive strains of H. pylori, the phylogeographic origin of the infecting strain, and several host genetic factors, including polymorphisms in genes encoding IL-1β, IL-8, IL-10, TNF-α, and IFN-γ [5,–7]. Many of these polymorphisms are discussed in detail in the accompanying review by Dalal and Moss. Thus, bacterial and host risk factors are important, but the ability to predict gastric cancer risk remains elusive. It is still unclear why only 1–3% of the infected population will progress to cancer. Thus, more research is necessary to better understand which factors are most critical for progression from H. pylori infection to gastric cancer.
During infection, the host immune system mounts a robust response that integrates innate and adaptive constituents, as well as the generation of a humoral response [8, 9]. Despite such a vigorous response, eradication of this pathogen is rarely achieved in the absence of antibiotics, leading to sustained inflammation over the lifetime of the infected host. Chronic inflammation leads to tissue injury, oxidative stress, and DNA damage [8, 10,–14]. We hypothesize that these highly deleterious outcomes of infection create a procarcinogenic environment in the stomach and contribute to gastric carcinogenesis. Thus, there is a need for fresh insights into the nature of chronic inflammation caused by H. pylori infection and its effect on gastric carcinogenesis. This review, in concert with the accompanying clinical review by Dalal and Moss, addresses the roles of H. pylori-synthesized factors, innate and adaptive responses, epithelial signaling, and metabolic events that lead to chronic inflammation, oxidative stress, and DNA damage within the context of gastric carcinogenesis.
H. pylori VIRULENCE CONSTITUENTS
cagA
cagA is one of the most potent virulence factors expressed by H. pylori, with pleiotropic effects on epithelial and immune cells within the stomach. Individuals infected with strains of H. pylori that possess the cagA gene harbor a markedly increased risk of developing gastric cancer (odds ratio=1.9) over persons infected with a cagA− strain [6]. CagA is translocated into host cells via a T4SS that is encoded on the same pathogenicity island as the cagA gene. The T4SS is composed of several proteins, and studies are ongoing to identify the functions of all of the T4SS constituents [15]. Once in the cell, host kinases phosphorylate CagA, which initiates a variety of CagA functions (Fig. 1), including activation of MAPKs, inhibition of apoptosis, disruption of cell growth, and dysregulation of cell motility [16, 17]. CagA phosphorylation occurs at the EPIYA motifs. The particular EPIYA motif that a strain harbors has been associated with varying degrees of clinical sequelae [18]. Intriguingly, the mechanism by which CagA increases an individual's risk for gastric cancer has not been fully elucidated.
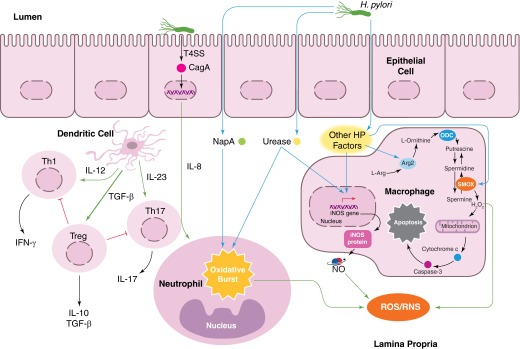
H. pylori infection leads to IL-8 production, which may be dependent on CagA translocation. IL-8 is a chemoattractant that recruits neutrophils. H. pylori NapA and urease cause neutrophils to undergo an oxidative burst, exuding substantial amounts of ROS into the infected microenvironment. Moreover, NapA leads to increased survival in neutrophils, compounding their effects on oxidative stress. Macrophage iNOS is induced by urease during infection, leading to the production of NO, a RNS. As yet, undetermined H. pylori (HP) factors induce Arg2 activation in macrophages, resulting in apoptosis. ODC is also induced in macrophages during infection. This leads to downstream H2O2 production by SMOX and apoptosis. Moreover, H. pylori infection alters the cytokine milieu produced by DCs in favor of an increased Treg response and a decreased Th1/Th17 response. Such alterations promote immune tolerance and persistence of the pathogen.
H. pylori strains that are cagA+ have been shown to induce higher levels of expression of TNF-α, IL-1β, and IL-8 in gastric epithelial cells (Fig. 1) [6, 19, 20]. Each of these cytokines is proinflammatory and represents markers of increased oxidative stress [10]. Moreover, a recent report from our group indicates that infection with cagA+ strains is associated with significantly increased levels of H2O2 production [21]. Consequently, infected cells and gastric tissues exhibit increased oxidative DNA damage, as measured by 8-oxoG and 8-OHdG levels [21]. In the well-established paradigm of carcinogenesis, DNA damage and mutations—especially within an oncogene or tumor-suppressor gene—are fundamental causes of cancer [22]. Thus, these data suggest that CagA-induced DNA damage is a plausible link between infection and the risk for gastric cancer [21].
