Abstract
Free full text
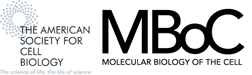
Role for Actin Filament Turnover and a Myosin II Motor in Cytoskeleton-driven Disassembly of the Epithelial Apical Junctional Complex
Abstract
Disassembly of the epithelial apical junctional complex (AJC), composed of the tight junction (TJ) and adherens junction (AJ), is important for normal tissue remodeling and pathogen-induced disruption of epithelial barriers. Using a calcium depletion model in T84 epithelial cells, we previously found that disassembly of the AJC results in endocytosis of AJ/TJ proteins. In the present study, we investigated the role of the actin cytoskeleton in disassembly and internalization of the AJC. Calcium depletion induced reorganization of apical F-actin into contractile rings. Internalized AJ/TJ proteins colocalized with these rings. Both depolymerization and stabilization of F-actin inhibited ring formation and disassembly of the AJC, suggesting a role for actin filament turnover. Actin reorganization was accompanied by activation (dephosphorylation) of cofilin-1 and its translocation to the F-actin rings. In addition, Arp3 and cortactin colocalized with these rings. F-actin reorganization and disassembly of the AJC were blocked by blebbistatin, an inhibitor of nonmuscle myosin II. Myosin IIA was expressed in T84 cells and colocalized with F-actin rings. We conclude that disassembly of the AJC in calcium-depleted cells is driven by reorganization of apical F-actin. Mechanisms of such reorganization involve cofilin-1-dependent depolymerization and Arp2/3-assisted repolymerization of actin filaments as well as myosin IIA-mediated contraction.
INTRODUCTION
Polarized epithelial cells restrict passage of solutes and macromolecules and regulate vectorial transcellular fluxes between different body compartments (Madara, 1998). Barrier function and polarity of epithelial monolayers depend on specialized intercellular structures referred to as an apical junctional complex (AJC; Yeaman et al., 1999; Matter and Balda, 2003). The two constituents of the AJC are the tight junction (TJ) and subjacent adherens junction (AJ; Farquhar and Palade, 1963). The AJ is vital for initiating and maintaining cell-cell contacts, whereas the TJ seals the intercellular space and regulates paracellular fluxes (Gumbiner, 1996; Vleminckx and Kemler, 1999; Yeaman et al., 1999; Matter and Balda, 2003). Both the TJ and AJ are composed of transmembrane proteins and cytosolic plaque proteins (Yap et al., 1997; Tsukita et al., 2001; Gonzáles-Mariscal et al., 2003). The former proteins physically associate with their counterparts at the plasma membrane of adjacent cell, whereas the latter link the TJ and AJ to the cytoskeleton and participate in intracellular signaling. Transmembrane proteins of the TJ include occludin, claudins, and junctional adhesion molecule (JAM)-1, whereas its cytoplasmic plaque consists of a number of scaffolding and signaling molecules such as the zonula occludens (ZO) protein family (Tsukita et al., 2001; Gonzáles-Mariscal et al., 2003). The major transmembrane protein constituent of AJ in epithelial cells is E-cadherin, which interacts with several catenin proteins on the cytosolic side of the plasma membrane (Yap et al., 1997).
Significant progress has been made in understanding mechanisms of assembly of the AJC during initiation of intercellular contacts and establishment of cell polarity (for review see Drubin and Nelson, 1996; Vasioukhin and Fuchs, 2001; Takai and Nakanishi, 2003). However, assembly of the AJC is only one part of its biogenesis that also involves demolition of apical junctions. For example, disassembly of the AJC occurs in embryonic morphogenesis and tissue remodeling (Decker, 1981; Caldwell et al., 1984; Miller and McClay, 1997; Jarrett et al., 2002), and during extrusion of apoptotic cells from epithelial layers (Madara, 1990). In addition, the mature AJC is likely to undergo constant reorganization manifested by endocytosis and recycling of junctional proteins (Le et al., 1999; Peifer and Yap, 2003; Matsuda et al., 2004). Such remodeling may serve to replace damaged proteins and/or a “tonic” signaling from apical junctions to the nucleus (Matter and Balda, 2003). Disassembly of the AJC is accelerated by variety of pathological stimuli including bacterial and viral proteins (Nusrat et al., 2001; Scott et al., 2002; Hopkins et al., 2003), cytokines (Han et al., 2003), growth factors (Kamei et al., 1999; Harhaj et al., 2002), and oxidative agents (Basuroy et al., 2003). However, the mechanism(s) by which epithelial AJs and TJs are disassembled remains poorly understood.
Decreases in extracellular calcium concentration to the micromolar range have been shown to induce disassembly of the epithelial AJC and have been used extensively to model formation and remodeling of apical junctions in vitro (Cereijido et al., 1978; Siliciano and Goodenough, 1988; Kartenbeck et al., 1991; Kamei et al., 1999; Ivanov et al., 2004). Using the calcium depletion model with T84 intestinal epithelial cells, we recently demonstrated that disruption of the AJC results in clathrin-mediated internalization of junctional proteins (Ivanov et al., 2004). However, intracellular events that trigger disruption and endocytosis of the AJC during calcium depletion remain poorly understood. In polarized epithelial cells, the TJs and AJs are physically linked to apical F-actin filaments that are organized in a perijunctional belt-like structure (Mooseker, 1985; Fanning, 2001). The perijunctional actin belt is critical for formation of the AJC (Madara et al., 1988; Citi et al., 1994; Stevenson and Begg, 1994; Ma et al., 2000) and regulation of paracellular permeability (reviewed in Turner, 2000; Fanning, 2001). The present study was designed to investigate the role of the actin cytoskeleton in disassembly of apical junctions in intestinal epithelial cells during calcium depletion.
