Abstract
Free full text

mTORC1 drives HIF-1α and VEGF-A signalling via multiple mechanisms involving 4E-BP1, S6K1 and STAT3
Associated Data
Abstract
Recent clinical trials using rapalogues in tuberous sclerosis complex (TSC) show regression in volume of typically vascularised tumours including angiomyolipomas (AMLs) and sub-ependymal giant cell astrocytomas (SEGAs). By blocking mechanistic/mammalian target of rapamycin complex 1 (mTORC1) signalling, rapalogue efficacy is likely to occur in part through suppression of hypoxia inducible factors (HIFs) and vascular endothelial growth factors (VEGFs). We show that rapamycin reduces HIF-1α protein levels, and to a lesser extent VEGF-A levels, in renal cystadenoma cells in a Tsc2+/− mouse model. We establish that mTORC1 drives HIF-1α protein accumulation through enhanced transcription of HIF-1α mRNA, a process that is blocked by either inhibition or knockdown of signal transducer and activation of transcription 3 (STAT3). Furthermore, we demonstrate that STAT3 is directly phosphorylated by mTORC1 on Ser727 during hypoxia, promoting HIF-1α mRNA transcription. mTORC1 also regulates HIF-1α synthesis on a translational level via co-operative regulation of both initiation factor 4E-binding protein 1 (4E-BP1) and ribosomal protein S6 kinase-1 (S6K1), whilst HIF-1α degradation remains unaffected. We therefore propose that mTORC1 drives HIF-1α synthesis in a multi-faceted manner through 4E-BP1/eIF4E, S6K1 and STAT3. Interestingly, we observe a disconnect between HIF-1α protein levels and VEGF-A expression. While both S6K1 and 4E-BP1 regulate HIF-1α translation, VEGF-A is primarily under the control of 4E-BP1/eIF4E. S6K1 inhibition reduces HIF-1α but not VEGF-A expression, suggesting that mTORC1 mediates VEGF-A expression via both HIF-1α-dependent and -independent mechanisms. Our work has important implications for the treatment of vascularised tumours, where mTORC1 acts as a central mediator of STAT3, HIF-1α, VEGF-A and angiogenesis via multiple signalling mechanisms.
Introduction
Tumour vascularisation is regulated by hypoxia inducible factor (HIF) proteins. HIFs drive expression of over 800 different genes, many of which control cellular processes involved in cancer progression.1 As well as promoting angiogenesis, HIF activation alters cellular metabolism resulting in enhanced glycolytic flux promoting survival under hypoxia.2 Inappropriate activation of HIF-1α under normoxia causes a metabolic shift towards aerobic glycolysis, driving the Warburg effect which is characteristic of tumour cells.3 HIFs also promote cell proliferation, survival and metastasis through secretion of growth factors,4 motility and permeability factors5 as well as expression of genes involved in the degradation/remodelling of the extracellular matrix.6, 7
HIF is centrally involved in the pathology of a number of inherited diseases that predispose patients to renal cell carcinoma (RCC), including Von Hippel-Lindau disease (VHL),8 Hereditary Leiomyomatosis and Renal Cell Cancer (HLRCC),9 and Birt-Hogg-Dubé (BHD) syndrome.10 In VHL, loss of VHL protein function prevents ubiquitin-targeted proteasomal removal of HIF, resulting in inappropriate accumulation of HIF protein under normoxia.11, 12 Likewise in HLRCC, loss of fumarate hydratase activity and a build-up of intracellular fumarate9 stablises HIF protein and drives RCC.
Tuberous Sclerosis Complex (TSC) is a genetic disorder that predisposes patients to non-malignant tumours in multiple organ systems. These include angiomyolipomas (AML) of the kidneys, cutaneous angiofibromas, subependymal nodules (SENs) or sub-ependymal giant cell astrocytomas (SEGAs) and cortical tubers in the brain, cardiac rhabdomyomas, lymphangioleiomyomatosis (LAM) in the lungs, and hamartomas in the eye (see review13). TSC-associated tumours are highly vascularised and express elevated levels of CD31, a marker of the vascular endothelium, and the angiogenic marker VEGF.14 In TSC, angiogenesis is thought to be mediated via increased signal transduction through Rheb and mammalian target of rapamycin complex 1 (mTORC1). Rheb/mTORC1 is normally repressed by the TSC:TBC tumour suppressor complex, that is functionally lost in TSC tumours.15 Indeed, regression of tumour volume is apparent in TSC patients16, 17 and mouse models18 after mTORC1 inhibition. Angiogenesis inhibitors have also demonstrated therapeutic benefit and increased survival in TSC mouse models.19 Given that the anti-angiogenic effects of rapamycin are well established in a variety of diseases,20-22 there is a surprising lack of clarity within the literature regarding the mechanisms behind mTORC1-mediated angiogenesis.
mTORC1 is comprised of the serine/threonine protein kinase mTOR, Lst8 and Raptor. Raptor associates with mTORC1 substrates through mTOR signalling (TOS) motifs to facilitate their recruitment and phosphorylation.23 Inappropriate activation of mTORC1 has been reported in a wide variety of malignancies and mTOR inhibitors are currently licensed or undergoing clinical trials for several different cancer types.24 We previously demonstrated that HIF-1α is modulated by mTORC1, with Rheb over-expression propagating high levels of HIF transcriptional activity in a rapamycin sensitive fashion.25 Subsequent studies have supported this finding, however there is a significant degree of conflict within the literature regarding the mechanism by which mTORC1 regulates HIF-1α. For example, Hudson et al. showed evidence that HIF-1α stability was reduced by rapamycin but HIF-1α translation and transcription were unaffected.26 By contrast, Duvel et al. showed evidence that regulation of HIF-1α downstream of mTORC1 was primarily the result of increased 4E-BP1 phosphorylation and cap-dependent translation.27 Tandon et al. suggested that mTORC1 regulated the translation of HIF-1α via S6K1. They showed that shRNA mediated knockdown of S6K1 had comparable affects to rapamycin on HIF-1α activity in PTEN deficient cells.28 Active mTORC1 has also been reported to increase HIF-1α mRNA levels in cells devoid of TSC2,27, 29 indicative of transcriptional control. Conversely, a study using human SN12C renal cell carcinoma cells with and without VHL indicated that HIF-1α mRNA levels were insensitive to mTOR inhibition,30 while Treins et al. found that HIF-1α mRNA was not increased with insulin stimulation,31 suggesting mTORC1 independence.
Whilst it is clear that mTORC1 plays a fundamental role in tumour angiogenesis, the mechanisms underpinning this are unclear and a complete analysis of these mechanisms is absent from the literature. Through various lines of investigation, we systematically challenge a series of potential mechanisms by which mTORC1 might regulate HIF-1α. By utilising an array of different assays to explore HIF-1α activity and mTORC1 signalling through S6K1, 4E-BP1, and STAT3, we provide evidence that underpins a better understanding of how mTORC1 regulates angiogenesis.