However, cagA is not the only microbial factor that increases cancer risk. A study of a cohort of patients from Colombia, a region with high infection rates but varying gastric cancer rates, revealed that the phylogeographic origin of an infected individual's strain also incurs an increased risk for cancer [5]. Even among cagA+-infected individuals, those colonized with H. pylori strains with European ancestry demonstrated increased oxidative stress and increased severity of preneoplastic lesions compared with cagA+ strains of African origin [5]. These data indicate that there may, in fact, be multiple H. pylori-derived factors that contribute to gastric carcinogenesis. Additionally, the coevolution of H. pylori with its human host shapes the risk for gastric carcinogenesis, as the effect of the phylogeographic origin of infecting strains is dependent on the genetic origins of an infected individual [23].
vacA
Another H. pylori virulence factor linked to gastric cancer is vacA, which enhances the ability of H. pylori to colonize successfully and persistently human gastric mucosa [24]. Moreover, strains harboring the vacA s1m1 allele are associated with increased severity of gastric inflammation and cancer risk [24]. VacA increases intracellular concentrations of ROS and augments oxidative stress in epithelial and immune cells [24,–26]. However, the precise mechanism by which VacA induces these responses remains incompletely defined.
The mitochondrial membrane is one of several VacA targets [27]. One study indicated that VacA leads to the generation of ROS within the cytoplasm, as measured by DCFDA staining [25, 28]. This response was also observed in eosinophils and HeLa cells, indicating a more generalized effect of VacA intoxication during H. pylori infection. Concurrently, VacA leads to release of Ca2+ that causes activation of NF-κB [25]. Increased NF-κB activity results in expression of proinflammatory cytokines, which function to recruit other immune cells to the site of infection [25].
VacA has also been shown to play an enhancing and inhibitory role in autophagy [24, 26]. One recent study found that VacA is responsible for disrupting autophagy in human gastric epithelial cells [24]. Autophagy is critical for the clearance of intracellular debris, including ROS. As such, VacA-mediated disruption of autophagy results in an accumulation of intracellular ROS [24]. In contrast, a second study found that VacA has the capacity to induce autophagy within epithelial cells [26]. VacA can bind to LRP1 [26], and LRP1 activation results in the accumulation of intracellular ROS, which subsequently leads to the activation of protein kinase B and mouse double-minute 2 phosphorylation [26]. Consequently, p53 is degraded, and autophagy is induced [26]. In either role, VacA leads to an accumulation of ROS and contributes to oxidative stress during H. pylori infection.
Other factors
Several other microbial factors produced by H. pylori have been implicated in the initiation of chronic inflammation and oxidative stress during infection. NapA is primarily responsible for the recruitment of neutrophils to the site of infection (Fig. 1) [29]. NapA is sufficient to induce the neutrophil oxidative burst, resulting in an influx of oxyradicals into the site of infection [29]. Oxyradicals create foci of high oxidative stress and cause collateral tissue damage. Interestingly, H. pylori is protected from oxyradical-mediated damage by a NapA-dependent mechanism [29]. The inability of the phagocytic burst to kill the pathogen, therefore, fails to halt the production of NapA and thus, perpetuates the infiltration of neutrophils poised to release highly toxic ROS.
Urease (Fig. 1) is another H. pylori virulence factor that is capable of recruiting neutrophils to the site of infection [30]. Recombinant urease is sufficient to induce neutrophil infiltration in a paw edema model in WT Swiss mice, leading to high levels of ROS and increased inflammation [30]. To the detriment of the host, infiltrating neutrophils, which are typically thought to be short-lived, demonstrate increased resistance to apoptosis and an increased lifespan [30]. Increased neutrophil longevity may be a contributing factor in establishing chronic gastric inflammation, a hallmark of H. pylori infection [8, 9]. The immunologic activity of urease is also evidenced by findings that urease is a potent inducer of iNOS in macrophages, thus contributing to innate immune activation, and nitrosative stress specifically, as discussed below [31].
H. pylori GGT also contributes to disease severity during infection by stimulating H2O2 production in gastric epithelial cells [32]. This induces a corresponding increase in NF-κB activation and IL-8 production [32]. IL-8 is a proinflammatory cytokine that is highly abundant within gastric tissue during H. pylori infection and is a hallmark for initiation of inflammation, as it is a C-X-C chemokine that serves as a chemoattractant for neutrophils [19, 33, 34]. Additionally, IL-8 has protumorigenic properties [35, 36]. When added to gastric epithelial cells, rGGT resulted in increased levels of 8-OHdG, an indicator of oxidative DNA damage, as measured by flow cytometry [32]. Increased ROS, proinflammatory cytokine production, and oxidative DNA damage create ideal conditions for the progression from infection to cancer.
INNATE AND ADAPTIVE IMMUNITY
The aforementioned H. pylori-derived factors are necessary for the induction of chronic inflammation and oxidative stress. However, these bacterial factors are not sufficient for the progression from infection to gastric cancer. The failure of the human immune system to eradicate the pathogen leads to a futile inflammatory response, which has been implicated directly in carcinogenesis [9].
Innate immunity
The oxidative burst from phagocytes—primarily neutrophils and macrophages—comprises a major component of the initial immune response to H. pylori (Fig. 1) [10]. As discussed previously, H. pylori is capable of inducing oxidative bursts, which release significant amounts of oxyradicals into the infected microenvironment [12, 29]. It is notable that several models of colon cancer have demonstrated that chronic inflammation leads to enhanced ROS production, increased DNA damage, and more severe disease pathology [37, 38]. Thus, the colon cancer model serves as a point of reference from which investigators can address similar questions in gastric cancer.