MATERIALS AND METHODS
Antibodies and Other Reagents
The following primary polyclonal (pAb) and monoclonal antibodies (mAb) were used to detect TJ, AJ, and cytoskeletal proteins by immunofluorescence labeling and Western blotting: antioccludin, ZO-1 and JAM-1 pAbs (Zymed Laboratories, San Francisco, CA); anti-JAM-1 mAb (1H2A9; Liu et al., 2000); anti-E-cadherin mAb (HECD-1; Zymed); anti-β-catenin and actin pAbs (Sigma, St. Louis, MO), anti-β-catenin mAb (BD PharMingen, San Diego, CA); antimammalian nonmuscle myosin (MNMM) IIA and IIB pAbs (Covance, Berkley, CA); anti-mono- and diphosphorylated regulatory myosin light chain and antiphospho-cofilin-1 pAbs (Cell Signaling Technology, Beverly, MA); anticofilin pAb (Cytoskeleton, Denver, CO); anticortactin mAb (Upstate Biotechnology, Lake Placid, NY). Polyclonal antibodies recognizing total and phosphorylated forms of actin depolymerizing factor/cofilin-1 were generously provided by Dr. James Bamburg (Colorado State University, Fort Collins, CO). Anti-MNMM IIC and actin-related protein 3 pAbs were generously provided respectively by Dr. Robert Adelstein (National Institutes of Health, Bethesda, MD) and Dr. Matthew Welch (University of California, Berkeley, CA). Alexa-488-conjugated G-actin, rhodamine-phalloidin, as well as donkey anti-rabbit and goat anti-mouse secondary antibodies conjugated to or Alexa-488 or Alexa-568 dyes were obtained from Molecular Probes (Eugene, OR); horseradish peroxidase-conjugated goat anti-rabbit and anti-mouse secondary antibodies were obtained from Jackson ImmunoResearch Laboratories (West Grove, PA).
Cytochalasin D, latrunculin A, 2,3-butanedione monoxime (BDM), and saponin were obtained from Sigma; jasplakinolide was purchased from Calbiochem (La Jolla, CA); S(-)-blebbistatin was obtained from Toronto Research Chemicals (North York, Canada). Other reagents were of the highest analytical grade and were obtained from Sigma.
Cell Culture
T84 intestinal epithelial cells (American Type Culture Collection, Manassas, VA) were cultured in a 1:1 mixture of DMEM and Ham's F-12 medium supplemented with 10 mM HEPES, 14 mM NaHCO3, 40 μg/ml penicillin, 100 μg/ml streptomycin, 5% newborn calf serum, and adjusted to pH 7.4 (further designated as T84 complete medium). For all experiments, T84 cells were grown for 8-14 d on collagen-coated, permeable polycarbonate filters, 0.4-μm pore size (Costar, Cambridge, MA). Filters with a surface area of 0.33 and 5 cm2 were used for immunocytochemical and biochemical experiments, respectively.
Calcium Depletion and Pharmacological Modulation of Apical Junction Disassembly
To deplete extracellular Ca2+, confluent T84 monolayers were washed twice with calcium-free Eagle's minimum essential medium for suspension culture (Sigma) supplemented with 2 mM EGTA, 10 mM HEPES, 14 mM NaHCO3, and 5% dialyzed newborn calf serum (designated here as S-MEM) and incubated in S-MEM for indicated times at 37°C. For pharmacological modulation of cytoskeleton, T84 cells were preincubated for 60 min with inhibitors in complete T84 medium followed by 60-min incubation in S-MEM containing the same concentration of inhibitor. Stock solutions of water-insoluble inhibitors were prepared in DMSO and diluted in cell culture media immediately before each experiment. The final concentration of DMSO was 0.1%; the same concentration of the vehicle was included in appropriate controls.
Immunofluorescence Labeling
Calcium-depleted T84 monolayers were rinsed twice with ice-cold calcium- and magnesium-free HBSS containing 10 mM HEPES (HBSS-), whereas control monolayers were rinsed with HEPES-buffered HBSS containing calcium and magnesium (HBSS+). Cells were fixed/permeabilized in absolute methanol for 20 min at -20°C followed by blocking in HBSS+ containing 1% bovine serum albumin (blocking buffer) for 60 min at room temperature and incubation for 60 min with primary antibodies in blocking buffer. Cell monolayers were then washed, incubated for 60 min with Alexa dyeconjugated secondary antibodies followed by rinsing and mounting on slides with ProLong Antifade medium (Molecular Probes). For double labeling of junctional proteins and myosin II with F-actin, monolayers were fixed in 3.7% paraformaldehyde (PFA), permeabilized with 0.5% Triton X-100 (TX-100), and sequentially stained with primary and green Alexa dye-conjugated secondary antibodies, whereas F-actin was labeled with rhodamine-phalloidin. Stained monolayers were examined using a Zeiss LSM510 laser scanning confocal microscope (Zeiss Microimaging, Thornwood, NY) coupled to a Zeiss 100M axiovert and 63× or 100× Pan-Apochromat oil lenses. Fluorescent dyes were imaged sequentially in frame-interlace mode to eliminate cross-talk between channels. Images shown are representative of at least three experiments, with multiple images taken per slide.
Transmission Electron Microscopy
Confluent T84 monolayers were fixed in 4% buffered glutaraldehyde and cut into 1-μm strips using a microtome. The strips were postfixed in 1% osmium tetraoxide, sequentially dehydrated through graded alcohols and propylene oxide, and then infiltrated with Embed-812 (Electron Microscopy Sciences, Ft. Washington, PA). Strips were embedded for cross-section orientation. Semithin (0.5 mm) sections were cut, stained with toluidine blue, and examined for adequacy. Ultrathin section (900 Å) were cut with a diamond knife, stained with uranyl acetate and lead citrate, and examined with a Philips EM201 electron microscope (Philips Electronics, Mahwah, NJ).
Immunoblotting
Cells were homogenized in a lysis buffer (20 mM Tris, 50 mM NaCl, 2 mM EDTA, 2 mM EGTA, 1% sodium deoxycholate, 1% TX-100, and 0.1% SDS, pH 7.4), containing a proteinase inhibitor cocktail (1:100, Sigma) and phosphatase inhibitor cocktails 1 and 2 (both at 1:200, Sigma). Lysates were then cleared by centrifugation (20 min at 14,000 × g) and immediately boiled in SDS sample buffer. PAGE and immunoblotting were conducted by standard methods with 10-20 μg protein per lane. The results shown are representative immunoblots of at least three independent experiments. Quantification of protein expression was performed by densitometric analysis of Western blot images on the UN-SCAN-IT automated digitizing system (Silk Scientific, Orem, UT).
