Abstract
Free full text

Mitochondrial pathobiology in ALS
Abstract
Amyotrophic lateral sclerosis (ALS) is the third most common human adult-onset neurodegenerative disease. Some forms of ALS are inherited, and disease-causing genes have been identified. Nevertheless, the mechanisms of neurodegeneration in ALS are unresolved. Genetic, biochemical, and morphological analyses of human ALS as well as cell and animal models of ALS reveal that mitochondria could have roles in this neurodegeneration. The varied functions and properties of mitochondria might render subsets of selectively vulnerable neurons intrinsically susceptible to cellular aging and stress and overlying genetic variations. Changes occur in mitochondrial respiratory chain enzymes and mitochondrial programmed cell death proteins in ALS. Transgenic mouse models of ALS reveal possible principles governing the biology of neurodegeneration that implicate mitochondria and the mitochondrial permeability transition pore. This paper reviews how mitochondrial pathobiology might contribute to the mechanisms of neurodegeneration in ALS.
Synposis of human ALS
ALS is a progressive and severely disabling neurological disease in humans characterized by initial muscle weakness, and then muscle atrophy, spasticity, and eventual paralysis and death typically 3 to 5 years after diagnosis (Rowland and Shneider 2001). The cause of the spasticity, paralysis and death is progressive degeneration and elimination of upper motor neurons in cerebral cortex and lower motor neurons in brainstem and spinal cord (Fig. 1) (Rowland and Shneider 2001; Sathasivam et al. 2001). Degeneration and loss of spinal and neocortical interneurons also occur in human ALS (Stephens et al. 2006; Maekawa et al. 2004). More than 5,000 people in the USA are diagnosed with ALS each year (ALS Association, http://www.alsa.org), and, in parts of the United Kingdom, three people die every day from some form of motor neuron disease (http://www.mndassociation.org). Other than life support management, no effective treatments exist for ALS (Zoccolella et al. 2009).
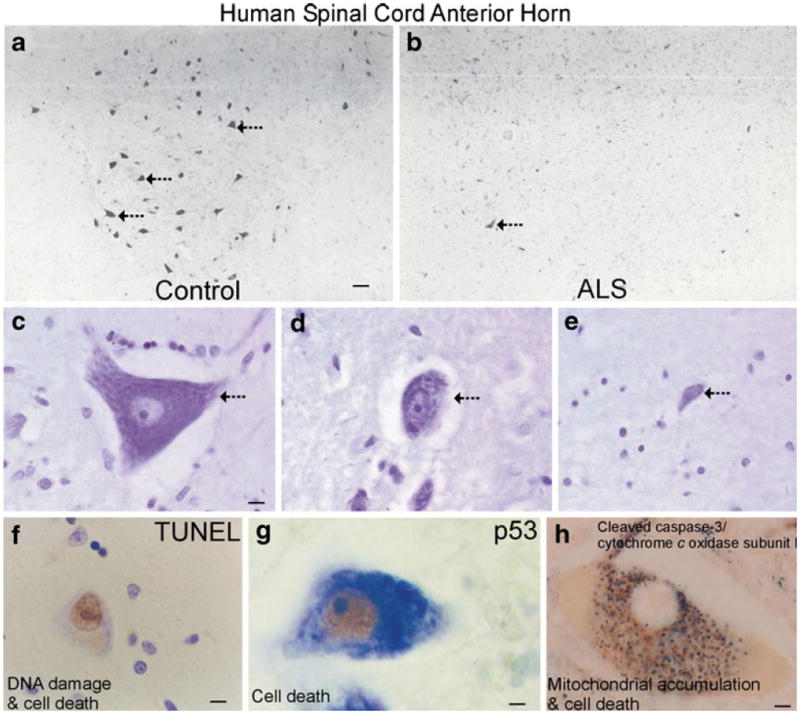
Motor neurons in spinal cord degenerate in people with ALS. a In normal control individuals, the anterior horns of the spinal cord contain many large, multipolar motor neurons (large dark cells, hatched arrows). b In ALS cases, the anterior horn is depleted of large neurons (dark cell, hatched arrow) and remaining neurons are atrophic. These attritional chromatolytic motor neurons display a dark condensed nucleus as seen microscopically. Scale bar in A=76 μm (same for b). c, d, and e Nissl staining shows that the degeneration of motor neurons in human familial ALS is characterized by shrinkage and progressive condensation of the cytoplasm and nucleus. The motor neuron in c (arrow) appears normal. It has a large, multipolar cell body and a large nucleus containing reticular network of chromatin and a large nucleolus. Scale bar=7 μm (same for d,e).The motor neuron in d (arrow) has undergone severe somatodentritic attrition. The motor neuron in e is at near endstage degeneration (arrow). The cell has shrunken to about 10% of normal size and has become highly condensed. The cell in e is identified as a residual motor neuron based the large nucleolus and residual large Nissl bodies. f Cell death assays (e.g., TUNEL) identify subsets of motor neurons in the process of DNA fragmentation. Nuclear DNA fragmentation (brown labeling) occurs in motor neurons in people with ALS as the nucleus condenses and the cell body shrinks. Motor neurons in the somatodendritic attrition stage (Martin 1999) accumulate DNA double strand breaks. Scale bar=7 μm. g In individuals with ALS, the tumor suppressor p53 accumulates in the nucleus (brown labeling) of motor neurons. Scale bar=5 μm, h Degenerating motor neurons in human ALS are immunopositive for cleaved caspase-3 (black-dark green labeling) in the somatodendritic attrition stage (Martin 1999). Motor neurons show prominent accumulation around the nucleus (pale circle) of discrete mitochondria (brown-orange labeling, detected with antibody to cytochrome c oxidase subunit I) exhibiting little light microscopic evidence for swelling. Scale bar=5 μm
It is still not understood why specific neuronal populations are selectively vulnerable in ALS, such as certain somatic motor neurons and interneurons (Rowland and Shneider 2001; Sathasivam et al. 2001; Martin 2010a). The molecular pathogenesis of ALS is understood poorly, contributing to the lack of appropriate target identification and effective mechanism-based therapies to treat even the symptoms of this disease. At least two forms of ALS exist: idiopathic (sporadic) and heritable (familial). Most people with ALS have no known genetic contributions and are designated sporadic. Aging is a strong risk factor for ALS because the average age of onset is 55 (ALS Association, www.alsa.org). Familial forms of ALS have autosomal dominant or autosomal recessive inheritance patterns and make up ~10% or less of all ALS cases. ALS-linked mutations occur in the genes (Table 1) encoding SOD1 (ALS1), Alsin (ALS2), senataxin (ALS4), fused in sarcoma (FUS, ALS6), vesicle associated membrane protein (VAMP/synaptobrevin)-associated protein B (VAPB, ALS8), p150 dynactin (DCTN1), TAR-DNA binding protein (TADBP or TDP43, ALS10) and optineurin (ALS12) (Deng et al. 1993; Schymick et al. 2007; Kabashi et al. 2008; Vance et al. 2009; Maruyama et al. 2010). Variations in the phosphoinositide phosphatase FIG4 gene cause ALS11 (Chow et al. 2009). Several other genes are believed to be susceptibility factors for ALS (Table 1) (Saeed et al. 2006; Elden et al. 2010).
Table 1
Mutant/polymorphic genes linked to ALS
Locus | Inheritance | Gene | Protein Name & Function |
---|---|---|---|
ALS1/21q22 | autosomal dominant (adult onset) | SOD1 | Cu/Zn superoxide dismutase; dismutation of supeoxide |
ALS2/2q33.2 | autosomal recessive (juvenile onset primary lateral sclerosis) | Alsin | Alsin/guanine exchange factor for RAB5A and Rac1 |
ALS4/9q34 | autosomal dominant (adult onset) | SETX | Senataxin/helicase; RNA processing |
ALS6/16q12 | autosomal recessive (adult onset) | FUS | Fused in sarcoma, component of heterogeneous nuclear ribonuclear protein complex; RNA/DNA binding protein |
ALS8/20q13.33 | autosomal dominant (adult onset) | VAPB | VAMP-associated protein B/part of SNARE complex |
2q13 | autosomal dominant (adult onset, atypical ALS) | DCTN1 | Dynactin p150glued/axonal transport, link between dynein and microtubule network |
ALS10/1p36.22 | autosomal dominant & sporadic (adult onset) | TARDBP | TAR DNA binding protein, DNA and RNA binding protein; regulates RNA splicing |
ALS11/6q21 | autosomal recessive (adult onset) | FIG4 | FIG4 homolog, SAC1 lipid phosphatase domain containing; regulates phosphotidylinositol turnover |
ALS12/10p13 | autosomal recessive & dominant (adult onset) | OPTN | Optineurin; inhibits NFκB activation |
14q11.1-q11.2 | susceptibility factor | ANG | Angiogenin; angiogenesis; stimulates production of rRNA |
22q12.2 | susceptibility factor | NEFH | Neurofilament, heavy polypeptide; neurofilament subunit |
12q12-q13 | susceptibility factor | PRPH | Peripherin; intermediate filament formation |
5q13 | susceptibility factor | SMN1/SMN2 | Survival motor neuron; RNA processing |
7q36.6 | susceptibility factor | DPP6 | Dipeptidyl-peptidase 6; S9B serine protease, binds voltage-gated potassium channels |
7q21.3 | susceptibility factor for sporadic | PON1 | Paraoxonase; organophasphate pesticide/nerve agent detoxification |
12q24.1 | susceptibility factor for sporadic | ATXN2 | Ataxin-2; RNA processing |
Evidence for mitochondrial dysfunction in human ALS
Human ALS is associated with mitochondrial abnormalities. Structural abnormalities in mitochondria are seen by electron microscopy (EM) in skeletal muscle, liver, spinal motor neurons and motor cortex of ALS patients (Sasaki and Iwata 1999; Menzies et al. 2002). A mutation in cytochrome c oxidase subunit I was found in a patient with a motor neuron disease phenotype (Comi et al. 1998). Another patient with motor neuron disease had a mutation in a mitochondrial tRNA gene (Borthwick et al. 2006). One type of mitochondrial DNA (mtDNA) mutation, called the common mtDNA deletion (mtDNA4977), is found non-uniformly within different human brain areas in aging; the highest levels are detected in the striatum and substantia nigra (Soong et al. 1992; Corral-Debrinski et al. 1992). However, no significant accumulation of the 5 kb common deletion in mtDNA has been found by single-cell analysis of motor neurons from sporadic ALS cases compared to controls (Mawrin et al. 2004). Overall, there is a lack of strong direct evidence for mitochondrial abnormalities participating in disease-causing mechanisms of human ALS, despite many associational/correlative data from human and animal/cell models.