Unlike many other human pathogens, H. pylori has the ability to survive the oxidative burst. NapA, catalase, and superoxide dismutase work in concert to alleviate the stress of this response [12]. Furthermore, H. pylori can survive oxidative damage by subverting phagolysome-mediated killing in a VacA-dependent manner, the details of which remain to be determined [39]. The ability of H. pylori to evade the innate immune response creates a cycle of consistent immunocyte infiltration, oxidative burst, and DNA damage that contributes to gastric carcinogenesis.
In addition to generating an oxidative burst, macrophages express iNOS and produce NO in response to H. pylori infection (Fig. 1) [31, 40]. NO is a powerful signaling molecule that regulates immune function and exerts antimicrobial properties [41]. NO can eradicate H. pylori but only when released in very high concentrations [11, 42]. Marked increases in NO contribute to increased nitrosative stress. Although a direct causal link has not been established between nitrosative stress and H. pylori-induced gastric cancer, RNS likely cooperate with ROS to create an environment conducive to DNA damage and neoplastic transformation. In fact, RNS have been implicated as mediators of DNA damage in H. pylori-infected mice [43].
In humans, increased expression of iNOS occurs in immune cells and gastric epithelial cells, suggesting that NO may also play a role in H. pylori-induced injury [34]. However, the interactions among NO signaling, RNS, and nitrosative stress remain unclear, and more studies are needed to address their potential link to gastric carcinogenesis.
Additionally, H. pylori induces the expression of Arg2 and ODC, leading to increased macrophage apoptosis [44]. Indeed, we have demonstrated that the rapid infiltration of macrophages into the stomach in a murine model of infection is matched by a corresponding increase in the level of apoptosis of these cells [45]. This is mediated by induction of c-Myc that enhances expression of ODC [45, 46] and the intrinsic pathway of apoptosis [47]. The loss of the macrophage component at the site of infection is another hallmark of the failed immune response to H. pylori.
Another key aspect of the insufficient immune response is incomplete polarization of macrophages to the antibacterial M1 phenotype. We have reported that oral administration of DFMO, an irreversible inhibitor of ODC, reduces H. pylori colonization in mice, and this was associated with increased iNOS protein expression and NO production by gastric macrophages [42]. Additionally, mice with deletion of Arg2 exhibit an increased inflammatory response to H. pylori and reduced colonization levels [48]. Whereas ODC and Arg2 are not the prototype markers of M2, they function in much the same way by reducing antimicrobial NO production and thus, an M1 response. Recently, Mregs have been described, representing a third class of macrophage subtypes [49]. We have found that gastric macrophages from H. pylori-infected mice can express IL-10 and TGF-β, which also can act to impair the M1 response. By failing to eradicate the bacteria, the innate immune system continually mounts a response to H. pylori, further contributing to the malicious cycle of immune cell ROS production, oxidative stress, and DNA damage.
DC activation by H. pylori has also been demonstrated in a number of studies [8, 50]. Intriguingly, it appears that as in the intestine, DCs send dendrites into the gastric lumen to sample lumen antigens [51]. It is established that signaling through DCs can have potent effects on activating Th1 and Th17 responses [51]. However, in the case of H. pylori infection, DCs can also contribute to the bacterial persistence by inducing immune tolerance, discussed below.
Adaptive immunity
The adaptive branch of the immune system, specifically Th cells, has also been implicated as contributory to H. pylori-induced chronic inflammation [52]. Th1 and Th17 T cell subsets primarily mediate chronic gastritis (Fig. 1) [53, 54]. Th1 cells secrete high levels of proinflammatory IFN-γ, and IL-17 produced by Th17 cells has been shown to enhance IL-8 production [52, 53, 55, 56]. These proinflammatory cytokines serve to recruit immunocytes to the site of infection and perpetuate the chronic inflammatory state of an infected host. Moreover, the influx of Th1 and Th17 cells into the gastric mucosa has been associated with increased epithelial damage, increased epithelial proliferation, and metaplastic responses [57].
Numerous studies have also found that Tregs are present within the gastric mucosa during infection [51, 58,–60]. H. pylori, acting through DCs, can preferentially induce a Treg response versus Th1/Th17 responses (Fig. 1) [51, 61]. This is in marked contrast to findings in models of colitis, where DCs and macrophages are linked to activation of Th1/Th17 responses. Moreover, IL-10 produced by Tregs results in decreased IL-8 production [60]. This facilitates bacterial persistence within the host and promotes immune tolerance. The role of innate lymphoid cells as major producers of IL-17 remains an area to be investigated in H. pylori infection.
It has been noted that in areas of particularly high gastric cancer risk, children that are infected with H. pylori demonstrate significantly higher levels of gastritis than children in low-risk regions [62]. However, when compared with adults, a cohort of H. pylori-infected children in Santiago, Chile, demonstrated increased levels of Tregs and the regulatory cytokine TGF-β [63]. Additionally, these Chilean children have a down-regulated IL-17 response and thus, decreased gastritis scores [64]. These data provide a conceptual framework, suggesting that the fact that gastric cancer is a disease of middle to old age may relate to loss of immune tolerance in the form of Tregs as individuals age, leading to more inflammation and ultimately, more oxidative DNA damage.
Taken together, these data clearly implicate innate and adaptive immune cells in the induction of oxidative stress, inflammation-induced tissue damage, and the progression to cancer.