Fractionation of G- and F-actin and Incorporation of Exogenous G-actin
Quantification of G- and F-actin was performed by TX-100 fractionation of intracellular actin as previously described (Cramer et al., 2002). Briefly, control and calcium-depleted monolayers were washed with HBSS+ and HBSS-, respectively, and G-actin was extracted by gentle shaking for 5 min at room temperature in HBSS+ containing 1% TX-100, the proteinase inhibitor cocktail, and 1 μg/ml phalloidin to prevent filament disassembly. This extraction method has been shown to remove ~95% of monomeric actin (Cramer et al., 2002). The TX-100-soluble G-actin fraction was mixed with an equal volume of SDS sample buffer and boiled. Filters were then briefly washed with HBSS+ and the TX-100-insoluble F-actin fraction collected by scraping cells in 2 volumes of SDS sample buffer, and boiled. The amount of actin in each fraction was determined by gel electrophoresis and Western blotting as described above. A similar procedure was also used to determine association of junctional proteins and myosin II with F-actin, except that the TX-100 extraction was performed for 15 min on ice.
Introduction of exogenous G-actin into permeabilized cells was performed as previously described (Symons and Mitchison, 1991). Briefly, T84 monolayers were calcium-depleted for 15 min and washed twice in a rinsing buffer (20 mM HEPES, 138 mM KCl, 4 mM MgCl2, 3 mM EGTA, 1 mM ATP, pH 7.4). Cells were then incubated for 5 min at room temperature in a permeabilizing buffer (0.2 mg/ml saponin in the rising buffer) containing 1 μM of fluorescently labeled G-actin. Thereafter, cells were washed with HBSS-, fixed with PFA, permeabilized with 0.5% TX-100, and counterstained for F-actin with rhodamine-phalloidin.
Transepithelial Resistance Measurement
Effect of calcium depletion on transepithelial electrical resistance (TEER) was measured using an EVOMX voltohmmeter (World Precision Instruments, Sarasota, FL). The resistance of cell-free collagen-coated filters was subtracted from each experimental point.
Statistics
Numerical values from individual experiments were pooled and expressed as mean ± SE of the mean (SE) throughout. Values obtained for control and calcium-depleted groups were compared by two-tailed Student's t tests, with statistical significance assumed at p < 0.05.
RESULTS
Disassembly of the AJC in Calcium-depleted Cells Depends on Integrity of Actin Filaments and Is Associated with Formation of Contractile Apical F-actin Rings
To investigate whether disassembly of the AJC and internalization of junctional proteins in calcium-depleted epithelial cells depends on integrity of the actin cytoskeleton, we treated T84 cells with cytochalasin D or latrunculin A, low-molecular-weight agents that depolymerize F-actin via different mechanisms. Cytochalasin D prevents polymerization of actin filaments by capping their fast growing end (Urbanik and Ware, 1989), whereas latrunculin A depolymerizes the filaments by sequestering monomeric actin (Morton et al., 2000). In agreement with our previous study (Ivanov et al., 2004), depletion of extracellular calcium for 60 min disrupted the AJC in vehicle-treated T84 cells and caused translocation of AJ (E-cadherin) and TJ (occludin and JAM-1) proteins from intercellular contacts into centrally located ring-like structures (Figure 1). Pretreatment of T84 monolayers with cytochalasin D (10 μM) or latrunculin A (5 μM) significantly attenuated disruption of the AJC and translocation of junctional proteins in calcium-depleted cells (Figure 1).
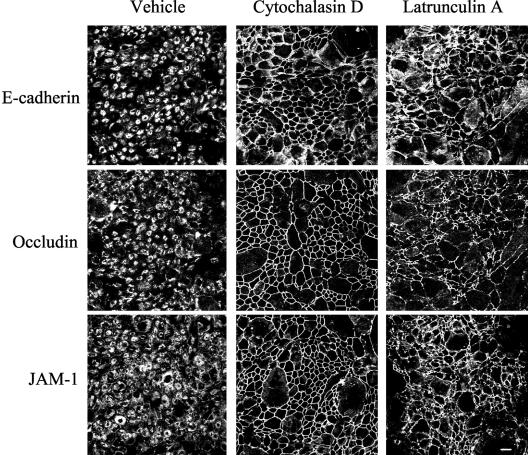
Depolymerization of F-actin prevents disassembly of the AJC in calcium-depleted epithelial cells. Confluent T84 cells were preincubated for 60 min in either cytochalasin D (10 μM) or latrunculin A (5 μM) or vehicle followed by 60 min incubation in S-MEM containing the same concentrations of drugs. Localization of E-cadherin, occludin, and JAM-1 was determined by immunofluorescence labeling and confocal microscopy. As can be seen, in vehicle treated cells calcium depletion leads to translocation of junctional proteins from areas of cell-cell contact into centrally located ring-like structures. The F-actin-depolymerizing agents prevent disassembly of the AJC and translocation of junctional proteins. Bar, 10 μm.
To analyze the effect of calcium depletion on organization of junction-associated actin filaments, we visualized F-actin using a fluorescent derivative of phalloidin. In T84 cells cultivated with a normal concentration of calcium, apical F-actin appeared as a thick belt at the level of the AJC as well as an array of dots and short filaments at the base of brush-border microvilli (Figure 2). Calcium depletion resulted in dramatic reorganization of apical F-actin into centrally located rings with radiating F-actin cables (Figure 2, arrows). During the course of calcium depletion, the actin rings were observed to decrease in diameter, which indicates their contraction (compare images corresponding to 10- and 30-min time points in Figure 2). After 120 min of calcium depletion, the actin rings began to disintegrate into small vesicle-like structures (Figure 2, dashed arrows).