Intracellular Ca2+ abnormalities and excitotoxicity are suspected links to mitochondrial dysfunction and oxidative stress in ALS (Beal 2002; Martin 2010a, b). Mitochondria regulate cytoplasmic Ca2+ levels (Babcock and Hille 1998; Nicholls 2002; Zorov et al. 2007). Electron microscopy on skeletal muscle biopsies of people with sporadic ALS shows changes indicative of elevated Ca2+ in motor neuron terminals, with some mitochondria showing an augmented Ca2+ signal (Siklos et al. 1996). Excitotoxicity has been implicated in the pathogenesis of ALS for a long time (Rothstein et al. 1992) and is another possible mechanism of motor neuron damage in ALS (Heath et al. 2002; Martin 2010a). Many sporadic ALS patients have reduced levels of synaptosomal high-affinity glutamate uptake (Rothstein et al. 1992) and astroglial glutamate transporter EAAT2 (excitatory amino acid transporter 2 or GLT1) in motor cortex and spinal cord (Rothstein et al. 1995). These changes could likely be secondary; however, reductions in levels of activity of EAAT2 in spinal cord might increase the extracellular concentrations of glutamate at synapses on motor neurons. Motor neurons might be particularly sensitive to glutamate excitotoxicity because they have a low proportion of GluR2-edited or under-edited AMPA subtype glutamate receptor on their surfaces, predisposing these cells to risk of excess Ca2+ entry and mitochondrial perturbations (Heath et al. 2002; Kwak and Kawahara 2005). Cell culture studies show that excess glutamate receptor activation in neurons can cause increased intracellular Ca2+, enhanced mitochondrial reactive oxygen species (ROS) production, bioenergetic failure, and mitochondrial trafficking abnormalities (Chang and Reynolds 2006). Ca2+-induced generation of ROS in brain mitochondria is mediated by mitochondrial permeability transition (Hansson et al. 2008). Motor neurons are particularly affected by inhibition of mitochondrial metabolism which can cause elevated cytosolic Ca2+ levels, excitability, and oxidative stress (Bergmann and Keller 2004). Recent data have provided a fresh prespective on ALS pathogenesis by demonstrating that motor neurons in transgenic (tg) ALS mice have faulty synaptic inhibition long before disease symptoms emerge, suggesting that inhibitory glycine receptor and interneuron abnormalities are upstream mechanisms while, possibly, excitotoxicity is secondary (Chang and Martin 2009; Chang and Martin 2011). While many drugs targeting excitotoxicity as a mechanism have failed in human ALS clinical trials, the drug riluzole, a blocker of tetrodotoxin-sensitive sodium channels, is currently approved by the Food and Drug Administration for ALS treatment with marginal efficacy (Zoccolella et al. 2009).
Markers of oxidative stress and ROS damage are elevated in postmortem human ALS tissues (Beal 2002). In sporadic and familial ALS, protein carbonyls are elevated in motor cortex (Ferrante et al. 1997). Tyrosine nitration is elevated in human ALS nervous tissues (Abe et al. 1995; Beal et al. 1997; Sasaki et al. 2000). Studies of respiratory chain enzyme activities are discrepant. Experiments on autopsy tissue have shown increases in complex I, II, and III activities in vulnerable and non-vulnerable brain regions in patients with familial ALS-mutant SOD1 (Browne et al. 1998), but experiments by others show decreased complex IV activity in spinal cord ventral horn (Borthwick et al. 1999) and skeletal muscle (Vielhaber et al. 2000) of sporadic ALS cases. In sporadic ALS skeletal muscle, reductions in activity of respiratory chain complexes with subunits encoded by mtDNA are associated with reduced mtDNA content (Vielhaber et al. 2000) and decreased nitric oxide synthase (NOS) levels (Soraru et al. 2007). Alterations in skeletal muscle mitochondria are progressive (Echaniz-Laguna et al. 2006) and could be intrinsic to skeletal muscle and disease-causing, as suspected in human SOD1 tg mice (Wong and Martin 2010), rather than merely due to neurogenic atrophy, as assumed commonly.
Mitochondrial-orchestrated programmed cell death (PCD) involving p53 has been implicated in human ALS
PCD appears to contribute to the selective degeneration of motor neurons in human ALS, albeit seemingly as a non-classical form differing from apoptosis (Fig. 1) Martin 1999; Martin and Liu 2004; Martin 2010a). Motor neurons appear to pass through sequential stages of chromatolysis, suggestive of initial axonal injury (Lieberman 1971), somatodendritic attrition without extensive cytoplasmic vacuolation, and then nuclear DNA fragmentation, nuclear and chromatin condensation, and cell death (Fig. 1) (Martin 1999). Motor neurons in people that have died from sporadic ALS and familial ALS show the same type of degeneration (Martin 1999). This cell death in human motor neurons is idefined by genomic DNA fragmentation (determined by DNA agarose gel electrophoresis and in situ DNA nick-end labeling) and cell loss and is associated with accumulation of perikaryal mitochondria, cytochrome c, and cleaved caspase-3 (Fig. 1h) (Martin 1999; Martin and Liu 2004; Ginsberg et al. 2006). p53 protein also increases in vulnerable CNS regions in people with ALS, and it accumulates specifically in the nucleus of lower and upper motor neurons with nuclear DNA damage (Fig. 1g) (Martin 2000; Martin et al. 2000). This p53 is active functionally because it is phosphorylated at serine-392 and has increased DNA binding activity (Martin et al. 2000). However, the morphology of this cell death is distinct from classical apoptosis, despite the nuclear condensation (Martin et al. 1998; Martin 1999; Martin 2010a). Nevertheless, Bax and Bak1 protein levels are increased in mitochondria-enriched fractions of selectively vulnerable motor regions (spinal cord anterior horn and motor cortex gray matter), but not in regions unaffected by the disease (somatosensory cortex gray matter) (Martin 1999). In marked contrast, Bcl-2 protein is depleted severely in mitochondria-enriched fractions of affected regions and is sequestered in the cytosol (Martin 1999). Although these western blot results lacked direct specificity for motor neuron events (Martin 1999), subsequent immunohistochemistry (Martin and Liu 2004) and laser capture microdissection of motor neurons combined with mass spectroscopy-protein profiling (Ginsberg et al. 2006) have confirmed the presence of intact active caspase-3 in human ALS motor neurons.
Studies (Martin 1999; Martin 2000; Martin and Liu 2004) support the concept of an aberrant re-emergence of a mitochondrial-directed PCD mechanism, involving p53 activation and redistributions of mitochondrial cell death proteins, participating in the pathogenesis of motor neuron degeneration in human ALS (Fig. 1). The morphological and biochemical changes seen in human ALS are modeled robustly and faithfully at morphological and molecular levels in axonal injury/target deprivation (axotomy) models of motor neuron degeneration in adult rodents (Martin et al. 2000; Martin et al. 2005) but not in the current most commonly used human mutant SOD1 tg mouse models (Martin and Liu 2004; Martin et al. 2007). However, a new tg mouse expressing human mutant SOD1 only in skeletal muscle develops a motor neuron disease phenotype with morphological and biochemical changes very similar that those seen in human ALS motor neurons (Wong and Martin 2010).
Mitochondrial pathobiology in cell and mouse models of ALS
A common genetic mutation in human SOD1 that is linked to familial ALS (Table 1) is the substitution of glycine by alanine at position 93 (G93A) (Turner and Talbot 2008). SOD1 (also called Cu/Zn SOD) is a metalloenzyme of 153 amino acids (~16 kDa) that binds one copper ion and one zinc ion per subunit and is active as a ~32 kDa non-covalently linked homodimer (McCord and Fridovich 1969; Fridovich 1995). SOD1 is responsible for the detoxification and maintenance of intracellular O2•- concentration in the low femtomolar range by catalyzing its dismutation (McCord and Fridovich 1969; Fridovich 1995). SOD1 is ubiquitous (intracellular SOD concentrations are typically ~10-40 micromolar) in most tissues, possibly with highest levels in neurons (Rakhit et al. 2004).