ALTERED CELLULAR PROCESSES
DNA damage and repair
H. pylori infection is strongly associated with DNA damage, one of the most deleterious outcomes of chronic oxidative stress and high ROS concentrations [65]. The inability to contain oxidative stress effectively and repair damaged DNA leads to neoplastic transformation and carcinogenesis. As has been discussed, H. pylori is capable of inducing high levels of ROS production from the neutrophil oxidative burst [29]. Moreover, H. pylori infection leads to the de novo synthesis of ROS in epithelial cells in both in vitro and in vivo models of infection [66,–68]. These high concentrations of ROS have been linked to various forms of DNA damage, including oxidative DNA damage, DNA deamination, and alkylation [37, 69, 70].
The Big Blue transgenic mouse is a useful system in which to investigate DNA damage in various tissues, including the stomach. One study using these mice demonstrated that H. pylori infection was linked to high levels of DNA damage [71, 72]. Within gastric epithelial cells from these mice, AT to GC and GC to TA transversions were increased fourfold following 6 months of infection compared with cells isolated from uninfected control mice [72]. In the same model, Big Blue mice deficient for OGG1 developed significantly decreased levels of inflammation compared with WT controls after 6 months of infection with H. pylori strain SS1 [72]. Consequently, the transversions oberved in H. pylori-infected WT mice were no longer present in infected OGG1−/− mice [72]. These data clearly demonstrate the highly deleterious effects of ROS and the direct relationship between chronic inflammation and oxidative stress [72].
In addition to base-pair transversions, H. pylori infection induces DNA double-strand breaks, leading to genomic instabilty and neoplastic transformation [73]. Our laboratory determined recently that H. pylori infection leads to significantly increased levels of phospho-histone H2AX.2, a marker of double-strand breaks that can be assessed by flow cytometry or immunohistochemistry, in gastric epithelial cells.
Under normal circumstances, DNA damage is repaired by a number of different mechanisms depending on the type of damage sustained. H. pylori, however, maintains the ability to disrupt DNA damage repair pathways within the host. For example, H. pylori inhibits the base excision repair pathway [74, 75]. Whereas the exact mechanism of inhibition has yet to be determined, infection is associated with significantly decreased levels of DNA mismatch repair proteins, including MSH2, MSH6, and MLH1 [74]. Additional studies have also found marked decreases in MSH3, PMS1, and PMS2, other proteins involved in mismatch repair [75]. Intriguingly, dysregulation of these same proteins has been linked to colon carcinogenesis, providing the impetus for similar studies to be performed in gastric cancer models [74].
H. pylori has also been shown to inhibit the expression of APE-1 mRNA [75], which is involved in base excision repair of nuclear and mitochondrial DNA [75]. Another study demonstrated that ROS accumulation, as a result of H. pylori infection, increased APE-1 expression [76], suggesting that infected cells have a higher capacity to repair oxidative DNA damage. Further studies are expected to provide important insights into these complex relationships.
APE-1 becomes acetylated during H. pylori infection, which leads to inhibition of Bax expression and decreased p53-mediated apoptosis [77]. In our studies, we have determined that a subpopulation of gastric epithelial cells within H. pylori-infected stomachs accumulates high levels of DNA damage but remains resistant to apoptosis [21, 78, 79]; thus, genetically damaged cells are able to persist and proliferate, leading to carcinogenesis (Fig. 2).
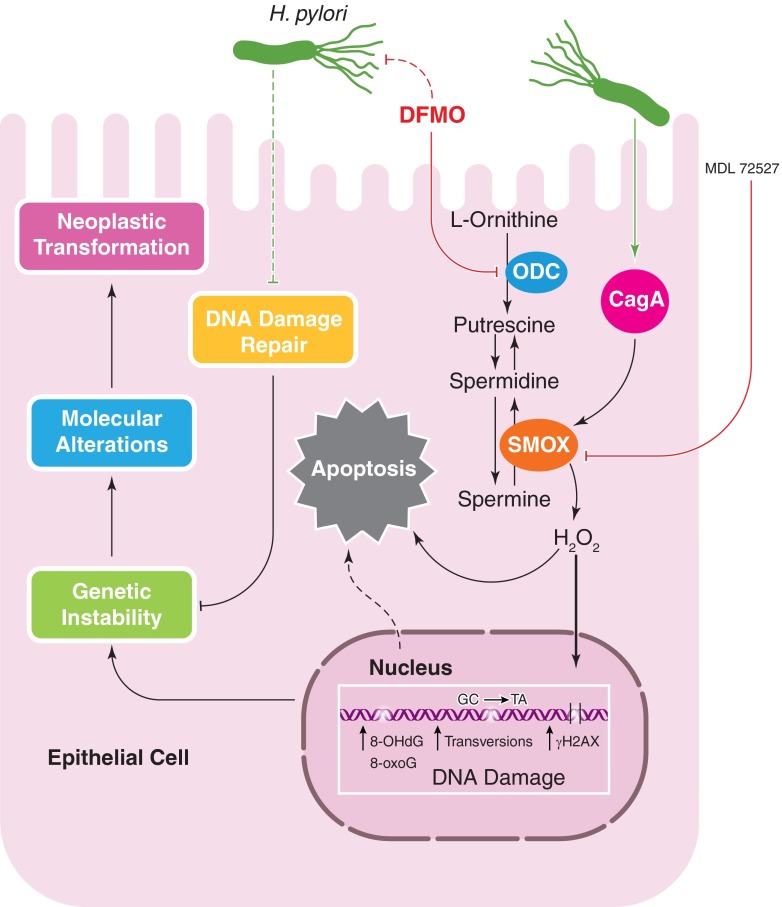
H. pylori CagA induces SMOX expression, leading to H2O2 production, which causes ROS accumulation and apoptosis. MDL 72527 inhibits SMOX and attenuates the deleterious effects of its activation. DFMO is a specific inhibitor of ODC, which also serves to decrease SMOX-mediated DNA damage and oxidative stress. DFMO exerts an inhibitory effect on H. pylori. ROS lead to various forms of DNA damage, including transversions, oxidative DNA damage (8-OHdG and 8-oxoG), and double-strand breaks (γH2AX). This, combined with H. pylori-mediated inhibition of DNA damage repair, leads to genomic instability within gastric epithelial cells. Subsequently, molecular alterations and neoplastic transformations occur, lowering the threshold for gastric carcinogenesis.