Results
Rapamycin reduces HIF-1α and VEGF-A expression in renal cystadenoma cells from Tsc2+/− mice
We previously demonstrated that HIF-1α activity was markedly elevated in Tsc2−/− cell lines and sensitive to rapamycin inhibition.25 We therefore examined the effects of rapamycin on the expression of HIF-1α and its gene target VEGF-A in a Tsc2+/− mouse model (described here32) (Figure 1). These mice exhibit reduced Tsc2 expression in renal cystadenomas, as demonstrated in Figure 1A.
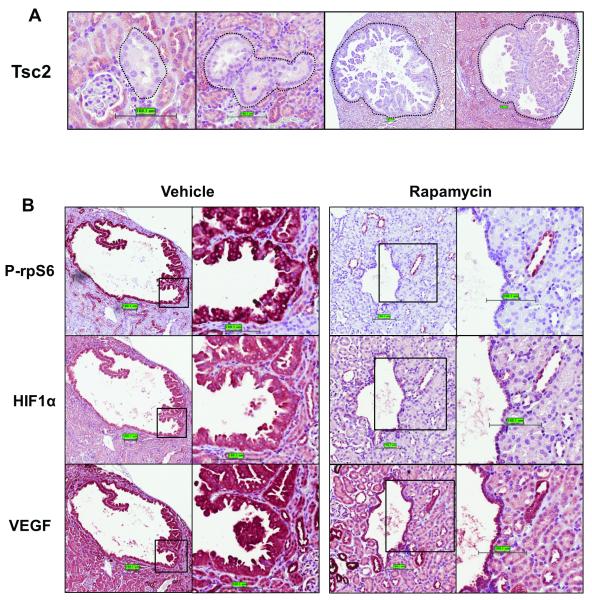
A: Paraffin-embedded kidney sections from five 12 month old Tsc2+/− mice were analysed by IHC. Thirty five cystadenomas were analysed for Tsc2 expression. Tsc2 expression was either undetectable or markedly reduced within these lesions. B: Tsc2+/− mice of 12 months old were treated with 10 mg/kg rapamycin (n=4) or vehicle (n=4) for two months. Paraffin-embedded kidney sections were stained using IHC with an antibody against phosphor-rpS6 (Ser235/236), HIF-1α or VEGF-A. Thirty renal lesions were scored for each treatment group. All lesions from mice treated with rapamycin showed a significant reduction in phospho-rpS6 and HIF-1α. VEGF-A was also reduced by rapamycin treatment although to a lesser extent.
Twelve month old Tsc2+/− mice were treated with 10 mg/kg rapamycin 5 times a week (or with vehicle as a control) for 2 months. Kidney sections were subjected to immunohistochemical (IHC) analysis to assess HIF-1α and VEGF-A protein expression (Figure 1B). Ribosomal protein S6 (rpS6) phosphorylation was also analysed as a read-out of mTORC1 signalling. Strong staining of HIF-1α and VEGF-A total protein as well as phosphorylated rpS6 was apparent in the cells lining and projecting into the lumen of renal cysts. Rapamycin potently reduced HIF-1α protein expression (as well as phosphorylation of rpS6) and VEGF-A expression was also reduced by rapamycin, albeit to a lesser extent. Brugarolas et al.29 previously reported that VEGF-A expression was relatively insensitive to rapamycin in cell line models for TSC, we show that this is also the case in this TSC mouse model.
HIF-1α is potently induced by active mTOR mutants
To explore this mechanism further, we employed cell line models allowing us to manipulate specific facets of mTORC1 signalling. We first utilised two constitutively active mTOR mutants; L1460P and E2419K (see Figure 2A) originally identified in yeast by Urano et al.33 By expressing these mutants alongside a luciferase reporter vector driven by HIF-1α response elements, we were able to specifically examine the effects of mTOR on HIF-1α. As Figure 2B demonstrates, we observed a 3-fold increase in HIF-1α activity in cells expressing the active mutants compared to wild-type mTOR. As mTORC1 activity is dependent upon an amino acid input (for review see34), and HIF-1α regulation has not been studied in the context of amino acid availability, we utilised these mutants to examine the effects of nutrient withdrawal upon HIF-1α. Nutrient withdrawal potently inhibited HIF-1α activity in cells expressing either wild-type or the E2419K mutant of mTOR. In concordance with our previous study, 35 HIF-1α activity was also partially resistant to amino acid deprivation in cells expressing L1460P. This finding provides the first direct evidence that amino acids regulate HIF-1α activity via an mTORC1-dependent mechanism. Given that HIF-1α activity in cells expressing the L1460P mutant was partially insensitive to nutrient withdrawal, it is possible that the L1460P mutant is inappropriately targeted to the lysosomes and could therefore be a useful tool for investigating amino acid signalling to mTORC1. Whilst these data clearly show that mTORC1 regulates HIF-1α, it remained unclear how this occurs. Figure 2C indicates possible mechanisms underlying this regulation.
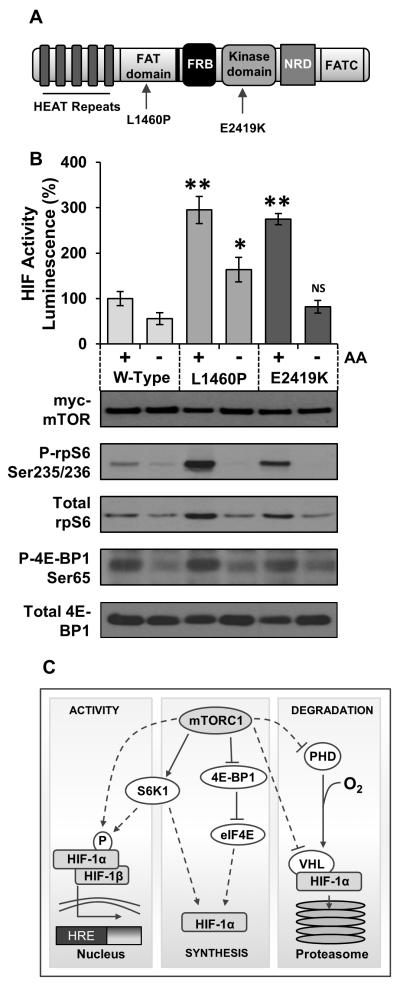
A: mTOR schematic indicating conserved regions and mutation positions. Domains: FRB refers to FKBP12/Rapamycin binding domain, NRD: Negative regulatory domain. B: HEK293 cells transfected with mTOR mutants as indicated in conjunction with the HIF-1α inducible luciferase reporter. Cells were starved of amino acids (AA) and cultured in media containing 1 mM DMOG (to mimic hypoxia) for 4 h. Cells were stimulated with 10 μg/ml insulin 15 min prior to lysis. Luminescence was measured and standardised to total protein as determined by a Bradford assay. Error bars indicate standard deviation between three independent experiments. C: mTORC1 may be able to regulate the synthesis, degradation or activity of HIF-1α. There are several different ways in which this may occur; dotted lines indicate potential mechanisms of regulation whilst solid lines indicate known facets of the pathway. ** = p < 0.001, * = p < 0.05 levels of significance when compared to wild-type. NS = not significantly different to wild-type.