Cell culture experiments reveal mitochondrial dysfunction in the presence of human mutant SOD1 (mSOD1) (Ferri et al. 2006). Expression of several mSOD1 variants increases mitochondrial superoxide levels and causes toxicity in rat primary embryonic motor neurons (Estévez et al. 1999), human neuroblastoma cells (Flanagan et al. 2002), and mouse neuroblastoma-spinal cord (NSC)-34 cells, a hybrid cell line with some motor neuron-like characteristics produced by fusion of motor neuron-enriched embryonic mouse spinal cord cells with mouse neuroblastoma cells (Bilsland et al. 2008).These responses can be attenuated by over-expression of MnSOD (Flanagan et al. 2002). ALS-mSOD1 variants, compared to human wild-type SOD1, associate more with mitochondria in NSC-34 cells and appear to form cross-linked oligomers that shift the mitochondrial GSH/GSSH ratio toward oxidation (Ferri et al. 2006).
Gurney and colleagues were the first to develop tg mice that express human mSOD1 (Gurney et al. 1994; Dal Canto and Gurney 1994; Chiu et al. 1995). Now, these mice are used widely as an animal model of ALS (Martin and Liu 2004; Turner and Talbot 2008). In the otiginal tg mice, human mSOD1 was expressed ubiquitously driven by its endogenous promoter in a tissue/cell non-selective pattern against a background of normal wildtype mouse SOD1 (Gurney et al. 1994). Effects of this human mutant gene in mice are profound. Hemizygous tg mice expressing high copy number of the G93A variant of human SOD1 become completely paralyzed and die at ~16–18 weeks of age (Gurney et al. 1994). G93A-mSOD1 mice with reduced transgene copy number have a much slower disease progression and die at ~8–9 months of age (Gurney et al. 1994; Martin et al. 2009).
Spinal motor neurons and interneurons in mice expressing G93Ahigh-mSOD1 undergo prominent degeneration; about 60%–80% of lumbar motor neurons are eliminated by end-stage disease (Chiu et al. 1995; Mohajeri et al. 1998; Martin et al. 2007). Subsets of spinal interneurons degenerate before motor neurons in G93Ahigh-mSOD1 (Martin et al. 2007; Chang and Martin 2009) and some are the glycinergic Renshaw cells (Chang and Martin 2009). Unlike the degeneration of motor neurons in human ALS (Martin 1999), motor neurons in G93Ahigh-mSOD1 mice do not degenerate with a morphology resembling any form of apoptosis or apoptosis-necrosis hybrids (Martin 2010a). The motor neurons degeneration seen in G93Ahigh-mSOD1 mice more closely resembles a prolonged necrotic-like cell death process (Martin et al. 2007) involving early-occurring mitochondrial damage, cellular swelling, and dissolution (Kong and Xu 1998; Bendotti et al. 2001; Jaarsma et al. 2001; Sasaki et al. 2004). Biochemically, the death of motor neurons in G93Ahigh-mSOD1 is characterized by cell body and mitochondrial swelling and formation of DNA single-strand breaks prior to double-strand breaks occurring in nuclear DNA and mtDNA (Martin et al. 2007). The motor neuron death in G93Ahigh-mSOD1 mice is independent of activation of caspases-1 and –3, and also appears to be independent of capsase-8 and AIF activation within motor neurons (Martin et al. 2007). Indeed, caspase-dependent and p53-mediated apoptosis mechanisms might be blocked actively in G93Ahigh-mSOD1 mouse motor neurons, possibly by up-regulation of inhibitors of apoptosis and changes in the nuclear import of proteins (Martin et al. 2007). More work is needed on the cell death and its mechanisms in G93Alow-mSOD1 mice, because these mice could be more relevant physiologically and preclinically to the human disease compared to G93Ahigh-mSOD1 mouse.
Mitochondrial disease has been implicated in the mechanisms of motor neuron degeneration in tg mSOD1 mouse models, but until recently, most evidence has been circumstantial. In different mSOD1 mouse models of ALS, mitochondria in spinal cord neurons exhibit prominebt structural pathology (Wong et al. 1995; Kong and Xu 1998; Bendotti et al. 2001; Jaarsma et al. 2001; Sasaki et al. 2004; Martin et al. 2007), and some of the mitochondrial degeneration occurs very early in the course of the disease (Bendotti et al. 2001; Martin et al. 2007). Mitochondrial microvacuolar damage in motor neurons is seen by electron microscopy at 4 weeks of age in G93Ahigh mice (Martin et al. 2007). It has been argued that mitochondrial damage in G93Ahigh-mSOD1 mice is related to supra-normal levels of SOD1 and might not be related causally to the disease process because tg mice expressing high levels of human wild-type SOD1 show some mitochondrial pathology (Jaarsma et al. 2001), but mitochondrial abnormalities in motor neurons have been found histologically also in G93Alow-mSOD1 mice (Sasaki et al. 2004) and in mice with only skeletal muscle expression of human SOD1 (Wong and Martin 2010). Thus, mitochondria could be primary sites of human SOD1 toxicity in tg mice irrespective of transgene copy number, tissue expression, and expression level of human SOD1, but direct, unequivocal causal relationships have been lacking.
Mutated and wild-type forms of human SOD1 can cause ALS in tg mice (Wong and Martin 2010). Human mSOD1 proteins appear to acquire a toxic property or function, rather than having diminished O2- scavenging activity (Deng et al. 1993; Borchelt et al. 1994; Yim et al. 1996). Wild-type SOD1 can gain toxic properties through loss of Zn (Estévez et al. 1999) and oxidative modification (Kabashi et al. 2007; Ezzi et al. 2007). A gain in aberrant oxidative chemistry appears to contribute to the mechanisms of mitochondriopathy in G93Ahigh mice (Beckman et al. 1993; Liochev and Fridovich 2003). G93A-mSOD1 has enhanced free radical-generating capacity compared to wild-type enzyme (Yim et al. 1996) and can catalyze protein oxidation by hydroxyl-like intermediates and carbonate radical (Pacher et al. 2007). G93Ahigh mice have increased protein carbonyl formation in total spinal cord tissue extracts at pre-symptomatic disease (Andrus et al. 1998). Protein carbonyl formation in mitochondrial membrane-enriched fractions of spinal cord is a robust signature of incipient disease (Andrus et al. 1998). A mass spectroscopy study of G93Ahigh mice identified proteins in total spinal cord tissue extracts with greater than baseline carbonyl modification, including SOD1, translationally controlled tumor protein, and UCHL1 (Poon et al. 2005). Nitrated and aggregated cytochrome c oxidase subunit-I accumulate in G93Ahigh mouse spinal cord (Martin et al. 2007). Nitrated MnSOD accumulates also in G93Ahigh mouse spinal cord (Martin et al. 2007). Toxic properties of mSOD1 might also be mediated through protein binding or aggregation. Endogenous mouse SOD1 (Okado-Matsumoto and Fridovich 2001) and human wild-type SOD1 and mSOD1 (Higgins et al. 2002) associate with mitochondria. Human SOD1 mutants associate with spinal cord mitochondria in mSOD1 mice and can bind Bcl-2 (Pasinelli et al. 2004), thus potentially being decoys or dominant negative regulators of cell survival molecules, but it is not known if this process is occurring specifically in motor neurons. This observation is intriguing considering the finding that mitochondrial Bcl-2 is depleted in human ALS (Martin 1999). Binding of mSOD1 (and perhaps its low-mobility species) to mitochondria has been reported to be spinal cord selective and age-dependent (Pasinelli et al. 2004), but this work also lacks cellular resolution. A recent biochemical in vitro study has shown that endogenous SOD1 in the mitochondrial intermembrane space controls cytochrome c-catalyzed peroxidation and that G93A-mSOD1 mediates greater ROS production in the intermembrane space compared to wild-type SOD1 (Goldsteins et al. 2008). Human SOD1 mutants can also shift mitochondrial redox potential when expressed in cultured cells (Ferri et al. 2006). Nevertheless, the direct links between the physicochemical changes in wild-type and mutant SOD1 and the mitochondrial functional and structural changes associated with ALS and motor neuron degeneration remain uncertain.