Within cancer research, the use of biomarkers is an important tool for the early detection of cancer and for determining prognosis. As DNA damage is a hallmark of carcinogenesis, signatures of DNA damage, specifically 8-oxoG, may serve as powerful biomarkers for gastric cancer [5, 80]. 8-oxoG and 8-OHdG assays can measure nucleotide adducts that reflect oxidative DNA damage [80] and serve as direct markers of DNA damage and proxy markers for oxidative stress in vivo [14, 21, 80]. H. pylori causes an increase in ROS from immune cell infiltration and de novo synthesis by epithelial cells (Fig. 2) [5, 6, 10, 12, 21, 34, 66, 67, 69]. Oxidative stress leads to oxidative DNA damage, making 8-oxoG an ideal candidate biomarker for detecting and assessing prognosis of gastric cancer [5, 80, 81].
Polyamine synthesis and metabolism
Polyamines are polycationic amino acids that exert pleiotropic effects within host cells, including inhibition of cytokine production and modulation of apoptosis [11]. L-Arginine is processed via the Arg2-ODC pathway. As discussed previously, H. pylori induces Arg2 and ODC expression in macrophages, leading to increased macrophage apoptosis [82].
Within gastric epithelial cells, H. pylori exerts its effects on a different enzyme within the same pathway, SMOX, leading to deleterious effects. H. pylori increases SMOX, which is responsible for the back-conversion of spermine to spermidine (Fig. 2) [14, 21]. H2O2 is a byproduct of this reaction and can be measured directly with the Amplex Red enzyme assay, in addition to flow cytometry, using DCFDA [14, 47]. Increased H2O2 leads to mitochondrial membrane depolarization and the activation of caspase-mediated apoptosis [21, 47]. This effect is more pronounced following infection with cagA+ strains compared with isogenic mutants lacking cagA [14, 21]. Individuals infected with strains of H. pylori that harbor cagA and have a functional cag secretion system have significantly higher levels of SMOX protein within gastric epithelial cells compared with persons infected with cag− strains. Additionally, in human subjects, patients harboring strains with a functional cag secretion system exhibited higher levels of SMOX when assessed by flow cytometry or immunohistochemistry [83].
Concomitant with increased SMOX expression, there is a corresponding increase in oxidative DNA damage and apoptosis in vitro and in vivo [14, 21]. In vitro, small interfering RNA knockdown of SMOX ameliorates H. pylori-induced DNA damage [14, 21]. We have also demonstrated that MDL 72527 (Fig. 2), an inhibitor of polyamine oxidation, prevents DNA damage in gastric epithelial cells in vitro, caused by H. pylori infection or transfection of a plasmid expressing CagA [14, 21]. In parallel, we have found that Mongolian gerbils treated with MDL 72527 developed decreased levels of H2O2 production and DNA damage and less dysplasia and carcinoma.
Moreover, our data also show that inhibition of ODC, the rate-limiting enzyme for polyamine synthesis, with DFMO, caused decreased DNA damage and marked attenuation in the development of dysplasia and gastric adenocarcinoma in the gerbil model of H. pylori infection. Similarly, mice that are heterozygous for ODC (homozygous deletion is lethal) also exhibit decreased colonization and gastritis in our studies.
SMOX may ultimately prove to be a druggable target for gastric cancer treatment, but currently, there is no specific or potent inhibitor available for human use. It should be noted that eradication of H. pylori is associated with reduced SMOX expression levels in the human stomach when compared with pre-eradication levels [14]. However, H. pylori antibiotic-based eradication success rates are in the range of 80% in areas of the world where gastric cancer is common, such as Latin America [84, 85]. Thus additional strategies are needed, particularly because eradication of H. pylori does not appear to be useful once precancerous lesions have developed; this subject is thoroughly discussed in the accompanying review by Dalal and Moss. Specifically, we hypothesize that DNA damage in infected patients can be reduced and potentially, reversed by blocking the polyamine-driven oxidative stress and contend that use of DFMO may be an effective strategy in patients with atrophic gastritis and intestinal metaplasia that are at the highest risk for neoplastic transformation. DFMO has been used as a chemopreventive agent to reduce colonic adenomas [86], and there is a track record of its safety for chronic use in humans [86,–88]. Thus, DFMO, with or without antibiotic treatment, should be considered, and studies are planned from our group to assess this in high-risk human populations in Latin America.