Rapamycin does not affect the stability of HIF-1α protein
We next analysed the effects of rapamycin upon endogenous HIF-1α protein accumulation in HEK293 cells under hypoxia (Figure 3A). Cycloheximide, which blocks ribosomal translocation, was used as a control to prevent de novo protein synthesis of HIF-1α. As Figure 3A indicates, cycloheximide pre-treatment was sufficient to completely abolish HIF-1α protein synthesis under hypoxia., Rapamycin pre-treatment caused a reduction in HIF-1α protein levels by approximately one third at all time points (Figure 3A), clearly demonstrating that mTORC1 promotes accumulation of HIF-1α protein. Hudson et al.’s work indicated that rapamycin decreased the stability of HIF-1α,26 whereas other groups have suggested that rapamycin reduces the synthesis of HIF-1α at a transcriptional or translational level.26, 27, 36
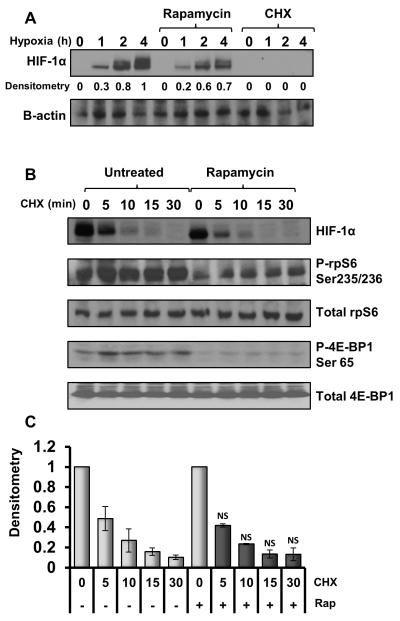
A: HEK293 cells were treated with vehicle, 10 μg/ml cycloheximide or 50 nM rapamycin for 30 min prior to hypoxic incubation for the given time points. B: HEK293 cells were cultured under hypoxia for 2 h to allow build-up of HIF-1α protein then pre-treated with 50 nM rapamycin or vehicle for 30 min. 10 μg/ml cycloheximide was added to the cells before hypoxic incubation for the given time points. Lysates were sonicated and analysed for HIF-1α and β-actin protein levels using western blotting. C: HIF-1α protein levels from Figure 3B were analysed using densitometry. Data was collated from three individually repeated experiments and analysed for statistical significance. No difference in the half-life of HIF-1α was observed between the rapamycin (rap) treated and untreated samples. (NS indicates not significant when compared with untreated equivalent time point.)
As cycloheximide completely blocked HIF-1α synthesis, a pulse-chase experiment was performed in hypoxic HEK293 cells in the presence and absence of rapamycin (Figure 3B) to determine whether HIF-1α stability is affected by rapamycin. In the absence of de novo HIF-1α protein synthesis, HIF-1α levels decreased rapidly with a loss of approximately 50% of HIF-1α protein after just 5 min. This rapid reduction in HIF-1α protein may suggest residual activity of prolyl-hydroxylase enzymes, even under hypoxia. Importantly, however, rapamycin did not impact the half-life of HIF-1α. Furthermore, inhibition of mTOR with the ATP-competitive inhibitor, KU-0063794, had no additional effect upon the stability of HIF-1α when compared to rapamycin (see supplementary data figure 1). Densitometry analysis of the three independent experiments further supported this conclusion (Figure 3C) as no significant differences were observed between the rapamycin treated and pre-treated samples at any of the time-points analysed. Our work determines that mTORC1 promotes HIF-1α through increased synthesis rather than reduced degradation, in support of the work by Duvel and Tandon et al.27, 36
Both rapamycin and KU-0063794 suppress HIF-1α protein accumulation
We next analysed HIF-1α protein levels in hypoxic HEK293 cells expressing the E2419K active mutant of mTOR. The E2419K mutant increased endogenous HIF-1α protein levels by approximately one-third after 2 h of hypoxia (Figure 4A), suggesting that during the initial stages of hypoxia, mTORC1 activation promotes HIF-1α synthesis to elicit the hypoxic response. Phosphorylation of 4E-BP1 and rpS6 were also analysed to demonstrate upregulation of mTORC1 in the presence of the active E2419K mutant. Interestingly, we observed fluctuations in 4E-BP1 Ser65 phosphorylation under hypoxia (Figure 4A); this is likely to be the result of feedback inhibition to mTORC1 from the HIF-1α target REDD1.37 rpS6 phosphorylation and HIF-1α protein levels were maintained indicating that mTORC1 is not fully suppressed.
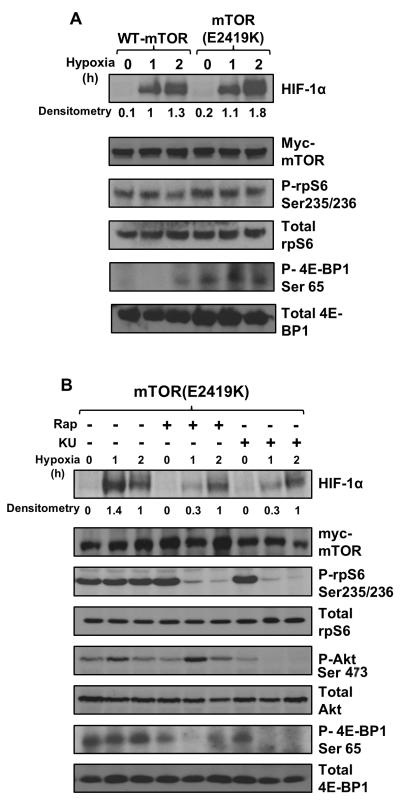
A: HEK293 cells were transfected with wild-type or the active mutant of mTOR (E2419K) then placed into the hypoxic chamber for the given time points. Lysates were sonicated and analysed for HIF-1α protein levels, as well as phosphorylated and total 4E-BP1 and rpS6 to indicate mTORC1 activity. Result shown is representative of three independent replicated experiments. B: HEK293 cells expressing the active mutant were treated with 50 nM rapamycin (rap) or 1 μM KU-0063794 (KU) for 1 h prior to hypoxic exposure and a time course was performed as described in 4A. Akt phosphorylation at Ser473 was used as a readout for mTORC2 activity whilst phospho-rpS6 and phospho-4E-BP1 were used as indicators of mTORC1 activity.