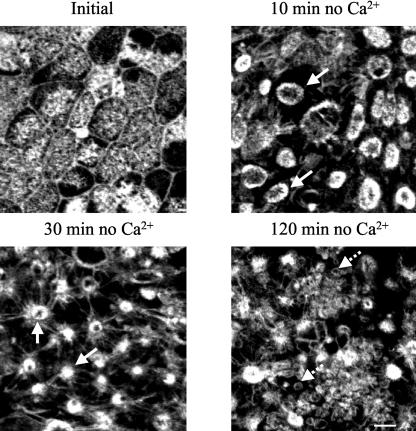
Calcium depletion induces rapid reorganization of apical F-actin cytoskeleton. Confluent T84 monolayers were incubated in S-MEM for indicated times and organization of F-actin at the level of the AJC was visualized using fluorescently labeled phalloidin and confocal microscopy. In control T84 cells, apical actin is organized in short brush-border filaments surrounded by the perijunctional F-actin belt. Depletion of extracellular calcium leads to reorganization of apical F-actin into ring-like structures (arrows) and radiating F-actin cables. The rings progressively contract and eventually collapse into small vesicles in the late stages of calcium depletion (dashed arrows). Bar, 5 μm.
Evidence suggests that, in polarized epithelial cells cultured in normal concentration of calcium, the perijunctional actin belt is physically attached to the plasma membrane (Mooseker, 1985; Volberg et al., 1986; Fanning, 2001). If such attachment is preserved in calcium-depleted cells (Castillo et al., 1998), contraction of apical F-actin ring would result in retraction of plasma membranes of adjacent cells and provide force required for disruption of their intercellular junctions. To prove this, we analyzed the morphology of calcium-depleted T84 cells using transmission electron microscopy. Electron micrographs of T84 monolayers cultured in normal concentration of calcium revealed columnar, fully differentiated cells that contain well developed apical junctions (Figure 3, dotted arrow). At an early time point (30 min) of calcium depletion, a centripetal retraction of plasma membrane at the cell apex, effectively separating the brush-border from cell body was observed (Figure 3, arrow). Such retraction created apical and subapical filopodia-like structures that are likely to represent retraction fibers through which neighboring cells remain attached to each other (Figure 3, asterisk). We observed this membrane retraction to be limited to the cell apex and not accompanied by gross changes in cell shape at early times of calcium depletion (Figure 3). We did not investigate the fate of apical blebs; however, it is possible that they may de-attach from the cell, as has been observed in energy-depleted hepatocytes (Zahrebelski et al., 1995) and renal epithelial cells (Chen and Wagner, 2001), or fuse with the cell body after disintegration of the F-actin ring. On the basis of these ultrastructural findings, we suggest that the F-actin ring observed by fluorescent labeling and confocal microscopy is located in and beneath the furrow separating the apical brush-border, whereas radiating F-actin cables support retraction fibers.
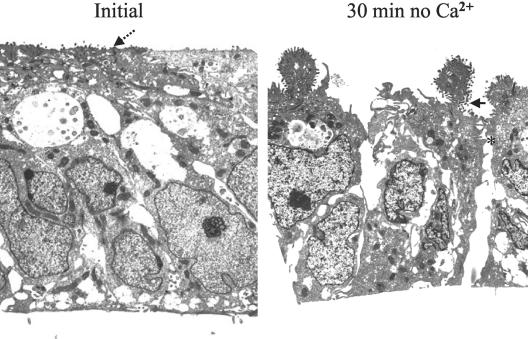
Calcium depletion induces apical contraction in intestinal epithelial cells. Morphology of normal T84 cell monolayer and cells depleted in calcium for 30 min was evaluated by transmission electron microscopy. In normal monolayers, cells are attached to each other by apical junctions (dotted arrow). At an early stage of calcium depletion, a centripetal retraction of the plasma membrane and separation of brush-border is observed (arrow), whereas cells remain in contact with each other by retraction fibers (asterisk).
To link reorganization of the perijunctional actin belt and disassembly of the AJC in calcium-depleted cells, we double-labeled F-actin with different AJ/TJ proteins. At early times (30 min) of calcium depletion, the ring-like structures containing E-cadherin and occludin clearly colocalized with F-actin rings (Figure 4A, arrows). Similar colocalization with F-actin was also found for ZO-1, JAM-1, and β-catenin (our unpublished results). The ring-like structures containing E-cadherin and occludin were resistant to extraction with the TX-100/phalloidin buffer, indicating physical association of these junctional proteins with F-actin (our unpublished results). At later times (60-120 min) of calcium depletion, junction proteins appeared underneath the actin rings and became more diffusely distributed in a subapical compartment (our unpublished results; see also Ivanov et al., 2004).
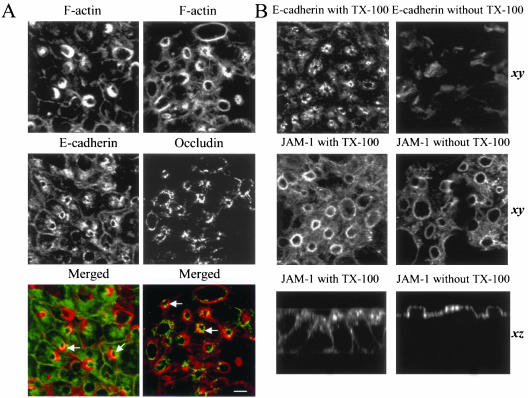
Translocated junctional proteins colocalize with F-actin rings that are assembled both intracellularly and on the plasma membrane. (A) T84 cells were incubated for 30 min in calcium-free media and double-labeled for E-cadherin and occludin (green) with F-actin (red). The ring-like structures containing E-cadherin and occludin (arrows) clearly colocalize with F-actin rings. (B) Calcium-depleted (30 min) T84 cells were fixed with PFA and labeled with antibodies recognizing extracellular domains of E-cadherin and JAM-1 with and without TX-100 permeabilization. E-cadherin containing ring-like structures are visible only in permeabilized cells, suggesting their cytosolic localization. Ring-like structures containing JAM-1 are observed in permeabilized and nonpermeabilized cells, indicating that they are partially assembled on the plasma membrane. Reconstructed xz images show broad apical staining of JAM-1 in permeabilized cells and narrow surface labeling in nonpermeabilized cells, suggesting that the ring-like structures contain both surface-exposed and internalized JAM-1. Bar, 5 μm.