EM studies of motor neurons in G93Ahigh mice have shown that the outer mitochondrial membrane (OMM) remains relatively intact to permit formation of mega-mitochondria (Kong and Xu 1998; Martin et al. 2007; Martin et al. 2009). Moreover, early in the disease of these mice, mitochondria in dendrites in spinal cord ventral horn undergo extensive cristae and matrix remodeling, while few mitochondria in motor neuron cell bodies show major structural changes (Martin et al. 2009). Thus, disease might start distally in mitochondria of motor neuron processes (Martin et al. 2007; Martin et al. 2009). Another interpretation of ultrastructural findings is that the mSOD1 causes mitochondrial degeneration by inducing OMM extension and leakage and intermembrane space expansion (Higgins et al. 2003). Mechanisms for this damage could be related to mSOD1 gaining access to the mitochondrial intermembrane space (Okado-Matsumoto and Fridovich 2001; Higgins et al. 2002; Higgins et al. 2003). This mitochondrial conformation seen by EM might favor the formation of the mitochondrial permeability transition pore (Crompton 2004; Bernardi et al. 2006; Zorov et al. 2007); indeed, we found evidence for increased contact sites between the OMM and IMM in dendritic mitochondria in G93Ahigh mice (Martin et al. 2009). Another feature of motor neurons in young G93Ahigh mice, before symptoms emerge, is apparent fission of ultrastructurally normal mitochondria in cell bodies and fragmentation of abnormal mitochondria (Martin et al. 2009). It is not clear if the cristae and matrix remodeling and the apparent fragmentation and fission mitochondria are related or independent events and if these abnormalities interfere with mitochondrial trafficking; nevertheless, morphological observations enforce the idea that mitochondria could be important to the pathobiology of mSOD1 toxicity to motor neurons in G93Ahigh mice.
We have hypothesized that mitochondrial trafficking perturbations occur in motor neurons of mSOD1 (Martin et al. 2007). Some data support the novel idea that mitochondria might act as messengers from distal regions (axon branches and dendrites) of motor neurons in mSOD1 mice. G93Ahigh-mSOD1 mouse motor neurons accumulate mitochondria from the axon terminals and generate higher levels of superoxide, nitric oxide (NO), and peroxynitrite (ONOO-) than motor neurons in tg mice expressing human wild-type SOD1 (Martin et al. 2007). This mitochondrial accumulation occurs at a time when motor neuron cell body volume is increasing, suggestive of ongoing abnormalities with ATP production or plasma membrane Na+,K+ ATPase (Martin et al. 2007). G93A-mSOD1 perturbs anterograde axonal transport of mitochondria in cultured primary embryonic motor neurons (De Vos et al. 2007) making it possible that retrogradely transported mitochondria with toxic properties from the neuromuscular junction fail to be returned to distal processes (Martin et al. 2007). Mitochondria with enhanced toxic potential from distal axons and terminals could therefore have a “Trojan horse” role in triggering degeneration of motor neurons in ALS via retrograde transport from diseased skeletal muscle.
Motor neurons in G93Ahigh-mSOD1 mice also accumulate higher levels of intracellular Ca2+ than motor neurons in age-match control mice (Martin et al. 2007). This finding could be relevant to activation of the mitochondrial permeability transition pore (Zoratti and Szabo 1995; Crompton 1999; Leung and halestrap 2008). The intracellular Ca2+ signal in motor neurons is very compartmental and mitochondrial-like in its appearance (Martin et al. 2005; Martin et al. 2007). Abnormal elevations of intracellular Ca2+ in G93Ahigh-mSOD1 mouse motor neurons have been seen also by different Ca2+ detection methods (Siklos et al. 1998; Jaiswal and Keller 2009). Recent work on a mouse neuromuscular junction preparation has shown that mitochondrial Ca2+ accumulation is accompanied by greater mitochondrial depolarization, specifically within motor neuron terminals of human mutant SOD1 tg mice (Nguyen et al. 2009).
NO signaling mechanisms in mitochondria of ALS mice appear to be involved in the pathogenesis. Motor neurons seem to be unique regarding NO production because they express constitutively low levels of inducible NO synthase (iNOS) (Martin et al. 2005; Martin et al. 2007; Chen et al. 2010). G93Ahigh-mSOD1 mouse motor neurons accumulate nicotinamide adenine dinucleotide phosphate diaphorase and iNOS-like immunoreactivity (Martin et al. 2007; Chen et al. 2010). iNOS is also up-regulated aberrantly in human sporadic ALS motor neurons (Sasaki et al. 2000). iNos (Nos2) gene knockout (Martin et al. 2007) and iNOS inhibition with 1,400 W (Chen et al. 2010) extend significantly the lifespan of G93Ahigh-mSOD1 mice. Thus, mitochondrial oxidative stress, Ca2+ dysregulation, iNOS activation, protein nitration, and protein aggregation (not necessarily SOD1 aggregation) are all likely intrinsic, cell-autonomous mechanisms in the process of motor neuron degeneration caused by mSOD1 in mice (Chen et al. 2010). The mechanistic basis for the differences between human ALS and mSOD1 mice, regarding cell death phenotype (Martin 2010a; Martin 2010b) is not yet clear, but could be related to the extreme non-physiological expression of toxic mSOD1 or to fundamental differences in cellular death mechanisms in human and mouse neurons or tissue inflammation that drive motor neurons in mSOD1 tg mice to necrotic-like death according to the cell death matrix concept (Martin 2010a). Another contributing factor for this difference between human and mouse motor neurons is that mitochondria are functionally diverse and have species-specific activities and molecular compositions, including the makeup of the mitochondrial permeability transition pore (Kunz 2003). These possibilities allow for skepticism regarding the suitability of previous tg mSOD1 mouse lines to model human ALS. Therefore, we have recently created a tg mouse with restricted expression of human SOD1 in skeletal muscle that develop ALS with a motor neuron degeneration phenotype similar to that seen in human ALS (Wong and Martin 2010).
The mPTP contributes to disease mechanisms in ALS mice
Despite the implication of toxic effects of mSOD1 on mitochondria in mouse ALS, cause-effect relationships between abnormal functioning of mitochondria and initiation and progression of disease have been uncertain. These relationships need to be known because this knowledge could lead to new mechanism-based treatments for ALS. One specific therapeutic target of investigation for mitochondria in disease causality in ALS is the mPTP (Martin 2010c).
The mPTP was first implicated in mouse ALS pathogenesis using pharmacological approaches. Cyclopsorine A treatment of G93Ahigh mice, delivered into the cerebral ventricle or systemically to mice on a multiple drug resistance type 1a/b background (to inactivate the blood-brain barrier), improved outcome modestly (Keep et al. 2001; Karlsson et al. 2004; Kirkinezos et al. 2004). These studies were confounded by the immunosuppressant actions of cyclopsorine A through calcineurin inhibition. Pharmacological studies using cyclophilin D (CyPD) inhibitors devoid of effects on calcineurin need to be done on ALS mice. Another study showed that treatment with cholest-4-en-3-one oxime (TRO19622), a drug that binds the voltage-dependent anion channel (VDAC) and the 18 kDa translocator protein (TSPO, or peripheral benzodiazepine receptor), improved motor performance, delayed disease onset, and extended survival of G93Ahigh mice (Bordet et al. 2007). However, another study using a different TSPO ligand (Ro-4864) did not show positive effects with G93Ahigh mice (Mills et al. 2008).
We identified CyPD and the adenine nucleotide translocator (ANT) as targets of nitration in ALS mice (Martin et al. 2009). CyPD nitration is elevated in early- to mid-symptomatic stages, but declines to baseline at end-stage disease (Martin et al. 2009). ANT nitration is pertinent because it occurs in pre-symptomatic and symptomatic stages but not at end-stage disease or in tg mice expressing human wild-type SOD1 (Martin et al. 2009). The ANT is important in the context of age-related neurodegenerative disease because it undergoes carbonyl modification during aging as seen in housefly flight muscle (Yan and Sohal 1998) and rat brain (Prokai et al. 2007). In vitro cell-free and cell experiments have shown that NO and ONOO- can act directly on the ANT to induce mitochondrial permeabilization in a cyclosporine A-sensitive manner (Vieira et al. 2001). Oxidative stress enhances the binding of CyPD to ANT (McStay et al. 2002). Some SOD1 mutants are unstable and lose copper (Trumbull and Beckman 2009), and interestingly, copper interactions with ANT and thiol modification of ANT can cause mPTP opening (Costantini et al. 2000; García et al. 2007). Together these data and future work could reveal that oxidative and nitrative damage to proteins, some of which are components of the mPTP (Bernardi et al. 2006: Leung and Halestrap 2008), in G93Ahigh mice is targeted rather than stochastic and could impinge on the functioning of the mPTP.
We examined directly the role of CyPD in the process of motor neuron disease in ALS mice through gene-ablation (Martin et al. 2009). G93Ahigh-mSOD1 mice without CyPD show markedly delayed disease onset and lived significantly longer than tg mice with CyPD. The effect of CyPD deletion was much more prominent in female mice than in male mice (Martin et al. 2009). Female mice showed positive effects with only haplo-deletion of CyPD. Ppif gene ablation in tg mice with much lower levels of human mSOD1 expression and a slower disease progression (G93Alow-mSOD1 mice) also show significantly delayed disease onset and lived significantly longer than tg mice with CyPD (Martin et al. 2009). Thus, some form of mPTP pathobiology is occurring regardless of whether transgene expression of G93A is high or low.