An important, additional benefit of DFMO stems from the discovery that DFMO treatment leads to depletion of the tetrahydrofolate substrate needed for thymidine synthesis in a colon cancer model, which occurs by induction of the enzyme S-adenosylmethionine decarboxylase [89]; this may help explain the ability of DFMO to reduce tumor cell growth that has been noted for decades [90]. Thus, the ability of DFMO to exert this effect in H. pylori-infected gastric epithelial cells needs to be investigated. Finally, we have reported that DFMO itself can have antimicrobial effects (Fig. 2), as it reduced H. pylori growth rate in culture and attenuated CagA levels and induction of IL-8 upon coculture of treated H. pylori strains with gastric epithelial cells [91].
The antioxidant response
The antioxidant response is an important component for protection of cells from oxidative damage. HO-1 is a potent antioxidant and anti-inflammatory enzyme that is responsible for the degradation of heme [92]. It is known to exert wide-ranging, protective functions across many disease models [92]. HO-1 expression is induced by the presence of NO, leading to decreased IL-8 expression and inflammation [41]. Within an H. pylori infection model, exogenous enhancement of HO-1 expression prevents CagA phosphorylation in gastric epithelial cells, significantly attenuating H. pylori virulence and pathogenesis [93]. CagA phosphorylation is an important step in unleashing detrimental effects of CagA within host cells [94, 95]. Phospho-CagA is likely involved in inducing ROS production, but further studies using in vitro and in vivo models are needed to determine its role in the stimulation of ROS generation.
To the detriment of an infected host, the protective role of HO-1 in gastric epithelial cells is almost completely lost during H. pylori infection. As cagA+ strains can down-regulate HO-1, it is apparent that H. pylori can enhance its own pathogenesis through this mechanism [41]. HO-1 expression depends on NF-κB signaling [41], and in the specific case of NO-induced NF-κB activation, H. pylori can disrupt this process [41]. The loss of this component of NF-κB signaling may be important. NF-κB activation can have many effects, and these may vary with acute and chronic time-points; whereas its activation is proinflammatory, its inhibition has been associated with increased ROS, apoptosis and necrosis, and DNA damage and more rapid development of preneoplasia in vivo [96]. The loss of an effective antioxidant response to H. pylori-induced oxidative stress is another potential, contributing factor to the progression to gastric carcinoma.
HO-1 is not the only component of the antioxidant response that is inhibited by H. pylori infection in the progression to gastric cancer. GPX3 functions to reduce oxyradicals to H2O, thereby protecting cells from oxidative damage [97]. GPX3 expression is silenced by DNA hypermethylation in gastric carcinoma and is associated with tumor invasion and lymph node metastases [97]. As H. pylori is the strongest known risk factor for gastric cancer, GPX3 inhibition could potentially be caused—directly or indirectly—by the bacterium or occur as a consequence of inflammation. Further studies are needed to determine fully the role of H. pylori in dysregulating the oxidative stress response.
MICROBIOTA AND ENVIRONMENTAL FACTORS
In the past decade, the role of the human microbiome has become increasingly scrutinized, especially within the context of gastrointestinal cancer [98, 99], and H. pylori-induced neoplasia is no different [98]. A recent study suggests that the severity of gastric inflammation in WT C57BL/6 mice infected with H. pylori is directly related to the preinfection microbiota [100]. The specific type of Clostridium species present in the gastric microbiome determines the degree of CD4+ T cell infiltration and IFN-γ expression [100]. Whereas few studies have examined the human microbiome and its relationship to H. pylori pathogenesis, analysis of the gastric microbiota could potentially be used as a tool to determine the risk of developing advanced gastric lesions of dysplasia and carcinoma [100, 101]. It is established that gastric atrophy, with associated hypochlorhydria, is coupled with gastric carcinogenesis. Changes in the microbiota over time with chronic H. pylori infection and development of atrophy may represent another contributing factor to the finding that gastric cancer is a disease of middle to old age that is not present in childhood and is rare in younger adults. This may also provide new insights into why inflammation alone is not sufficient to cause gastric cancer, as gastritis is universal with H. pylori infection, but this progresses to cancer in the minority of cases.
The composition of an individual's diet may also play a role in H. pylori pathogenesis and gastric cancer risk. A diet high in salt or red meat exacerbates cancer risk in humans infected with H. pylori, whereas fruit and vegetable intake decreases risk [102, 103]. Mongolian gerbils maintained on an iron-depleted diet also develop increased H. pylori-induced inflammation, dysplasia, and carcinoma [104]. Other environmental factors, such as smoking and alcohol use, may contribute to cancer risk, but the data remain inconclusive [103, 105].