Several previous studies including ours showed internalization of AJ/TJ proteins after calcium depletion (Kartenbeck et al., 1991; Kamei et al., 1999; Le et al., 1999; Ivanov et al., 2004), whereas others reported distribution of the AJC remnants within plasma membrane (Pitelka et al., 1983; Troyanovsky et al., 1999; Fukuhara et al., 2002). To test whether the ring-like structures containing AJ/TJ proteins are initially assembled on the cell surface or intracellularly, we labeled PFA-fixed TX-100-permeabilized and nonpermeabilized T84 cells with antibodies recognizing extracellular domains of E-cadherin and JAM-1. E-cadherin-containing rings were identified only in permeabilized calcium-depleted cells (Figure 4B), indicating their intracellular localization. In contrast, the JAM-1-containing ring-like structures were visible in both nonpermeabilized and permeabilized cells (Figure 4B), suggesting that the rings are exposed to the cell surface. In support of this, reconstructed xz images demonstrated a narrow band of JAM-1 labeling at the apical plasma membrane of nonpermeabilized cells (Figure 4B). However, xz image of permeabilized cells revealed much broader apical JAM-1 labeling (Figure 4B), likely representing both plasma membrane and submembranous, internalized pools of the protein. These data suggest that during calcium depletion AJ proteins undergo direct F-actin-dependent internalization into a subapical compartment. TJ proteins initially reorganize into actin-associated ring-like structure at the plasma membrane after which they are at least partially internalized (Figure 4; see also Ivanov et al., 2004).
Formation of Contractile F-actin Rings Involves Turnover of Actin Filaments Associated with Activation of Cofilin-1/ADF and Recruitment of the Arp2/3 Complex
Given the observation that formation of contractile F-actin rings appears to be a driving force for disassembly of the AJC in calcium-depleted epithelial cells, we next investigated mechanisms involved in formation and contraction of these rings. Two potential mechanisms were investigated: a turnover (depolymerization/repolymerization) of cortical actin filaments and a myosin-dependent movement of stable actin filaments.
We first investigated whether depolymerization of pre-existing actin filaments is required for creation of F-actin rings in calcium-depleted cells. Actin depolymerization was inhibited using jasplakinolide, a cell-permeable agent that promotes polymerization and stabilizes actin filaments (Bubb et al., 1994, 2000). Because jasplakinolide has been shown to block phalloidin binding to F-actin (Bubb et al., 1994, 2000), we visualized total actin with polyclonal antibody. In calcium-depleted cells, this antibody detected the F-actin rings similar to those visualized by rhodamine-phalloidin (Figure 5). Incubation of T84 cells with jasplakinolide (2 μM) drastically attenuated ring formation. Furthermore, stabilization of F-actin with jasplakinolide prevented disassembly of the AJC and translocation of E-cadherin and occludin from areas of cell-cell contacts (Figure 5), thus suggesting a critical role for F-actin depolymerization in these processes.
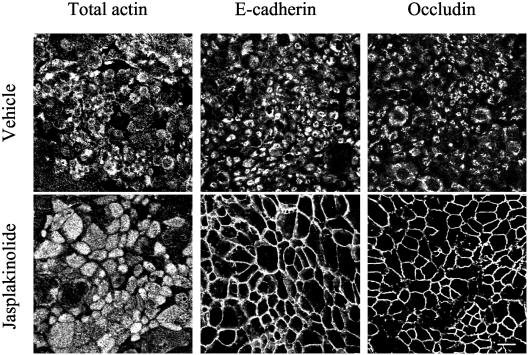
Stabilization of actin filaments attenuates F-actin reorganization and disassembly of the AJC in calcium-depleted cells. T84 cells were preincubated for 60 min with jasplakinolide (2 μM) or vehicle followed by 60 min incubation in S-MEM containing the same concentrations of jasplakinolide. Localization of total actin, E-cadherin, and occludin was determined by immunolabeling and confocal microscopy. Jasplakinolide, which prevents depolymerization of actin filaments, substantially attenuates formation of contractile actin rings and disassembly of AJs and TJs in calcium-depleted cells. Bar, 10 μm.
We next analyzed whether calcium depletion induces activation of members of the actin depolymerizing factor (ADF)/cofilin protein family. These experiments were designed because ADF/cofilin proteins are known to greatly accelerate depolymerization of filamentous actin in living cells (Lappalainen and Drubin, 1997). It is generally believed that ADF/cofilin proteins possess much stronger F-actin-depolymerizing activity when they exist in the nonphosphorylated state (Moon and Drubin, 1995; Bamburg, 1999; Ono, 2003). Therefore, we used commercially available antibodies recognizing either total or phosphorylated (Ser3) cofilin-1 to analyze the phosphorylation status and thus activity of this protein. As shown in Figure 6, calcium depletion caused a progressive decrease in the amount of phosphorylated cofilin-1 in T84 total cell lysates without a significant effect on the total amounts of cofilin-1. Densitometry analysis of Western blots revealed that after 60 min of calcium depletion, the average amounts of phosphorylated and total cofilin-1 were, respectively, 11 and 76% of control levels (Figure 6B). Similar results (our unpublished data) were obtained using a different set of antibodies recognizing either total cofilin-1 and its homologue ADF (Morgan et al., 1993) or identically phosphorylated forms of these two proteins (Meberg et al., 1998). We also used the latter antibodies to analyze the effect of calcium depletion on intracellular localization of cofilin-1/ADF. Double labeling showed colocalization of total cofilin-1/ADF in areas of F-actin rings in calcium-depleted cells (Figure 6B, arrows). In contrast, no substantial colocalization of F-actin and phosphorylated cofilin-1/ADF was found, indicating that the nonphosphorylated, active forms of these proteins colocalize with F-actin rings. Taken together, these data suggest that calcium depletion is accompanied by activation (dephosphorylation) of cofilin-1 and its translocation to F-actin rings.