Nevertheless, most G93A-mSOD1 mice without CyPD develop eventually motor neuron disease and die. Other work on CypD null mice has shown that high concentrations of Ca2+ (2 mM) can still lead to mPTP activation without CyPD and that cell deaths caused by Bid, Bax, DNA damage and TNF-α still occur without CyPD (Grimm and Brdiczka 2007). The effects of CyPD deficiency on motor neuron cell death mechanisms thus need detailed examination, but the cell death phenotype might switch or convert to another form with the attenuation of mitochondrial swelling. A switch in the cell death morphology and molecular mechanisms in motor neurons of mSOD1 mice without CyPD is an outcome consistent with the cell death matrix concept (Martin 2010a).
Acknowledgments
The author thanks all of the individuals in his lab for their hard work, particularly Yan Pan and Ann Price for data generated on human ALS autopsy brain and spinal cord and mSOD1 tg mice. This work was supported by grants from the U.S. Public Health Service, NIH-NINDS (NS065895, NS052098) and NIH-NIA (AG016282).
References
- Abe K, Pan L-H, Watanabe M, Kato T, Itoyama Y. Induction of nitrotyrosine-like immunoreactivity in the lower motor neuron of amyotrophic lateral sclerosis. Neurosci Lett. 1995;199:152–154. [Abstract] [Google Scholar]
- Andrus PK, Fleck TJ, Gurney ME, Hall ED. Protein oxidative damage in a transgenic mouse model of familial amyotrophic lateral sclerosis. J Neurochem. 1998;71:2041–2048. [Abstract] [Google Scholar]
- Babcock D, Hille B. Mitochondrial oversight of cellular Ca2+ signaling. Curr Opin Neurobiol. 1998;8:398–404. [Abstract] [Google Scholar]
- Beal MF. Oxidatively modified protein in aging and disease. Free Radic Biol Med. 2002;32:797–803. [Abstract] [Google Scholar]
- Beal MF, Ferrante RJ, Browne SE, Matthews RT, Kowall NW, Brown RH., Jr Increased 3-nitrotyrosine in both sporadic and familial amyotrophic lateral sclerosis. Ann Neurol. 1997;42:644–654. [Abstract] [Google Scholar]
- Beckman JS, Carson M, Smith CD, Koppenol WH. ALS, SOD and peroxynitrite. Nature. 1993;364:548. [Abstract] [Google Scholar]
- Bendotti C, Calvaresi N, Chiveri L, Prelle A, Moggio M, Braga M, Silani V, De Biasi S. Early vacuolization and mitochondrial damage in motor neurons of FALS mice are not associated with apoptosis or with changes in cytochrome oxidase histochemical reactivity. J Neurol Sci. 2001;1912:5–33. [Abstract] [Google Scholar]
- Bergmann F, Keller BU. Impact of mitochondrial inhibition on excitability and cytosolic Ca2+ levels in brainstem motoneurones. J Physiol. 2004;5554:5–59. [Abstract] [Google Scholar]
- Bernardi P, Krauskopf A, Basso E, Petronilli V, Blalchy-Dyson E, Di Lisa F, Forte MA. The mitochondrial permeability transition from in vitro artifact to disease target. FEBS J. 2006;273:2077–2099. [Abstract] [Google Scholar]
- Bilsland LG, Nirmalananthan N, Yip J, Greensmith L, Duhcen MR. Expression of mutant SOD1G93A in astrocytes induces functional deficits in motoneuron mitochondria. J Neurochem. 2008;107:1271–1283. [Abstract] [Google Scholar]
- Borchelt DR, Lee MK, Slunt HH, Guarnieri M, Xu Z-S, Wong PC, Brown RH, Jr, Price DL, Sisodia SS, Cleveland DW. Superoxide dismutase 1 with mutations linked to familial amyotrophic lateral sclerosis possesses significant activity. Proc Natl Acad Sc USA. 1994;918:292–8296. [Europe PMC free article] [Abstract] [Google Scholar]
- Bordet T, Buisson B, Michaud M, Drouot C, Galea P, Delaage P, Akentieva NP, Evers AS, Covey DF, Ostuni MA, Lacapere JJ-J, Massaad C, Schmacher M, Steidl E-M, Maux D, Delaage M, Henderson CE, Pruss RM. Identification and characterization of cholest-4-en-3-one, oxime (TRO19622), a novel drug candidate for amyotrophic lateral sclerosis. J Pharmacol Exp Ther. 2007;3227:09–720. [Abstract] [Google Scholar]
- Borthwick GM, Johnson MA, Ince PG, Shaw PJ, Turnbull DM. Mitochondrial enzyme activity in amyotrophic lateral sclerosis: implications for the role of mitochondria in neuronal cell death. Ann Neurol. 1999;46:787–790. [Abstract] [Google Scholar]
- Borthwick GM, Taylo RW, Walls TJ, Tonska K, Taylor GA, Shaw PJ, Ince PG, Turnbull DM. Motor neuron disease in a patient with a mitochondrial tRNAIle mutation. Ann Neurol. 2006;59:570–574. [Abstract] [Google Scholar]
- Browne SE, Bowling AC, Baik MJ, Gurney M, Brown RH, Jr, Beal MF. Metabolic dysfunction in familial, but not sporadic, amyotrophic lateral sclerosis. J Neurochem. 1998;71:281–287. [Abstract] [Google Scholar]
- Chang Q, Martin LJ. Glycinergic innervation of motoneurons is deficient in amyotrophic lateral sclerosis mice: a confocal quantitative analysis. Am J Path. 2009;174:574–585. [Europe PMC free article] [Abstract] [Google Scholar]
- Chang Q, Martin LJ. Glycine receptor channels in spinal motoneurons are abnormal in a transgenic mouse model of amyotrophic lateral sclerosis. J Neurosci. 2011;3:2815–2827. [Europe PMC free article] [Abstract] [Google Scholar]
- Chang DTW, Reynolds IJ. Mitochondrial trafficking and morphology in healthy and injured neurons. Prog Brain Res. 2006;80:241–268. [Abstract] [Google Scholar]
- Chen K, Northington FJ, Martin LJ. Inducible nitric oxide synthase is present in motor neuron mitochondria and Schwann cells and contributes to disease mechanisms in ALS mice. Brain Struct Func. 2010;214:219–234. [Europe PMC free article] [Abstract] [Google Scholar]
- Chiu AY, Zhai P, Dal Canto MC, Peters TM, Kwon YW, Prattis SM, Gurney ME. Age-dependent penetrance of disease in a transgenic mouse model of familial amyotrophic lateral sclerosis. Mol Cell Neurosci. 1995;6:349–362. [Abstract] [Google Scholar]
- Chow CY, Lander JE, Bergren SK, Sapp PC, Grant AE, Jones JM, Everett L, Lenk GM, McKenna-Yasek DM, Weisman LS, Figlewicz D, Brown RH, Meisler MH. Deleterious variants of FIG4, a phosphoinositade phosphatase, in patients with ALS. Am J Human Gen. 2009;84:85–88. [Europe PMC free article] [Abstract] [Google Scholar]
- Comi GP, Bordoni A, Salani S, Franeschina L, Sciacco M, Prelle A, Fortunato F, Zeviani M, Napoli L, Bresolin N, Moggio M, Ausenda CD, Taanman JW, Scarlato G. Cytochrome c oxidase subunit I microdeletion in a patient with motor neuron disease. Ann Neurol. 1998;43:110–116. [Abstract] [Google Scholar]
- Corral-Debrinski M, Horton T, Lott MT, Shoffner JM, Beal MF, Wallace DC. Mitochondrial DNA deletions in human brain: regional variability and increase with advanced age. Nat Genet. 1992;2:324–329. [Abstract] [Google Scholar]
- Costantini P, Belzacq A-S, Vieira HLA, Larochette N, de Pablo MA, Zamzami N, Susin SA, Brenner C, Kroemer G. Oxidation of a critical thiol residue of the adenine nucleotide translocator enforces Bcl-2-independent permeability transition pore opening and apoptosis. Oncogene. 2000;19:307–314. [Abstract] [Google Scholar]
- Crompton M. The mitochondrial permeability transition pore and its role in cell death. Biochem J. 1999;341:233–249. [Europe PMC free article] [Abstract] [Google Scholar]
- Crompton M. Mitochondria and aging: a role for the permeability transition? Aging Cell. 2004;3:3–6. [Abstract] [Google Scholar]
- Dal Canto MC, Gurney ME. Development of central nervous system pathology in a murine transgenic model of human amyotrophic lateral sclerosis. Am J Pathol. 1994;1451:271–1279. [Europe PMC free article] [Abstract] [Google Scholar]
- De Vos KJ, Chapman AL, Tennant ME, Manser C, Tudor EL, Lau K-F, Browlees J, Ackerley S, Shaw PJ, McLoughlin DM, Shaw CE, Leigh PN, Miller CCJ, Grierson AJ. Familial amyotrophic lateral sclerosis-linked SOD1 mutants perturb fast axonal transport to reduce axonal mitochondrial content. Hum Mol Genet. 2007;16:2720–2728. [Europe PMC free article] [Abstract] [Google Scholar]