CLINICAL PRIORITIES
Basic research has provided a plethora of information regarding the mechanisms underlying H. pylori infection and gastric cancer development. However, to use this information effectively, we should prioritize each finding by its clinical relevance and potential for application, as many questions remain unanswered (Table 1). We suggest that targeting the polyamine synthesis pathways, using specific small molecule inhibitors, is the most promising, new therapeutic approach currently available for testing. DFMO has proven to be an effective therapeutic in other models of cancer and in murine models of H. pylori infection, as discussed in this review. We contend that clinical trials testing the efficacy of DFMO in preventing or possibly treating gastric cancer, especially in high-risk regions, are warranted, and we hope that they will be undertaken as soon as possible.
Table 1.
Lessons learned | Outstanding questions |
---|---|
H. pylori virulence factors, especially CagA and VacA, contribute to oxidative stress. H. pylori alters the ratio of T cell subtypes in favor of an augmented Treg response.H. pylori infection leads to increased oxidative DNA damage and increased DNA transversions. Also, H. pylori inhibits the mismatch repair and base excision repair pathways.H. pylori infection increases both ODC and SMOX expression in mice. Inhibition of either of these enzymes decreases inflammation, oxidative stress, and DNA damage. The preinfection microbiota appears to affect H. pylori-induced pathology. | Who warrants therapy for H. pylori infection given that a minority of persons develops cancer, and certain H. pylori strains are inversely related to other diseases? |
What are the mechanisms underlying the impact of CagA phosphorylation on the clinical outcome of infection? | |
Does more robust phosphorylation correlate with increased oxidative stress and/or DNA damage? | |
Do CagA and VacA collaborate to augment the production of ROS and what is the mechanism of such an interaction? | |
Are there other H. pylori virulence factors that contribute to the generation of oxidative stress and chronic inflammation? | |
Do we need new therapies directed against H. pylori? | |
What impact does H. pylori infection have on macrophage differentiation into M1, M2 and Mreg subtypes, and dendritic cell responses, particularly in humans, and how does this impact inflammation and gastric carcinogenesis? | |
Could targeting T cell differentiation to increase the ratio of Tregs to Th1/Th17 cells be a plausible strategy to alleviate H. pylori-induced inflammation, or would this be counter-productive by leading to higher levels of colonization and thus more induction of oxidative stress in gastric epithelial cells? | |
Does eradication of H. pylori using antimicrobial agents inhibit DNA damage once precancerous histology has developed? | |
Is inhibition of ODC a more effective strategy to reduce DNA damage and prevent gastric carcinogenesis? | |
Is augmentation of antioxidant responses during H. pylori infection feasible for prevention of neoplasia? | |
What bacterial genera, exogenous of Helicobacter, populate the human stomach? | |
Which genera and species impact more severe clinical outcomes? | |
Can the microbiome be targeted either alone or in conjunction with H. pylori to lower gastric cancer risk? | |
Can concepts learned from studying H. pylori and gastric cancer be applied easily to other types of cancer that arise from inflammatory foci? |
Moreover, as this review demonstrates, gastric cancer only occurs in 1–3% of those infected with H. pylori. We believe that it is critical for clinicians and researchers to determine risk factors for the development of gastric cancer. In doing so, together, we can better determine the need to eradicate H. pylori infection and the need to begin chemopreventive measures. As the field stands, improved risk stratification in infected individuals is a top priority. Our work indicates that polyamine synthesis and oxidation are key elements in gastric carcinogenesis by generation of oxidative stress-induced DNA damage in gastric epithelial cells and that analysis of this pathway in high-risk populations will provide key insights into causation and selection of subjects for chemoprevention.
CONCLUSIONS
H. pylori infection is indisputably associated with chronic inflammation, which generates pathologic levels of oxidative stress over prolonged periods of time [38, 43, 70, 73, 75]. Our laboratory and others' have implicated the generation of ROS as an important mediator of DNA damage, and we hypothesize that this lays the foundation for gastric carcinogenesis. Several pathways, including polyamine synthesis and catabolism, have been identified as having potentially detrimental effects on gastric carcinogenesis. Further studies are needed to parse out the molecular signaling events that lead to such consequences, which will ultimately identify new targets for therapeutic intervention and improve risk stratification for H. pylori-infected patients. However, human trials testing the efficacy of DFMO represent a critical means to determine if H. pylori-induced DNA damage and its carcinogenic effects can be halted or even reversed.
ACKNOWLEDGMENTS
This work was supported by the U.S. National Institutes of Health Grants R01DK053620;, R01AT004821;, P01CA028842;, and P01CA116087; (to K.T.W.) and R01DK58587;, R01CA77955;, and P01CA116087 (to R.M.P.); the Vanderbilt University Digestive Disease Research Center, supported by grants P30DK058404; and UL1RR024975 (Vanderbilt Clinical & Translational Science Awards, Pilot Project to K.T.W.); and a Merit Review Grant 1I01BX001453 from the Office of Medical Research, Department of Veterans Affairs (to K.T.W.). D.M.H. was supported by U.S. National Institutes of Health Grant 5T32GM008554. D.M.H and K.T.W. received support from the Thomas F. Frist, Sr., endowment (awarded to K.T.W.).