Previous work demonstrates that some substrates of mTORC1 are more robustly inhibited with mTOR kinase inhibitors as opposed to rapalogues.38, 39 To determine whether mTOR kinase inhibitors may be more effective than rapamycin at suppressing HIF-1α, we compared the effects of rapamycin to the ATP-competitive KU-0063794 mTOR kinase inhibitor (Figure 4B).40 Treatment with KU-0063794 suppressed Akt phosphorylation at the mTORC2 site (Ser473), as well as reducing rpS6 phosphorylation, indicating suppression of both mTOR complexes. Ser65 phosphorylation of 4E-BP1 was partially rapamycin insensitive, as has previously been reported.41 Interestingly, we observed complete recovery of 4E-BP1 phosphorylation in just 2 h following rapamycin treatment. By contrast, Choo et al. found that this recovery took approximately 6 h. It is likely that in our experiment, over-expression of the active mTOR mutant was associated with a faster recovery from rapamycin repression.
mTORC1 inhibition with rapamycin caused a significant suppression in HIF-1α activity (as before). However, no further inhibition of the HIF-1α protein level was observed with KU-0063794 compared to rapamycin. This is consistent with mTORC1, rather than mTORC2, driving the accumulation of HIF-1α protein. Also consistent with previous observations, rapamycin treatment caused a slight increase in Akt phosphorylation, which is likely to be a result of reduced negative feedback signalling to IRS-1 via S6K1.42
Active S6K1 promotes HIF-1α protein accumulation
As depicted in Figure 2C, mTORC1 may promote HIF-1α synthesis via enhanced protein translation. S6K1 promotes protein translation through phosphorylation of numerous eukaryotic initiation factors43-45 and may drive HIF-1α protein synthesis in this manner. Tandon et al. reported that S6K1 was essential for the translation of HIF-1α,36 whereas Duvel et al. showed that S6K1 knockdown had no effect on a translational luciferase reporter driven by the HIF 5′UTR.27
To address this conflict and assess the involvement of S6K1 in HIF-1α regulation, we utilised a constitutively active S6K1 mutant (F5A-E389-R3A see Figure 5A). F5A-E389-R3A contains a mutation within the mTORC1 signalling (TOS) motif, a triple mutation to the ‘RSPRR’ motif which acts as an mTORC1-mediated auto-inhibitory domain, as well as an E389 phospho-mimetic mutation within the linker region of S6K1. This F5A-E389-R3A mutant therefore drives a constitutively high level of S6K1 activity in cells regardless of mTORC1 activity.46 HEK293 cells were transfected with either pRK7 or HAS6K1-F5A-E389-R3A and cultured under hypoxia. HIF-1α protein accumulation was determined over a 4 h time-course. Expression of the active S6K1 mutant caused an approximate 2-fold increase in HIF-1α protein when compared to empty vector (Figure 5B). Conversely, the impact upon VEGF-A expression was not as dramatic, with the active S6K1 mutant causing only a slight increase in protein levels of VEGF-A. To confirm this effect, HEK293 cells were treated for 1 h prior to hypoxic incubation with the highly specific S6K1 inhibitor, PF-4708671.47 As Figure 5D demonstrates, HIF-1α protein levels were highly sensitive to S6K1 inhibition, however VEGF-A expression levels were maintained despite the drop in HIF-1α protein. This suggests that VEGF-A expression is mediated via both HIF-1α-dependent and -independent mechanisms, as has previously been reported within the literature.48

A: Schematic showing mutant constructs of S6K1 utilised, indicating conserved domains and mutated regions. B: HEK293s were transfected with either pRK7 or HA-S6K1-F5A-E389-R3A and subjected to hypoxia for the given time points prior to lysis. HIF-1α protein levels were quantified using western blotting and imageJ software for densitometry analysis. Result is representative of three independent experiments. C: Untransfected HEK293 cells were treated for 1 h with 10 μM PF-4708671 and 15 min with 10 μg/ml insulin (ins) prior to 4 h hypoxic/normoxic incubation. Cells were lysed directly in sample buffer, sonicated and analysed for protein levels using western blotting. Densitometry analysis was performed using imageJ software. D: Duplicate HEK293 cells expressing either empty vector, wild-type or active S6K1 mutant were treated with vehicle or 10 μM PF-4708671 as indicated and incubated in the hypoxic chamber for 1 hr. mRNA was extracted from half the lysates and Q-PCR was performed to determine HIF-1α mRNA levels, standardised to β-actin. The remaining lysates were sonicated and analysed by western blotting for HIF-1α expression and S6K1 activity. NS indicates no significant changes in HIF-1α mRNA levels under any condition.
In order to determine whether S6K1 modulated HIF-1α expression on a transcriptional and/or translational level, HIF-1α mRNA levels were also examined. Figure 5D demonstrates that HIF-1α mRNA levels are unaffected by either expression of the active S6K1 mutant or inhibition of S6K1 with PF-4708671, indicating that S6K1 mediates HIF-1α protein expression solely through effects on protein translation. Interestingly, PF-4708671 acted as a dominant inhibitor of HIF-1α, reducing HIF-1α protein expression to a level lower than cells expressing empty vector, even in the absence of insulin stimulation. PF-4708671 also caused an increase in S6K1 Thr389 phosphorylation, as previously reported.47 Phosphorylation of rpS6 was unaffected by PF-4708671 in the absence of insulin stimulation (Figure 5E), indicating that another kinase such as RSK or S6K2 may be modulating rpS6 phosphorylation under these conditions. Consistent with a study by Pearce et al.,47 we also observed that Thr389 phosphorylation of S6K1 was induced upon treatment with PF-4708671 under serum-starved conditions.