- Deng H-X, Hentati A, Tainer JA, Iqbal Z, Cayabyab A, Hung W-Y, Getzoff ED, Hu P, Herzfeldt B, Roos RP, Warner C, Deng G, Soriano E, Smyth C, Parge HE, Ahmed A, Roses AD, Hallewell RA, Pericak-Vance MA, Siddique T. Amyotrophic lateral sclerosis and structural defects in Cu, Zn superoxide dismutase. Science. 1993;261:1047–1051. [Abstract] [Google Scholar]
- Echaniz-Laguna A, Zoll J, Ponsot E, N’Guessan B, Tranchant C, Loeffler J-P, Lampert E. Muscular mitochondrial function in amyotrophic lateral sclerosis is progressively altered as the disease develops: a temporal study in man. Exp Neurol. 2006;198:25–30. [Abstract] [Google Scholar]
- Elden AC, Kim HJ, Hart MP, Chen-Plotkin AS, Johnson BS, Fang X, Armakola M, Geser F, Greene R, Lu MM, Padmanabhan A, Clay-Falcone D, McCluskey L, Elman L, Juhr D, Gruber PJ, Rüb U, Auburger G, Trojanowski JQ, Lee VM, Van Deerlin VM, Bonini NM, Gitler AD. Ataxin-2 intermediate-length polyglutamine expansions are associated with increased risk for ALS. Nature. 2010;466:1069–1075. [Europe PMC free article] [Abstract] [Google Scholar]
- Estévez AG, Crow JP, Sampson JB, Reiter C, Zhuang Y, Richardson GJ, Tarpey L, Barbeito MM, Beckman JS. Induction of nitric oxide-dependent apoptosis in motor neurons by zinc-deficient superoxide dismutase. Science. 1999;286:2498–2500. [Abstract] [Google Scholar]
- Ezzi SA, Urushitani M, Julien J-P. Wild-type superoxide dismutase acquires binding and toxic properties of ALS-linked mutant forms through oxidation. J Neurochem. 2007;102:170–178. [Abstract] [Google Scholar]
- Ferrante RJ, Browne SE, Shinobu LA, Bowling AC, Baik MJ, MacGarvey U, Kowall NW, Brown RH, Jr, Beal MF. Evidence of increased oxidative damage in both sporadic and familial amyotrophic lateral sclerosis. J Neurochem. 1997;69:2064–2074. [Abstract] [Google Scholar]
- Ferri A, Cozzolino M, Crosio C, Nencini M, Casciati A, Gralla EB, Rotilio G, Valentine JS, Carri MT. Familial ALS-superoxide dismutases associate with mitochondria and shift their redox potentials. Proc Natl Acad Sci USA. 2006;103:13860–13865. [Europe PMC free article] [Abstract] [Google Scholar]
- Flanagan SW, Anderson RD, Ross MA, Oberley LW. Over-expression of manganese superoxide dismutase attenuates neuronal death in human cells expressing mutant (G37R) Cu/Zn-superoxide dismutase. J Neurochem. 2002;81:170–177. [Abstract] [Google Scholar]
- Fridovich I. Superoxide radical and superoxide dismutases. Annu Rev Biochem. 1995;64:97–112. [Abstract] [Google Scholar]
- García N, Martínez-Abundis E, Pavón N, Correa F, Chávez E. Copper induces permeability transition through its interaction with the adenine nucleotide translocase. Cell Biol Int. 2007;31:893–899. [Abstract] [Google Scholar]
- Ginsberg SD, Hemby SE, Mufson EJ, Martin LJ. Cell and tissue microdissection in combination with genomic and proteomic profiling. In: Wouterlood FG, Lanciego JL, Zaborszky L, editors. Neuroanatomical Tract-Tracing 3, Molecules, Neurons, and Systems. Springer; New York: 2006. pp. 109–141. [Google Scholar]
- Goldsteins G, Keksa-Goldsteine V, Ahtiniemi T, Jaronen M, Arens E, Akerman K, Chan RH, Koistinaho J. Deleterious role of superoxide dismutase in the mitochondrial intermembrane space. J Biol Chem. 2008;283:8446–8452. [Abstract] [Google Scholar]
- Grimm S, Brdiczka D. The permeability transition pore in cell death. Apoptosis. 2007;12:841–855. [Abstract] [Google Scholar]
- Gurney ME, Pu H, Chiu AY, Dal Canto MC, Polchow CY, Alexander DD, Caliendo J, Hentati A, Kwon YW, Deng HX, Chen W, Zhai P, Sufit RL, Siddique T. Motor neuron degeneration in mice that express a human Cu, Zn superoxide dismutase mutation. Science. 1994;264:1772–1775. [Abstract] [Google Scholar]
- Hansson MJ, Mansson R, Morota S, Uchino H, Kallur T, Sumi T, Ishii N, Shimazu M, Keep MF, Jegorov A, Elmer E. Calcium-induced generation of reactive oxygen species in brain mitochondria is mediated by permeability transition. Free Radic Biol Med. 2008;45:284–294. [Abstract] [Google Scholar]
- Heath PR, Tomkins J, Ince PG, Shaw PJ. Quantitative assessment of AMPA receptor mRNA in human spinal motor neurons isolated by laser capture microdissection. Neuroreport. 2002;13:1753–1757. [Abstract] [Google Scholar]
- Higgins CMJ, Jung C, Ding H, Xu Z. Mutant Cu, Zn Superoxide dismutase that causes motoneuron degeneration is present in mitochondria in the CNS. J Neurosci. 2002;22:RC215. [Europe PMC free article] [Abstract] [Google Scholar]
- Higgins CM, Jung C, Xu Z. ALS-associated mutant SOD1G93A causes mitochondrial vacuolation by expansion of the intermembrane space and by involvement of SOD1 aggregation and peroxisomes. BMC Neurosci. 2003;4:16. [Europe PMC free article] [Abstract] [Google Scholar]
- Jaarsma D, Rognoni F, van Duijn W, Verspaget HW, Haasdijk ED, Holstege JC. CuZn superoxide dismutase (SOD1) accumulates in vacuolated mitochondria in transgenic mice expressing amyotrophic lateral sclerosis-linked SOD1 mutations. Acta Neuropathol. 2001;102:293–305. [Abstract] [Google Scholar]
- Jaiswal MK, Keller BU. Cu/Zn superoxide dismutase typical for familial amyotrophic lateral sclerosis increases the vulnerability of mitochondria and perturbs Ca2+ homeostasis in SOD1G93A mice. Mol Pharmacol. 2009;75:478–489. [Abstract] [Google Scholar]
- Kabashi E, Valdmanis PN, Dion P, Rouleau GA. Oxidized/misfolded superoxide dismutase-1: the cause of all amyotrophic lateral sclerosis? Ann Neurol. 2007;62:553–559. [Abstract] [Google Scholar]
- Kabashi E, Valdmains PN, Dion P, Spiegelman D, McConkey BJ, Vande Velde C, Bouchard J-P, Lacomblez L, Pochigaeva K, Salachas F, Pradat P-F, Camu W, Meininger V, Dupre N, Rouleau GA. TARDBP mutations in individuals with sporadic and familial amyotrophic lateral sclerosis. Nat Genet. 2008;40:572–574. [Abstract] [Google Scholar]
- Karlsson J, Fong KS, Hansson MJ, Elmer E, Csiszar K, Keep MF. Life span extension and reduced neuronal death after weekly intraventricular cyclosporine injections in the G93A transgenic mouse model of amyotrophic lateral sclerosis. J Neurosurg. 2004;101:128–137. [Abstract] [Google Scholar]
- Keep M, Elmér E, Fong KSK, Csiszar K. Intrathecal cyclosporin prolongs survival of late-stage ALS mice. Brain Res. 2001;894:27–31. [Abstract] [Google Scholar]
- Kirkinezos IG, Hernandez BWG, Moraes CT. An ALS mouse model with a permeable blood-brain barrier benefits from systemic cyclosporine A treatment. J Neurochem. 2004;88:821–826. [Abstract] [Google Scholar]
- Kong J, Xu Z. Massive mitochondrial degeneration in motor neurons triggers the onset of amyotrophic lateral sclerosis in mice expressing a mutant SOD1. J Neurosci. 1998;18:3241–3250. [Europe PMC free article] [Abstract] [Google Scholar]
- Kunz WS. Different metabolic properties of mitochondrial oxidative phosphorylation in different cell types- important implications for mitochondrial cytopathies. Exp Physiol. 2003;88:149–154. [Abstract] [Google Scholar]
- Kwak S, Kawahara Y. Deficient RNA editing of GluR2 and neuronal death in amyotrophic lateral sclerosis. J Mol Med. 2005;83:110–120. [Abstract] [Google Scholar]
- Leung AWC, Halestrap AP. Recent progress in elucidating the molecular mechanism of the mitochondrial permeability transition pore. Biochim Biophys Acta. 2008;1777:946–952. [Abstract] [Google Scholar]
- Lieberman AR. The axon reaction: a review of the principal features of perikaryal responses to axon injury. Int Rev Neurobiol. 1971;14:49–124. [Abstract] [Google Scholar]