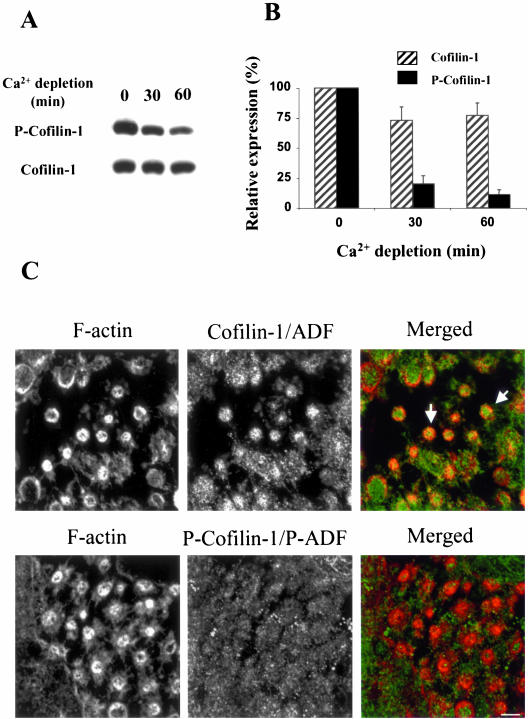
Calcium depletion is accompanied by activation of cofilin-1 and its accumulation in contractile F-actin rings. T84 cells were incubated in S-MEM for indicated times and amount of total and phosphorylated (P) cofilin-1 in cell lysates was determined by Western blotting. Representative blot (A) and quantification results from three independent experiments (B) shows that the amount of phosphorylated (inactive) but not total cofilin-1 drastically decreases during calcium depletion. (C) Calcium-depleted (30 min) cells were double-labeled for total cofilin-1/ADF and phosphorylated cofilin-1/ADF (green) with F-actin (red). Total cofilin-1/ADF colocalizes with F-actin rings (arrows); however, no such colocalization is seen for phosphorylated (inactive) cofilin-1/ADF. Bar, 5 μm.
Next we asked whether actin depolymerization is involved in the initial step of F-actin ring formation or whether biogenesis of such rings requires continuous depolymerization and de novo polymerization of actin. If the latter mechanism is valid, then sequestration of monomeric actin would be expected to induce disassembly of F-actin rings. To test this, we allowed the rings to develop during first 30 min of calcium depletion and then treated cells for another 30 min with either a high concentration of the G-actin-binding drug latrunculin A (50 μM) or vehicle. Figure 7A demonstrates that treatment with vehicle did not affect integrity of the F-actin rings, whereas sequestration of monomeric actin by latrunculin A caused rapid (within 30 min) ring disassembly. As a complementary approach, we investigated incorporation of exogenous G-actin into cytoskeletal structures of gently permeabilized, calcium-depleted T84 cells. We preincubated cells without calcium for 15 min with subsequent introduction of the fluorescently labeled G-actin in cell-permeabilizing buffer. Figure 7B shows that within 5 min of such introduction, a substantial amount of exogenous G-actin is incorporated into F-actin rings, especially at the ring edge (arrows). Interestingly, the rapid turnover of F-actin rings in calcium-depleted cells was not accompanied by significant changes in a balance between G and F-actin. Thus, the calculated G/F actin ratio was 0.96 ± 0.16 and 1.08 ± 0.3 (n = 3; p > 0.05) in T84 cells cultured in normal concentration of calcium and in calcium-free medium for 60 min, respectively.
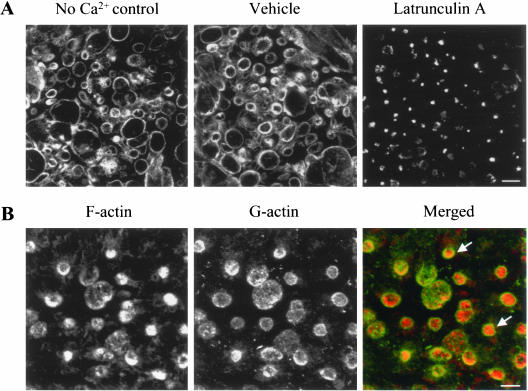
G-actin is essential for stability of the contractile F-actin rings, and it is incorporated into rings during calcium depletion. (A). T84 cells were preincubated in S-MEM for 30 min followed by another 30 min incubation in S-MEM containing either G-actin-binding agent, latrunculin A (50 μM), or vehicle. Sequestration of G-actin with latrunculin A, but not vehicle treatment results in rapid disassembly of preformed F-actin rings. Bar, 10 μm. (B). Fluorescently labeled exogenous G-actin (green) was introduced for 5 min into saponin-permeabilized calcium-depleted T84 cells, and its intracellular localization together with topography of F-actin (red) was determined by confocal microscopy. As can be seen, exogenous G-actin is incorporated into F-actin rings in calcium-depleted cells (arrows). Bar, 5 μm.
Because nucleation of new actin filaments is regulated by the actin related protein (Arp) 2/3 complex (Suetsugu et al., 2002; Welch and Mullins, 2002), one could expect accumulation of the Arp2/3 complex in contractile F-actin rings if de novo actin polymerization occurs during their biogenesis. Indeed, double-labeling experiments revealed substantial colocalization of Arp3 protein with F-actin rings in calcium-depleted cells (Figure 8A, arrows). Furthermore, these rings were also enriched in cortactin (Figure 8A, arrows), a protein that recruits the Arp2/3 complex to actin filaments (Weed et al., 2000; Urino et al., 2001). Another argument supporting the role of Arp2/3-dependent actin polymerization in the formation of contractile F-actin rings was obtained by using 2,3-butanedione monoxime (BDM), a low-molecular-weight agent that has been reported to prevent Arp2/3 binding to F-actin (Yarrow et al., 2003). As shown in Figure 8B, BDM treatment (30 mM) attenuated the formation of F-actin rings and translocation of E-cadherin and occludin from intercellular junctions after 30 min of calcium depletion (compare images on Figure 8B to the time-matched controls presented in Figures Figures22 and and4A).4A). The Arp2/3-related data reinforces the idea that de novo actin polymerization is important for biogenesis of contractile F-actin rings and disassembly of the AJC in calcium-depleted epithelial cells.