eIF4E availability is a rate limiting factor in the synthesis of both HIF-1α and VEGF-A
We next assessed 4E-BP1’s role as a negative regulator of HIF-1α through inhibition of eIF4E. eIF4E availability is considered a rate-limiting factor in 5′-cap-dependent translation downstream of mTORC1.49 Duvel et al. showed that 4E-BP1 represses HIF-1α by acting as a negative regulator of eIF4E,27 but in contrast Hudson et al. observed no effects of rapamycin treatment on HIF-1α translation.26 To examine whether inhibition of eIF4E could modulate HIF-1α activity, Tsc2−/− MEFs, which exhibit constitutively active mTORC1 signalling, were transfected with the HIF-1α luciferase reporter alongside mutant or wild-type 4E-BP1 and cultured under hypoxia. Two mutants of 4E-BP1 were utilised; 4E-BP1(F114A), which contains a mutation within the TOS motif (and so cannot be phosphorylated by mTORC1 and dominantly inhibits eIF4E), and the Y54A/L59A mutant which contains mutations within the eIF4E-binding domain. The 4E-BP1 Y54A/L59A mutant serves as a negative control as it is unable to interact with eIF4E. As expected; 4E-BP1(F114A) was less phosphorylated and consequently bound more avidly to eIF4E when compared to wild-type 4E-BP1 (Figure 6A). The 4E-BP1(F114A) TOS mutant, which potently bound to eIF4E, repressed the activity of HIF-1α by approximately one third compared with the wild-type (35 ±2%). In contrast, both the Y54A/L59A mutant and wild-type 4E-BP1 had minimal effects upon HIF-1α activity. The 4E-BP1(F114A) TOS-mutant was also able to suppress HIF-1α protein accumulation in the HEK293 cells, as demonstrated by the hypoxic time course in Figure 6C. There was an approximate 50% reduction in HIF-1α protein levels after 2 h of hypoxia in cells expressing 4E-BP1(F114A). This result implies that mTORC1 mediated phosphorylation of 4E-BP1 is a critical factor driving HIF-1α protein synthesis. In contrast to the effects of S6K1 inhibition, expression of the 4E-BP1(F114A) mutant caused a significant reduction in VEGF-A expression (Figure 6C), indicating that repression of 4E-BP1 by mTORC1 is crucial for the VEGF-A response. HIF-1α mRNA levels were unaffected by expression of the 4E-BP1(F114A) mutant (Figure 6D), suggesting that, in a manner analogous to S6K1, this regulation is also on a translational level. These data suggests that mTORC1 mediates HIF-1α activity by promoting 5′-cap-dependent translation through both 4E-BP1/eIF4E and S6K1, while the translation of VEGF-A is primarily regulated by 4E-BP1/eIF4E and not S6K1.
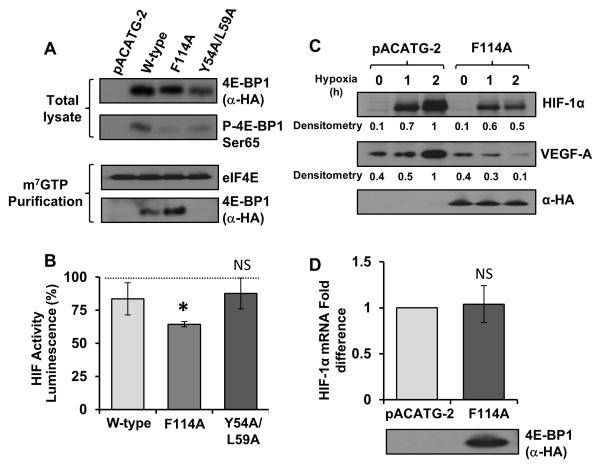
A & B: Tsc2−/− MEFs were transfected with either pACATG-2 or mutant constructs of 4E-BP1 alongside the HIF-1α luciferase reporter. Lysates were analysed for HIF-1α induced luciferase activity (standardised to total protein levels). Graph indicates results from three independent experiments. eIF4E was immunoprecipitated from the remainder of the lysates cells using m7GTP beads and 4E-BP1 binding was assessed using western blotting. C: The TOS-mutant of 4E-BP1 was over-expressed in HEK293 cells cultured under hypoxia for the given time points. Lysates were analysed for total HIF-1α and VEGF-A levels using western blotting. D: HEK293 cells expressing empty vector/4E-BP1 mutant were cultured under hypoxia for 4 h, mRNA was extracted from the total lysates and Q-PCR was performed to determine HIF-1α mRNA levels (standardised to β-actin). * = p-value < 0.05, levels of significance when compared with wild-type, NS = not significant when compared with empty vector control.
mTORC1 drives the transcription of HIF-1α via STAT3
To further investigate mTORC1 involvement in HIF-1α mRNA transcription, HEK293 cells were transfected with the active mTOR(E2491K) mutant and insulin stimulated (to promote maximal activation of mTORC1) in the presence or absence of rapamycin. Q-PCR analysis revealed a substantial rapamycin sensitive increase in HIF-1α mRNA levels in response to insulin, indicating a transcriptional facet of control through mTORC1 (Figure 7A). Although elevation of HIF-1α mRNA levels has been previously reported within Tsc2−/− MEFs,27, 29 the mechanism driving HIF-1α mRNA transcription downstream of mTORC1 is unknown. We hypothesised that another downstream signalling target of mTORC1 may be involved in this regulation. Previous work in tumour cells reported links between HIF-1α and STAT3.50 Furthermore, Yokogami et al. suggested a link between mTORC1 and STAT3, demonstrating phosphorylation of a C-terminal STAT3 peptide using a crude in vitro mTOR kinase assay.51

A: HEK293 cells expressing empty vector or the active mutant of mTOR were cultured under hypoxia in the presence of insulin and or rapamycin as indicated. mRNA was extracted from total lysates, HIF-1α mRNA was quantified as standardised to β-actin. B & C: Untransfected HEK293 cells were treated with rapamycin/STAT3 inhibitors for 45 min prior to 30 min of insulin/CNTF stimulation (as indicated). (B) mRNA was extracted from total lysates and quantified as above (C). Lysates were sonicated and analysed using western blotting. ImageJ software was utilised to analyse HIF-1α protein levels via densitometry (** = significant difference p-value < 0.001).
To determine whether STAT3 is involved in HIF-1α mRNA expression, HEK293 cells were pre-treated with either rapamycin, or STAT3 inhibitors: FLLL31 (an upstream JAK2 inhibitor) and 5,15-diphenylporphyrin (5,15,DPP), an Src homology-2 domain (SH2) antagonist in the presence of insulin (Figure 7B). CNTF (ciliary neurotrophic factor) was also utilised to stimulate STAT3 Tyr705 phosphorylation as this is considered to be a priming event required for Ser727 phosphorylation by mTORC1.52 Both STAT3 inhibitors were shown to potently block insulin induced expression of HIF-1α mRNA (Figure 7B). This result suggests that mTORC1 promotes HIF-1α mRNA expression in a STAT3-dependent manner. HIF-1α protein levels were also examined to verify this finding (Figure 7C). Both FLLL31 and 5,15,DPP treatment caused a suppression in HIF-1α protein levels, further supporting the conclusion that STAT3 drives the expression of HIF-1α mRNA. Intriguingly, the curcumin analogue, FLLL31, had a dramatic dominant inhibitory effect on HIF-1α, reducing expression levels by approximately 80%. In contrast, both rapamycin and 5,15,DPP treatment both caused an approximate 30% reduction in HIF-1α protein expression. Analysis of rpS6, 4E-BP1 and STAT3 Tyr705 phosphorylation in these cells revealed that this was due to dual suppression of both JAK2/STAT3 and mTORC1 signalling pathways by FLLL31. Although further studies are required, repression of HIF-1α protein levels through the capacity of FLLL31 to inhibit both JAK2/STAT3 and mTORC1 may have therapeutic advantages in the treatment of vascularised tumours where mTORC1 is active.