- Liochev SI, Fridovich I. Mutant Cu, Zn superoxide dismutases and familial amyotrophic lateral sclerosis: evaluation of oxidative hypotheses. Free Radic Biol Med. 2003;34:1383–1389. [Abstract] [Google Scholar]
- Maekawa S, Al-Sarraj S, Kibble M, Landau S, Parnavelas J, Cotter D, Everall I, Leigh PN. Cortical selective vulnerability in motor neurons disease: a morphometric study. Brain. 2004;127:1237–1251. [Abstract] [Google Scholar]
- Martin LJ, Al-Abdulla NA, Brambrink AM, Kirsch JR, Sieber FE, Portera-Cailliau C. Neurodegeneration in excitotoxicity, global cerebral ischemia, and target deprivation: a perspective on the contributions of apoptosis and necrosis. Brain Res Bull. 1998;46:281–309. [Abstract] [Google Scholar]
- Martin LJ. p53 is abnormally elevated and active in the CNS of patients with amyotrophic lateral sclerosis. Neurobiol Dis. 2000;7:613–622. [Abstract] [Google Scholar]
- Martin LJ, Gertz B, Pan Y, Price AC, Molkentin JD, Chang Q. The mitochondrial permeability transition pore in motor neurons: involvement in the pathobiology of ALS mice. Exp Neurol. 2009;218:33–346. [Europe PMC free article] [Abstract] [Google Scholar]
- Martin LJ. Mitochondrial and cell death mechanisms in neurodegenerative disease. Pharmaceuticals. 2010a;3:839–915. [Europe PMC free article] [Abstract] [Google Scholar]
- Martin LJ. The mitochondrial permeability transition pore: a molecular target for amyotrophic lateral sclerosis. Biochim Biophys Acta. 2010b;1802:186–197. [Europe PMC free article] [Abstract] [Google Scholar]
- Martin LJ. Olesoxime, a cholesterol-like neuroprotectant for the potential treatment of amyotrophic lateral sclerosis. IDrugs. 2010c;13:568–580. [Europe PMC free article] [Abstract] [Google Scholar]
- Martin LJ, Liu Z. Opportunities for neuroprotection in ALS using cell death mechanism rationales. Drug Discov Today. 2004;1:135–143. [Google Scholar]
- Martin LJ, Price AC, Kaiser A, Shaikh AY, Liu Z. Mechanisms for neuronal degeneration in amyotrophic lateral sclerosis and in models of motor neuron death. Int J Mol Med. 2000;5:3–13. [Abstract] [Google Scholar]
- Martin LJ, Chen K, Liu Z. Adult motor neuron apoptosis is mediated by nitric oxide and Fas death receptor linked by DNA damage and p53 activation. J Neurosci. 2005;25:6449–6459. [Europe PMC free article] [Abstract] [Google Scholar]
- Martin LJ, Liu Z, Chen K, Price AC, Pan Y, Swaby JA, Golden WC. Motor neuron degeneration in amyotrophic lateral sclerosis mutant superoxide dismutase-1 transgenic mice: mechanisms of mitochondriopathy and cell death. J Comp Neurol. 2007;500:20–46. [Abstract] [Google Scholar]
- Maruyama H, Morino H, Ito H, Izumi Y, Kato H, Watanabe Y, Kinoshita Y, Kamada M, Nodera H, Suzuki H, Komure O, Matsuura S, Kobatake K, Morimoto N, Abe K, Suzuki N, Aoki M, Kawata A, Hirai T, Kato T, Ogasawara K, Hirano A, Takumi T, Kusaka H, Hagiwara K, Kaji R, Kawakami H. Mutations of optineurin in amyotrophic lateral sclerosis. Nature. 2010;465:223–226. [Abstract] [Google Scholar]
- Mawrin C, Kirches E, Krause G, Wiedemann FR, Vorwerk CK, Bogerts B, Schildhaus HU, Dietzmann K, Schneider-Stock R. Single-cell analysis of mtDNA levels in sporadic amyotrophic lateral sclerosis. Neuroreport. 2004;15:939–943. [Abstract] [Google Scholar]
- McCord JM, Fridovich I. Superoxide dismutase, an enzymic function for erythrocuprein (hemocuprein) J Biol Chem. 1969;244:6049–6055. [Abstract] [Google Scholar]
- McStay GP, Clarke SJ, Halestrap AP. Role of critical thiol groups on the matrix surface of the adenine nucleotide translocase in the mechanism of the mitochondrial permeability transition pore. Biochem J. 2002;367:541–548. [Europe PMC free article] [Abstract] [Google Scholar]
- Menzies FM, Ince PG, Shaw PJ. Mitochondrial involvement in amyotrophic lateral sclerosis. Neurochem Intl. 2002;40:543–551. [Abstract] [Google Scholar]
- Mills C, Makwana M, Wallace A, Benn S, Schmidt H, Tegeder I, Costigan M, Brown RH, Jr, Raivich G, Woolf C. Ro5-4864 promotes neonatal motor neuron survival and nerve regeneration in adult rats. Eur J Neurosci. 2008;27:937–946. [Abstract] [Google Scholar]
- Mohajeri MH, Figlewicz DA, Bohn MC. Selective loss of alpha motoneurons innervating the medial gastrocnemius muscle in a mouse model of amyotrophic lateral sclerosis. Exp Neurol. 1998;150:329–336. [Abstract] [Google Scholar]
- Nguyen KT, Garcia-Chacon LE, Barrett JN, Barrett EF, David G. The ψm depolarization that accompanies mitochondrial Ca2+ uptake is greater in mutant SOD1 than in wild-type mouse motor terminals. Proc Natl Acad Sci USA. 2009;106:2007–2011. [Europe PMC free article] [Abstract] [Google Scholar]
- Nicholls DG. Mitochondrial function and dysfunction in the cell: its relevance to aging and aging-related disease. Intl J Biochem Cell Biol. 2002;34:1372–1381. [Abstract] [Google Scholar]
- Okado-Matsumoto A, Fridovich I. Subcellular distribution of superoxide (SOD) in rat liver. J Biol Chem. 2001;276:38388–38393. [Abstract] [Google Scholar]
- Pacher P, Beckman JS, Liaudet L. Nitric oxide and peroxynitrite in health and disease. Physiol Rev. 2007;87:315–424. [Europe PMC free article] [Abstract] [Google Scholar]
- Pasinelli P, Belford ME, Lennon N, Bacskai BJ, Hyman BT, Trotti D, Brown RH., Jr Amyotrophic lateral sclerosis-associated SOD1 mutant protein bind and aggregate with Bcl-2 in spinal cord mitochondria. Neuron. 2004;43:19–30. [Abstract] [Google Scholar]
- Poon HF, Hensley K, Thongboonkerd V, Merchant ML, Lynn BC, Pierce WM, Klein JB, Calabrese V, Butterfield DA. Redox proteomics analysis of oxidatively modified proteins in G93A-SOD1 transgenic mice- a model of familial amyotrophic lateral sclerosis. Free Radic Biol Med. 2005;39:435–462. [Abstract] [Google Scholar]
- Prokai L, Yan L-J, Vera-Serrano JL, Stevens SM, Jr, Forster MJ. Mass spectrometry-based survey of age-associated protein carbonylation in rat brain mitochondria. J Mass Spectrom. 2007;42:1583–1589. [Abstract] [Google Scholar]
- Rakhit R, Crow JP, Lepock JR, Kondejewski LH, Cashman NR, Chakrabartty A. Monomeric Cu, Zn-superoxide dismutase is a common misfolding intermediate in the oxidation models of sporadic and familial amyotrophic sclerosis. J Biol Chem. 2004;279:15499–15504. [Abstract] [Google Scholar]
- Rothstein JD, Martin LJ, Kuncl RW. Decreased glutamate transport by brain and spinal cord in amyotrophic lateral sclerosis. N Engl J Med. 1992;326:1464–1468. [Abstract] [Google Scholar]
- Rothstein JD, Van Kammen M, Levey AI, Martin LJ, Kuncl RW. Selective loss of glial glutamate transporter GLT-1 in amyotrophic lateral sclerosis. Ann Neurol. 1995;38:73–84. [Abstract] [Google Scholar]
- Rowland LP, Shneider NA. Amyotrophic lateral sclerosis. N Engl J Med. 2001;344:1688–1700. [Abstract] [Google Scholar]
- Saeed M, Siddique N, Hung WY, Usacheva E, Liu E, Sufit RL, Heller SL, Haines JL, Pericak-Vance M, Siddique T. Paraoxonase cluster polymorphisms are associated with sporadic ALS. Neurology. 2006;67:771–776. [Abstract] [Google Scholar]
- Sasaki S, Iwata M. Ultrastructural changes of synapses of Betz cell in patients with amyotrophic lateral sclerosis. Neurosci Lett. 1999;268:29–32. [Abstract] [Google Scholar]
- Sasaki S, Shibata N, Komori T, Iwata M. iNOS and nitrotyrosine immunoreactivity in amyotrophic lateral sclerosis. Neurosci Lett. 2000;291:44–48. [Abstract] [Google Scholar]
- Sasaki S, Warita H, Murakami T, Abe K, Iwata M. Ultrastructural study of mitochondria in the spinal cord of transgenic mice with a G93A mutant SOD1 gene. Acta Neuropathol. 2004;107:461–474. [Abstract] [Google Scholar]