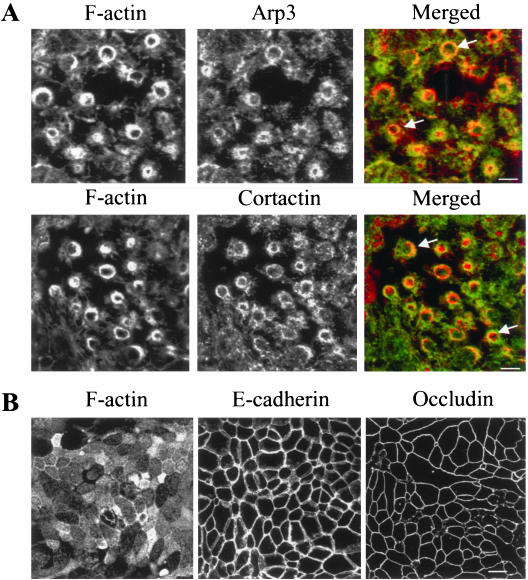
Arp2/3 complex is involved in formation of F-actin rings and disassembly of the AJC. (A). T84 cells were depleted in calcium for 30 min and double-labeled for Arp3 and cortactin (green) with F-actin (red). As can be seen, both components of the active Arp2/3 complex colocalize with F-actin rings (arrows). Bar, 5 μm. (B). T84 cells were preincubated for 60 min with BDM (30 mM) followed by 30-min incubation in S-MEM containing the same concentrations of BDM. Localization of F-actin, E-cadherin, and occludin was determined by fluorescence labeling and confocal microscopy. BDM, which dislocates the Arp2/3 complex from actin filaments, substantially attenuates formation of contractile F-actin rings and disassembly of AJs and TJs in calcium-depleted cells (compare with time-matched images of untreated calcium-depleted cells on Figures Figures22 and and4A).4A). Bar, 10 μm.
Activity of Myosin IIA Is Required for Biogenesis of Contractile F-actin Rings
Besides directed actin polymerization, it can be envisioned that simple sliding of neighboring actin filaments against each other may provide force for contraction of F-actin rings (Mooseker, 1985; Spudich, 2001). In human intestinal epithelial cells, such movement is assisted by mammalian nonmuscle myosin (MNMM) II (Mooseker, 1985). We sought to investigate whether MNMM II plays a role in contraction of F-actin rings and disassembly of the AJC during calcium depletion. As shown in Figure 9A, treatment of T84 cells with selective MNMM II inhibitor blebbistatin (50 μM) completely prevented reorganization of apical F-actin and translocation of E-cadherin and occludin from intercellular junctions. Blebbistatin treatment also affected the barrier properties of T84 monolayers (Figure 9B). Indeed at normal calcium concentration, inhibition of MNMM II slightly decreased TEER. However, after 60 min of calcium depletion, blebbistatin treatment significantly attenuated the drop in electrical resistance (Figure 9B). In calcium depleted, blebbistatin treated monolayers, the TEER remained in the range of 400-450 Ω cm2, which is characteristic of functional epithelial apical junctions.
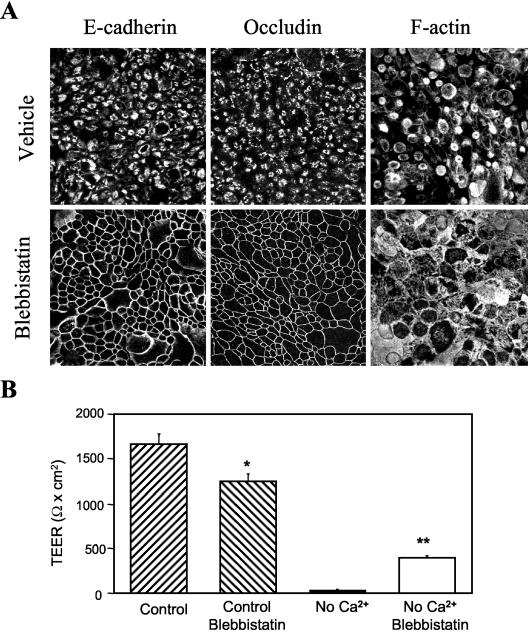
Inhibition of mammalian nonmuscle myosin (MNMM) II prevents F-actin reorganization, disassembly of the AJC, and barrier dysfunction in calcium-depleted cells. (A). T84 cells were preincubated for 60 min with blebbistatin (50 μM) or vehicle followed by 60-min incubation in S-MEM containing the same concentrations of the inhibitor. Localization of F-actin, E-cadherin, and occludin was determined by fluorescence labeling and confocal microscopy. Blebbistatin, which selectively inhibits activity of MNMM II, completely blocks formation of contractile F-actin rings and disassembly of AJs and TJs in calcium-depleted cells. (B). T84 cells were either incubated for 120 min in T84 complete medium containing blebbistatin (50 μM) or pretreated with blebbistatin for 60 min followed by 60 min of calcium depletion in the presence of the inhibitor. Appropriate controls were incubated with vehicle, and TEER in all groups was measured. Blebbistatin slightly decreases resistance in T84 monolayers at normal concentration of calcium, but significantly attenuates the drop in TEER during calcium depletion. Data are presented as mean ± SE (n = 3); *p < 0.05; **p < 0.01 comparing to vehicle-treated controls.
Three isoforms of MNMM II referred to as IIA, IIB, and IIC have been characterized to date (Murakami et al., 1991; Phillips et al., 1995; Golomb et al., 2004). The isoforms differ in their heavy-chain structure and may have distinct biological functions (Maupin et al., 1994; Kolega, 2003; Togo and Steinhardt, 2004). Although myosin II has been shown to be abundant in intestinal epithelial cells (Mooseker, 1985; Fanning, 2001), which isoforms are expressed is unknown. Using antibodies that specifically recognize different MNMM II heavy chains, we analyzed expression and localization of known myosin II isoforms in T84 cells. Figure 10A shows that MNMM IIA is strongly expressed, whereas expression of the IIB isoform is much weaker and IIC is virtually undetectable by Western blot. Immunolabeling and confocal microscopy showed that in polarized T84 cells cultured in a normal calcium concentration, MNMM IIA is enriched in the cell apex, presumably in brush-border microvilli (Figure 10B). In cells incubated for 30 min without calcium, MNMM IIA was found to strongly colocalize with contractile F-actin rings and to accumulate along F-actin cables penetrating retraction fibers (Figure 10B). These data are in agreement with previously observed colocalization of an unspecified myosin II isoform within F-actin rings in calcium-depleted MDCK and Caco-2 cells (Castillo et al., 1998; Ma et al., 2000b). Fractionation with TX-100/phalloidin-containing buffer demonstrated that in control cells the majority of MNMM IIA is TX-100-soluble (Figure 10C) and therefore weakly associated with actin filaments. This is not a surprising finding for a motor protein with a fast rate of association with/dissociation from the cytoskeleton (Spudich, 2001). Compared with the control, calcium-depleted cells had greater amounts of TX-100-insoluble MNMM IIA (Figure 10C), thus indicating increased physical association of myosin II with F-actin.