SEE CORRESPONDING ARTICLE ON PAGE 213
- 8-OHdG
- 8-hydroxy-2′-deoxyguanosine
- 8-oxoG
- 8-oxoguanosine
- APE-1
- apurinic/apyrimidinic endonuclease 1
- Arg2
- arginase II
- CagA/cagA
- cytotoxin-associated gene A
- DC
- dendritic cell
- DCFDA
- 7′-dichlorodihydrofluorescein diacetate
- DFMO
- α-difluoromethylornithine
- EPIYA
- Glu-Pro-Ile-Tyr-Ala
- GGT
- γ-glutamyl transferase
- GPX3
- glutathione peroxidase 3
- HO-1
- heme oxygenase 1
- LRP1
- lipoprotein receptor-related protein 1
- M1
- classically activated macrophage
- M2
- alternatively activated macrophage
- Mreg
- regulatory macrophage
- NapA
- neutrophil-activating protein A
- ODC
- ornithine decarboxylase
- OGG1
- 8-oxoguanosine DNA glycosylase
- RNS
- reactive nitrogen species
- ROS
- reactive oxygen species
- SMOX
- spermine oxidase
- T4SS
- type IV secretion system
- Treg
- regulatory T cell
- VacA/vacA
- vacuolating cytotoxin A
- WT
- wild-type
REFERENCES
Articles from Journal of Leukocyte Biology are provided here courtesy of The Society for Leukocyte Biology
Full text links
Read article at publisher's site: https://doi.org/10.1189/jlb.4bt0214-099r
Read article for free, from open access legal sources, via Unpaywall:
https://europepmc.org/articles/pmc4101087
Citations & impact
Impact metrics
Citations of article over time
Article citations
Dual roles of inflammatory programmed cell death in cancer: insights into pyroptosis and necroptosis.
Front Pharmacol, 15:1446486, 27 Aug 2024
Cited by: 0 articles | PMID: 39257400 | PMCID: PMC11384570
Review Free full text in Europe PMC
Unravelling the role of intratumoral bacteria in digestive system cancers: current insights and future perspectives.
J Transl Med, 22(1):545, 07 Jun 2024
Cited by: 0 articles | PMID: 38849871
Review
Platelet-lymphocyte ratio predicts chemotherapy response and prognosis in patients with gastric cancer undergoing radical resection.
Front Oncol, 14:1279011, 06 Mar 2024
Cited by: 0 articles | PMID: 38511137 | PMCID: PMC10951101
Effects of RAD50 SNP, sodium intake, and H. pylori infection on gastric cancer survival in Korea.
Gastric Cancer, 27(2):210-220, 09 Dec 2023
Cited by: 0 articles | PMID: 38070008
Review
Mucin Glycans: A Target for Cancer Therapy.
Molecules, 28(20):7033, 11 Oct 2023
Cited by: 5 articles | PMID: 37894512 | PMCID: PMC10609567
Review Free full text in Europe PMC
Go to all (49) article citations
Similar Articles
To arrive at the top five similar articles we use a word-weighted algorithm to compare words from the Title and Abstract of each citation.
At the bedside: Helicobacter pylori, dysregulated host responses, DNA damage, and gastric cancer.
J Leukoc Biol, 96(2):213-224, 13 May 2014
Cited by: 2 articles | PMID: 24823809 | PMCID: PMC4101088
Review Free full text in Europe PMC
Chronic inflammation and oxidative stress: the smoking gun for Helicobacter pylori-induced gastric cancer?
Gut Microbes, 4(6):475-481, 28 Jun 2013
Cited by: 70 articles | PMID: 23811829 | PMCID: PMC3928159
Review Free full text in Europe PMC
Carcinogenic bacterial pathogen Helicobacter pylori triggers DNA double-strand breaks and a DNA damage response in its host cells.
Proc Natl Acad Sci U S A, 108(36):14944-14949, 06 Sep 2011
Cited by: 200 articles | PMID: 21896770 | PMCID: PMC3169107
Molecular Pathogenesis of Helicobacter pylori-Related Gastric Cancer.
Gastroenterol Clin North Am, 44(3):625-638, 07 Jul 2015
Cited by: 17 articles | PMID: 26314672
Review
Funding
Funders who supported this work.
BLRD VA (1)
Grant ID: I01 BX001453
Merit Review (1)
Grant ID: 1I01BX001453
NCCIH NIH HHS (2)
Grant ID: R01 AT004821
Grant ID: R01AT004821
NCI NIH HHS (6)
Grant ID: R01 CA077955
Grant ID: P01 CA028842
Grant ID: P01CA116087
Grant ID: P01 CA116087
Grant ID: R01CA77955
Grant ID: P01CA028842
NCRR NIH HHS (2)
Grant ID: UL1RR024975
Grant ID: UL1 RR024975
NIDDK NIH HHS (6)
Grant ID: R01 DK058587
Grant ID: P30 DK058404
Grant ID: P30DK058404
Grant ID: R01DK053620
Grant ID: R01DK58587
Grant ID: R01 DK053620
NIGMS NIH HHS (2)
Grant ID: T32 GM008554
Grant ID: 5T32GM008554
Office of Medical Research, Department of Veterans Affairs
U.S. National Institutes of Health (7)
Grant ID: P01CA028842
Grant ID: R01AT004821
Grant ID: R01DK58587
Grant ID: 5T32GM008554
Grant ID: P01CA116087
Grant ID: R01CA77955
Grant ID: R01DK053620
Vanderbilt Clinical & Translational Science Awards
Vanderbilt University Digestive Disease Research Center (2)
Grant ID: UL1RR024975
Grant ID: P30DK058404