STAT3 is a direct substrate for mTORC1
Previous research by Yokogami et al. suggested that STAT3 may be a direct substrate for mTORC1.51 In support of these initial findings, our data indicates that STAT3 is regulated downstream of mTORC1. Other studies have also shown upregulation of STAT3 signalling in cell line models for TSC,53, 54 however the direct phosphorylation of STAT3 by mTORC1 has not been verified since the original study by Yokogami et al. fourteen years ago.51 It is important to note that the mTOR kinase assay employed by Yokogami et al. was not optimal; for instance, a short STAT3 peptide (which did not contain any potential Raptor interacting motifs for substrate recognition) was assayed alongside over-expressed mTOR in the absence of Raptor, using unphysiological MnCl2 and high ATP levels to artificially enhance kinase activity. We therefore utilised our previously optimised mTORC1 specific kinase assay,55 with more physiologically relevant assay conditions to verify whether full length recombinant STAT3 is indeed a direct substrate for mTORC1. We observed direct phosphorylation of STAT3 at Ser727 by mTORC1 (Figure 8A). In this in vitro mTORC1 assay, Rheb-GTP potently induced the activity of mTORC1 towards 4E-BP1 (employed as a control substrate) and STAT3, revealing that mTORC1 can directly phosphorylate STAT3 on Ser727.

A: mTORC1 kinase assay: An active mTORC1 complex was purified from insulin stimulated HEK293 cells grown under serum-starved conditions as described.35 GST-STAT3 and GST-4E-BP1 were purified from serum-starved HEK293 cells, as was GST-Rheb, which was then loaded with GTPγS. Purified mTORC1 complex was incubated alongside potential substrates with and without GTPγS-Rheb (as indicated) for 1 h at 30°C with gentle agitation. SDS-PAGE and western blotting with phospho-specific antibodies was utilised to determine specific phosphorylation events as well as mTOR/Raptor purification. B: HEK293 cells were transfected with either Non-target control or STAT3 shRNA and treated with either 10 μg/ml insulin, 50 nM rapamycin (rap) or 1 μM KU-0063794 (KU). Western blotting was utilised to determine the efficiency of STAT3 knockdown and downstream effects. Q-PCR was utilised to determine HIF-1α mRNA levels (standardised to β-actin). No significant differences were observed between insulin stimulation or mTORC1 inhibition with rap/KU (indicated by NS). C: Schematic showing multiple mTORC1 inputs into angiogenesis. As demonstrated in this study, mTORC1 regulates the translation of HIF-1α via both 4E-BP1/eIF4E and S6K1. Although S6K1 can also promote HIF-1α translation, VEGF-A does not appear to be directly affected by active S6K1. mTORC1 also controls the transcription of HIF-1α mRNA in a rapamycin sensitive fashion via STAT3 phosphorylation, indicating that mTORC1 mediates angiogenesis via three distinct mechanisms.
To confirm the involvement of STAT3 in the regulation of HIF-1α by mTORC1, we knocked down STAT3 in HEK293 cells and examined the levels of HIF-1α mRNA after insulin stimulation (Figure 8B). Under starved conditions, STAT3 knockdown substantially reduced the mRNA and protein levels of HIF-1α. Importantly, STAT3 knockdown completely abolished insulin-induced HIF-1α mRNA expression (as observed in Figure 7A), which was not further reduced upon mTORC1 inhibition with either rapamycin or KU-0063794. Collectively, our data supports a new signalling mechanism where mTORC1 mediates HIF-1α transcription via STAT3 (see mechanistic Figure 8C).
Discussion
Although mTORC1 is known to potently activate HIF-1α,25 the mechanism(s) governing this regulation have been poorly defined. We designed this study to systematically examine specific facets of mTORC1-mediated angiogenesis to better understand this process. Although previous studies have suggested roles for either S6K1 or 4E-BP1 in driving HIF-1α activity, few studies have investigated the effects of both. We have used a variety of methodologies to characterise angiogenic signalling downstream of mTORC1 and show for the first time that both 4E-BP1 and S6K1 promote the translation of HIF-1α. Furthermore, we confirm previous reports that mTORC1 drives HIF-1α translation and uncover the mechanism by which this occurs. We show that mTORC1 promotes the phosphorylation of STAT3 at Ser727 during hypoxia to promote HIF-1α transcription, and that STAT3 inhibition effectively represses insulin induced HIF-1α transcription in a manner similar to rapamycin. STAT3 knockdown also caused a significant reduction in HIF-1α mRNA levels, and resulted in a loss of HIF-1α transcriptional control via mTORC1, confirming that STAT3 is required for mTORC1-mediated HIF-1α transcription. Interestingly, our data reveals that STAT3 is not as robustly phosphorylated by mTORC1 as the well-characterised substrate 4E-BP1 (at least in the in vitro mTORC1 kinase assay). This suggests a hierarchy of mTORC1 substrate phosphorylation, and could help explain differential responses to mTORC1 inhibition between substrates.56
Previous studies identified putative hypoxia response elements (HREs) in the HIF-1α promoter, suggesting an auto-regulatory loop whereby HIF-1α may up-regulate synthesis of its own mRNA.57 Our data indicate that this is unlikely, as expression of the S6K1 and 4E-BP1 mutants caused substantial effects on HIF-1α protein but not mRNA levels. We show that HIF-1α mRNA levels are suppressed with mTORC1/STAT3 inhibition and conclude that mTORC1 mediates HIF-1α transcription via STAT3. We also confirm that STAT3 is a direct substrate for mTORC1. Like HIF-1α, STAT3 mediates several cellular processes linked with cancer progression including cellular survival, proliferation and metastasis.58, 59 It is likely that upregulation of STAT3 contributes to the pathology of TSC-associated hamartomas and cancer progression in tumours where mTORC1 is active.
Previous studies demonstrated upregulation of STAT3 Tyr705 phosphorylation in mouse/cell line models for TSC.53, 60 Our results indicate that Tyr705 phosphorylation is insensitive to rapamycin inhibition. This suggests that STAT3 Tyr705 phosphorylation may be upregulated as a result of disrupted feedback mechanisms due to prolonged mTORC1 activation in the TSC models.
We demonstrate that the STAT3 inhibitor FLLL31 has significant inhibitory effects on HIF-1α transcription and translation, through dual suppression of both mTORC1 and STAT3 signalling pathways. FLLL31 may therefore be an appealing small molecule to explore in the treatment of vascularised tumours. FLLL31 is a derivative of curcumin found in the spice turmeric.61 The anti-cancer properties of curcumin are well documented in vivo but it has been unsuccessful in clinical trials due to poor bioavailability and solubility.62 FLLL31 was shown to have increased potency compared with curcumin and may therefore be more effective in vivo.61 Our data indicate that curcumin analogues may be effective therapeutic agents for targeting vascularised tumours where mTORC1 is active, however there is a need for the development of curcumin analogues with better bioavailability.