- Sathasivam S, Ince PG, Shaw PJ. Apoptosis in amyotrophic lateral sclerosis: a review of the evidence. Neuropathol Appl Neurobiol. 2001;27:257–274. [Abstract] [Google Scholar]
- Schymick JC, Talbot K, Traynor GJ. Genetics of amyotrophic lateral sclerosis. Hum Mol Genet. 2007;16:R233–R242. [Abstract] [Google Scholar]
- Siklos L, Engelhardt J, Harat Y, Smith RG, Joo F, Appel SH. Ultrastructural evidence for altered calcium in motor nerve terminals in amyotrophic lateral sclerosis. Ann Neurol. 1996;39:203–216. [Abstract] [Google Scholar]
- Siklos L, Engelhardt JI, Alexianu ME, Gurney ME, Siddique T, Appel SH. Intracellular calcium parallels motoneuron degeneration in SOD-1 mutant mice. J Neuropath Exp Neurol. 1998;57:571–587. [Abstract] [Google Scholar]
- Soong NW, Hinton DR, Cortopassi G, Arnheim N. Mosaicism for a specific somatic mitochondrial DNA mutation in adult human brain. Nat Genet. 1992;2:318–323. [Abstract] [Google Scholar]
- Soraru G, Vergani L, Fedrizzi L, D’Ascenzo C, Polo A, Bernazzi B, Angelini C. Activities of mitochondrial complexes correlate with nNOS amount in muscle from ALS patients. Neuropath Appl Neurobiol. 2007;33:204–211. [Abstract] [Google Scholar]
- Stephens B, Guiloff RJ, Navarrete R, Newman P, Nikhar N, Lewis P. Widespread loss of neuronal populations in spinal ventral horn in sporadic motor neuron disease. A morphometric study. J Neurol Sci. 2006;244:41–58. [Abstract] [Google Scholar]
- Trumbull KA, Beckman JS. A role for copper in the toxicity of zinc-deficient superoxide dismutase to motor neurons in amyotrophic lateral sclerosis. Antioxid Redox Signal. 2009;11:1627–1639. [Europe PMC free article] [Abstract] [Google Scholar]
- Turner BJ, Talbot K. Transgenics, toxicity and therapeutics in rodent models of mutant SOD1-mediated familial ALS. Prog Neurobiol. 2008;85:94–134. [Abstract] [Google Scholar]
- Vance C, Rogelj B, Hortobagyi T, de Vos KJ, Nishimura AL, Sreedharan J, Hu X, Smith B, Ruddy D, Wright P, Ganesaligam J, Williams KL, Tripathi V, Saraj S, Al-Chalabi A, Leigh N, Blair IP, Nicholson G, de Belleroche J, Gallo J-M, Miller CC, Shaw CE. Mutations in FUS, an RNA processing protein, cause familial amyotrophic lateral sclerosis type 6. Science. 2009;323:1208–1211. [Europe PMC free article] [Abstract] [Google Scholar]
- Vieira HLA, Belzacq A-S, Haouzu D, Bernassola F, Cohen I, Jacotot E, Ferri KF, Hamel CE, Bartle LM, Melino G, Brenner C, Goldmacher V, Kroemer G. The adenine nucleotide translocator: a target of nitric oxide, peroxynitrite, and 4-hydroxynonenal. Oncogene. 2001;20:4305–4316. [Abstract] [Google Scholar]
- Vielhaber S, Kunz D, Winkler K, Wiedemann FR, Kirches E, Feistner H, Heinze HJ, Elger CE, Schubert W, Kunz WS. Mitochondrial DNA abnormalities in skeletal muscle of patients with sporadic amyotrophic lateral sclerosis. Brain. 2000;123:1339–1348. [Abstract] [Google Scholar]
- Wong M, Martin LJ. Skeletal muscle-restricted expression of human SOD1 causes motor neuron degeneration in transgenic mice. Hum Mol Genet. 2010;9:2284–2302. [Europe PMC free article] [Abstract] [Google Scholar]
- Wong PC, Pardo CA, Borchelt DR, Lee MK, Copeland NG, Jenkins NA, Sisodia SS, Cleveland DW, Price DL. An adverse property of a familial ALS-linked SOD1 mutation causes motor neuron disease characterized by vacuolar degeneration of mitochondria. Neuron. 1995;14:1105–1116. [Abstract] [Google Scholar]
- Yan L-J, Sohal RS. Mitochondrial adenine nucleotide translocase is modified oxidatively during aging. Proc Natl Acad Sci USA. 1998;95:12896–12901. [Europe PMC free article] [Abstract] [Google Scholar]
- Yim MB, Kang J-H, Yim H-S, Kwak H-S, Chock PB, Stadtman ER. A gain-of-function of an amyotrophic lateral sclerosis-associated Cu, Zn-superoxide dismutase mutant: an enhancement of free radical formation due to a decrease in Km for hydrogen peroxide. Proc Natl Acad Sci USA. 1996;93:5709–5714. [Europe PMC free article] [Abstract] [Google Scholar]
- Zoccolella S, Santamato A, Lamberti P. Current and emerging treatments for amyotrophic lateral sclerosis. Neuropsychiatr Dis Treat. 2009;5:577–595. [Europe PMC free article] [Abstract] [Google Scholar]
- Zoratti M, Szabo I. The mitochondrial permeability transition. Biochem Biophys Acta. 1995;1241:139–176. [Abstract] [Google Scholar]
- Zorov DB, Isave NK, Plotnikov EY, Zorova LD, Stelmashook EV, Vasileva AK, Arkhagelskaya AA, Khrjapenkova TG. The mitochondrion as Janus Bifrons. Biochemistry (Moscow) 2007;72:1115–1126. [Abstract] [Google Scholar]
Full text links
Read article at publisher's site: https://doi.org/10.1007/s10863-011-9395-y
Read article for free, from open access legal sources, via Unpaywall:
https://europepmc.org/articles/pmc4131252?pdf=render
Citations & impact
Impact metrics
Citations of article over time
Smart citations by scite.ai
Explore citation contexts and check if this article has been
supported or disputed.
https://scite.ai/reports/10.1007/s10863-011-9395-y
Article citations
Effect of the Mediterranean diet supplemented with nicotinamide riboside and pterostilbene and/or coconut oil on anthropometric variables in amyotrophic lateral sclerosis. A pilot study.
Front Nutr, 10:1232184, 22 Sep 2023
Cited by: 5 articles | PMID: 37810917 | PMCID: PMC10556480
Modulation of Reactive Oxygen Species Homeostasis as a Pleiotropic Effect of Commonly Used Drugs.
Front Aging, 3:905261, 14 Jun 2022
Cited by: 2 articles | PMID: 35821802 | PMCID: PMC9261327
Review Free full text in Europe PMC
Targeting Tau Mitigates Mitochondrial Fragmentation and Oxidative Stress in Amyotrophic Lateral Sclerosis.
Mol Neurobiol, 59(1):683-702, 10 Nov 2021
Cited by: 12 articles | PMID: 34757590
Mitochondrial calcium exchange in physiology and disease.
Physiol Rev, 102(2):893-992, 26 Oct 2021
Cited by: 106 articles | PMID: 34698550 | PMCID: PMC8816638
Review Free full text in Europe PMC
Role of EphA4 in Mediating Motor Neuron Death in MND.
Int J Mol Sci, 22(17):9430, 30 Aug 2021
Cited by: 2 articles | PMID: 34502339 | PMCID: PMC8430883
Review Free full text in Europe PMC
Go to all (33) article citations
Data
Data behind the article
This data has been text mined from the article, or deposited into data resources.
BioStudies: supplemental material and supporting data
Protein structures in PDBe (3)
-
(1 citation)
PDBe - 7q21View structure
-
(1 citation)
PDBe - 5q13View structure
-
(1 citation)
PDBe - 7q36View structure
Similar Articles
To arrive at the top five similar articles we use a word-weighted algorithm to compare words from the Title and Abstract of each citation.
Mitochondrial pathobiology in Parkinson's disease and amyotrophic lateral sclerosis.
J Alzheimers Dis, 20 Suppl 2:S335-56, 01 Jan 2010
Cited by: 14 articles | PMID: 20413846
Review
Biology of mitochondria in neurodegenerative diseases.
Prog Mol Biol Transl Sci, 107:355-415, 01 Jan 2012
Cited by: 93 articles | PMID: 22482456 | PMCID: PMC3530202
Review Free full text in Europe PMC
Mitochondrial and Cell Death Mechanisms in Neurodegenerative Diseases.
Pharmaceuticals (Basel), 3(4):839-915, 01 Jan 2010
Cited by: 109 articles | PMID: 21258649 | PMCID: PMC3023298
Review Free full text in Europe PMC
Mitochondriopathy in Parkinson disease and amyotrophic lateral sclerosis.
J Neuropathol Exp Neurol, 65(12):1103-1110, 01 Dec 2006
Cited by: 47 articles | PMID: 17146283
Review
Funding
Funders who supported this work.
NIA NIH HHS (2)
Grant ID: AG016282
Grant ID: R01 AG016282
NINDS NIH HHS (5)
Grant ID: NS052098
Grant ID: R01 NS052098
Grant ID: R01 NS065895
Grant ID: NS065895
Grant ID: R01 NS034100