It is well documented that VHL mediates proteasomal degradation of HIF-1α under normoxia.11 However, VHL and oxygen independent mechanisms of HIF-1α degradation have also been reported.63 Hudson et al. reported evidence that rapamycin influences the stability of HIF-1α, while its transcription and translation were unaffected.26 By contrast we present evidence from multiple experiments (Figures 5, ,66 and and7)7) that both HIF-1α translation and transcription are subject to modification by mTORC1, in concordance with other studies examining HIF-1α synthesis.27, 31, 64 Furthermore, we observe no difference in the half-life of HIF-1α protein in rapamycin treated and untreated cells. We therefore conclude that mTORC1 regulates the synthesis of HIF-1α (via both mRNA transcription and protein translation) whilst VHL mediates HIF-1α protein turnover (see Figure 8C).
S6K1 regulates a number of translation initiation factors, including eIF3,43 eIF4B,65 and elongation factor 2 kinase (eEF2k)66 and may enhance HIF-1α translation via these substrates. The specific S6K1 inhibitor PF-4708671 had a dramatic impact on HIF-1α protein levels whilst HIF-1α mRNA levels were maintained, indicating that S6K1 promotes HIF-1α translation but not its transcription. Interestingly, VEGF-A levels were insensitive to inhibition or activation of S6K1, while expression of the dominant inhibitory 4E-BP1 mutant had a dramatic impact upon VEGF-A levels. This indicates that mTORC1/4E-BP1 signalling drives VEGF-A expression via both HIF-1α-dependent and -independent mechanisms. Given that rapamycin is known to cause incomplete suppression of eIF4E,67 this finding may explain why VEGF-A expression was less sensitive to rapamycin inhibition than HIF-1α and phospho-rpS6 expression in the Tsc2+/− mice. Our data suggests that in the treatment of vascularised tumours, targeting eIF4E in combination with rapamycin may be a more effective strategy for reducing VEGF-A expression.
Previous work by Thomas et al. suggested that HIF-1α may be preferentially translated downstream of mTORC1 as a TOP (tract of pyrimidine) mRNA.30 Two recent studies used ribosomal profiling to produce a unified model for 5′-TOP mRNA translation. These studies demonstrated that mTORC1 promotes the translation of TOP mRNAs via 4E-BP1 phosphorylation and eIF4E availability.68, 69 Given that we observed S6K1 involvement in HIF-1α activity, as well as an mTORC1-dependent STAT3 input into HIF-1α mRNA transcription, it is likely that the rate of HIF-1α translation is proportional to the abundance of HIF-1α mRNA, rather than any selective qualities conferred by its mRNA structure. This is in concordance with current thinking that HIF-1α is not a genuine 5′-TOP mRNA.70
Our findings suggest that STAT3, 4E-BP1, and S6K1 act in concert to drive the angiogenic response through mTORC1, placing mTORC1 as a central mediator of the hypoxic response via multiple signalling outputs. Figure 8C indicates the mechanisms for the response that we delineated in the current study. Recent evidence has suggested that mRNAs translated by mTORC1 are regulated via eIF4E availability, and can be stratified into functional groups controlling oncogenic processes including cell proliferation, metabolism and invasion.69 We show evidence that not all the oncogenic signalling outputs downstream of mTORC1 are mediated solely by eIF4E availability, and that both S6K1 and 4E-BP1 facilitate the angiogenic response at a translational level.
Materials and Methods
Animal treatment and Immunohistochemistry (IHC)
Animal procedures were performed in accordance with the UK Home Office guidelines and approved by the Ethical Review Group of Cardiff University. Tsc2+/− mice (described here32) were treated with 10 mg/kg rapamycin/vehicle via intra-peritoneal injection 5 times a week for 2 months. Animals were humanely killed and kidney sections prepared for IHC analysis. Antibodies against HIF-1α, VEGF-A and phosphorylated rpS6 (S6 p-S235/236) (Cell Signalling Technology, Danvers, USA) were used for IHC. SignalStain Boost Rabbit specific IHC Detection Reagent (Cell Signalling Technology) was used for antigen staining. Briefly, paraffin-embedded mouse kidney sections were deparaffinised and rehydrated. Sections were boiled for 10 min in 10 mM sodium citrate buffer (pH 6.0). After 3 washes in TBST, sections were blocked in 1.5% (v/v) goat serum for 10 min at room temperature. Antigen staining was performed in accordance with manufacturer protocol.
Plasmid details
Myc-mTOR and active mutant constructs,55 HA-S6K1 constructs46 and 4E-BP1 constructs71 are described previously. The HIF-1α specific inducible luciferase reporter construct was purchased from Affymetrix Inc. (Milan, Italy). Gateway recombination cloning technology (Life Technologies, Paisley, UK) was utilised to generate GST-STAT3 from an I.M.A.G.E. clone (purchased from ATCC, Manassas, USA), in accordance with manufacturer guidelines. Both STAT3 shRNA (Clone ID: NM_003150.3-458s21c1) and non-target control MISSION shRNA (Clone ID: SHCO16) in the pLKO.1 vector were obtained from Sigma-Aldrich Company Ltd. (Dorset, UK).
Antibodies and biochemicals
Anti-myc, anti-rpS6 phospho-Ser235/236, anti-eIF4E, anti-4E-BP1 phospho-Ser65 and Thr36/45, anti-STAT3 Ser727 and Tyr705, and anti-β-actin antibodies were obtained from Cell Signalling Technology. Anti-Rheb was obtained from Santa Cruz (Heidelberg, Germany), anti-HA was obtained from Roche (Hertfordshire, UK), and Anti-HIF-1α was obtained from BD transduction laboratories (Oxford, UK). Rapamycin was obtained from Calbiochem (Nottingham, UK). Dimethyloxallyl Glycine (DMOG) was purchased from R & D systems (Minneapolis, USA). KU-0063794 was purchased from Chemquest Ltd. (Cheshire, UK). Cycloheximide, insulin, PF-4708671, 5,15-DPP, FLLL31 and all other general lab chemicals were obtained from Sigma-Aldrich unless otherwise stated.
Cell Culture and transfection
HEK293s, Tsc2−/− and Tsc2+/+ MEFs (a kind gift from Prof. D. Kwiatkowski, Harvard University, Boston) were cultured in Dulbecco’s Modified Eagle’s Medium (DMEM) supplemented with 10% (v/v) foetal calf serum and 100 μ/ml penicillin streptomycin (Life Technologies). Transfections were carried out using Lipofectamine 2000 in accordance with manufacturer protocol (Life Technologies). Reverse transfections for STAT3 knockdown were performed using JETPei transfection reagent (VWR International, Leicestershire, UK) in accordance with manufacturer protocol. Plates were coated with a solution of 20 μg/mL fibronectin (R & D Systems) to aid cell adherence during knockdown.
Time course experiments
HEK293 cells were transfected, drug treated as indicated and placed into a Binder CB150 hypoxic chamber set at 1 % O2 for the indicated time points. Cells were lysed directly in sample buffer (62.5 mM Tris HCL, 50 mM DTT, 2 % SDS (w/v), 10 % Glycerol (w/v), 0.1 % Bromophenol blue (w/v) pH 7.6) and sonicated for 5 × 20 s cycles on full power (30 amplitude microns) before analysis by western blotting.
Western Blotting
Lysates were resolved by SDS-PAGE and proteins were transferred to polyvinylidene difluoride membranes (Millipore, Edinburgh, UK). Membranes were blocked in 5 % (w/v) dry milk powder dissolved in Tris-buffered saline containing 0.1 % (v/v) Tween, then probed with primary antibody and horse radish peroxidase-conjugated secondary antibody (Sigma-Aldrich). Proteins were visualized using Enhanced Chemiluminescent solution and Hyperfilm (both GE Healthcare, Buckinghamshire, UK). All western blots are representative of at least three independent experiments. Where densitometry analysis is shown, imageJ 1.43v software was utilised to determine relative levels of HIF-1α, standardising the untreated control sample to 1 after either 1 or 2 h in hypoxia.
Luciferase Assays
These assays were carried out as described previously,55, 72 using the HIF-1α specific luciferase reporter construct (Affymetrix Inc.) and Promega dual-luciferase reporter assay system in accordance with manufacturer protocol (Promega, Southampton, UK).
mTORC1 kinase assays
Kinase assays were carried out as previously described.55 Recombinant GST-STAT3 was purified from rapamycin treated HEK293 cells and assayed in the presence and absence of 75 ng purified GST-Rheb for 1 h at 30°C with gentle agitation.
Quantitative PCR
Q-PCR was performed as previously described.10 Primer sets for HIF-1α (cat no. QT01039542) and β-actin (cat no. QT01680476) were purchased from Qiagen (Limburg, Netherlands) who retain the right to withhold primer sequence information. Fold change was calculated using the ddCT (delta-delta-Ct) method, standardised to β-actin. A dissociation step was performed to verify a single product was produced with each primer set. The size of PCR products was also verified by resolution on a 2% polyacrylamide gel with both HIF-1α and β-actin giving an approximate amplicon length of 104 bp. All Q-PCR primer assays in this study were at least 96% efficient.
Supplementary Material
1
Figure 1: No significant differences were observed in HIF-1α protein stability between rapamycin or KU-0063794 treated cells. HEK293 cells were cultured under hypoxia for 2 h to allow build-up of HIF-1α protein then pre-treated with 50 nM rapamycin or 1 μM KU-0063794 (KU) for 30 min. 10 μg/ml cycloheximide was added to the cells before hypoxic incubation for the given time points. Lysates were sonicated and analysed for HIF-1α and β-actin protein levels using western blotting. NS indicates no significant differences between equivalent time points.
Acknowledgments
This research was supported by the Association for International Cancer Research Career Development Fellowship [No. 06-914/915] (to A. Tee), a Junior Fellowship from the Tuberous Sclerosis Association [Ref: 2013-F02: Investigating mTORC1 independent functions of TSC2] (to K. Dodd) and a Cancer Research UK development fund (to J. Sampson and A. Tee). This work is further supported by the Welsh Government’s National Institute of Social Care and Health Research (NISCHR) through the Wales Gene Park and by the Tuberous Sclerosis Association.
Footnotes
Conflict of interest The authors declare no conflict of interest.
References
Full text links
Read article at publisher's site: https://doi.org/10.1038/onc.2014.164
Read article for free, from open access legal sources, via Unpaywall:
https://www.nature.com/articles/onc2014164.pdf
Citations & impact
Impact metrics
Citations of article over time
Alternative metrics
Smart citations by scite.ai
Explore citation contexts and check if this article has been
supported or disputed.
https://scite.ai/reports/10.1038/onc.2014.164
Article citations
Deciphering STAT3's negative regulation of LHPP in ESCC progression through single-cell transcriptomics analysis.
Mol Med, 30(1):192, 28 Oct 2024
Cited by: 0 articles | PMID: 39468431 | PMCID: PMC11520558
Pleiotrophin Activates cMet- and mTORC1-Dependent Protein Synthesis through PTPRZ1-The Role of α<sub>ν</sub>β<sub>3</sub> Integrin.
Int J Mol Sci, 25(19):10839, 09 Oct 2024
Cited by: 0 articles | PMID: 39409168 | PMCID: PMC11477150
Glycolysis modulation: New therapeutic strategies to improve pulmonary hypertension (Review).
Int J Mol Med, 54(6):115, 18 Oct 2024
Cited by: 0 articles | PMID: 39422043 | PMCID: PMC11518579
Review Free full text in Europe PMC
Progress in antitumor mechanisms and applications of phenformin (Review).
Oncol Rep, 52(5):151, 20 Sep 2024
Cited by: 0 articles | PMID: 39301645 | PMCID: PMC11421015
Review Free full text in Europe PMC
HOTAIR Promotes the Hyperactivation of PI3K/Akt and Wnt/β-Catenin Signaling Pathways via PTEN Hypermethylation in Cervical Cancer.
Cells, 13(17):1484, 04 Sep 2024
Cited by: 0 articles | PMID: 39273054 | PMCID: PMC11394386
Go to all (182) article citations
Data
Data behind the article
This data has been text mined from the article, or deposited into data resources.
BioStudies: supplemental material and supporting data
Similar Articles
To arrive at the top five similar articles we use a word-weighted algorithm to compare words from the Title and Abstract of each citation.
Re: mTORC1 Drives HIF-1α and VEGF-A Signalling via Multiple Mechanisms Involving 4E-BP1, S6K1 and STAT3.
J Urol, 195(2):524, 30 Oct 2015
Cited by: 2 articles | PMID: 26853037
Suppression of hypoxia-inducible factor 1α (HIF-1α) by tirapazamine is dependent on eIF2α phosphorylation rather than the mTORC1/4E-BP1 pathway.
PLoS One, 5(11):e13910, 09 Nov 2010
Cited by: 39 articles | PMID: 21085474 | PMCID: PMC2976688
Regulated in development and DNA damage 1 is necessary for hyperglycemia-induced vascular endothelial growth factor expression in the retina of diabetic rodents.
J Biol Chem, 290(6):3865-3874, 29 Dec 2014
Cited by: 37 articles | PMID: 25548280 | PMCID: PMC4319049
4E-BP1, a multifactor regulated multifunctional protein.
Cell Cycle, 15(6):781-786, 01 Jan 2016
Cited by: 161 articles | PMID: 26901143 | PMCID: PMC4845917
Review Free full text in Europe PMC
Funding
Funders who supported this work.
Cancer Research UK (1)
Grant ID: 06-914/915
Worldwide Cancer Research (1)
Identifying and characterising novel mammalian target of rapamycin (mTOR) substrates
Dr Andrew Tee, Cardiff University
Grant ID: 06-0914