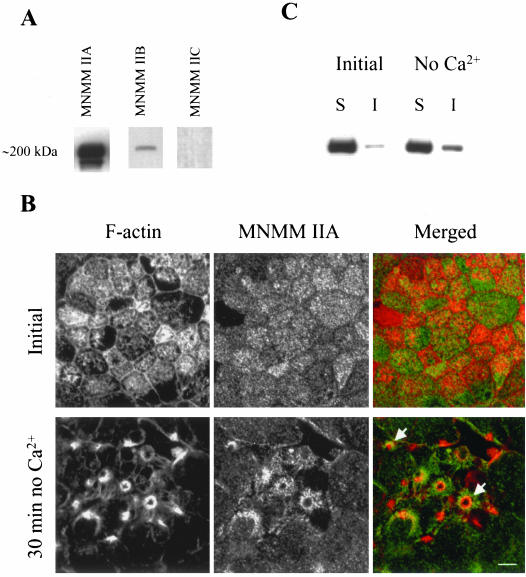
Mammalian nonmuscle myosin IIA isoform is predominantly expressed in T84 epithelial cells and colocalizes with F-actin rings during calcium depletion. (A) Expression of different MNMM II isoforms in T84 total cell lysates was determined by Western blotting. The MNMM IIA is highly expressed in T84 cells, whereas expression of the IIB isoform is weak and MNMM IIC is undetectable. (B). Control T84 cells and calcium-depleted (30 min) cells were double-labeled for MNMM IIA (green) with F-actin (red). As can be seen, in normal cells MNMM IIA is enriched in apical brush-border, whereas in calcium-depleted cells MNMM IIA colocalizes with F-actin rings (arrows). (C). Control T84 cells and cells incubated in S-MEM for 60 min were fractionated using a TX-100/phalloidin-containing buffer as described in MATERIAL AND METHODS, and distribution of MNMM IIA between TX-100-soluble (S) and -insoluble (I) fractions was determined. Calcium depletion increases amount of TX-100-insoluble (F-actin-associated) fraction of MNMM IIA. Bar, 5 μm.
Colocalization of the MNMM IIA heavy chain with F-actin does not necessarily reflect the presence of a fully functional motor protein. Activity of myosin II requires association of its heavy chains with phosphorylated regulatory myosin light chains (RMLC; reviewed in Tan et al., 1992; Bresnick, 1999). To determine whether actin rings contain active MNMM IIA, we performed double labeling of F-actin with two antibodies specifically recognizing mono-phosphorylated (Ser 19) and di-phosphorylated (Ser19/Thr18) RMLCs. After 30 min of calcium depletion, we found significant colocalization of phosphorylated RMLCs with contractile F-actin rings (Figure 11 arrows). Taken together our pharmacological and morphological data support a role of MNMM IIA in the formation of apical F-actin rings and disassembly of the AJC in calcium-depleted T84 cells.
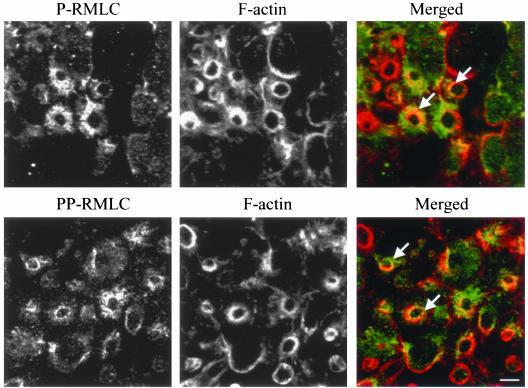
Mono- and di-phosphorylated regulatory myosin light chain colocalize with contractile F-actin rings in calcium-depleted cells. T84 cells were calcium-depleted for 30 min and double-labeled for mono- and diphosphorylated regulatory myosin light chain (P-RMLC and PP-RMLC, respectively; labeled in green) with F-actin (red). As can be seen, both P-RLMC and PP-RLMC colocalize with F-actin rings (arrows). Bar, 5 μm.
DISCUSSION
Formation of Contractile F-actin Rings Triggers Disruption of Apical Junctions in Calcium-depleted Cells
Depletion of extracellular calcium in differentiated epithelial monolayers causes orchestrated translocation of major AJ and TJ proteins from areas of cell-cell contacts and their subsequent endocytosis (Siliciano and Goodenough, 1988; Kartenbeck et al., 1991; Kamei et al., 1999; Ivanov et al., 2004). Rapid coordinated movement of large multiprotein complexes is likely to be mediated by cytoskeletal reorganization. Because in epithelial cells the apical perijunctional F-actin belt affiliates with the AJC and controls assembly (Vasioukhin and Fuchs, 2001) and barrier properties of apical junctions (Madara et al., 1988; Citi et al., 1994; Stevenson and Begg, 1994; Ma et al., 2000a; Turner et al., 2000), we sought to investigate the role of actin cytoskeleton in disassembly of the AJC after removal of extracellular calcium.
Depolymerization of F-actin filaments with either cytochalasin D or latrunculin A before calcium removal prevented translocation of AJ/TJ proteins in calcium-depleted T84 cells (Figure 1). These data and similar results reported in Madin-Darby canine kidney (MDCK) epithelial cells (Citi et al., 1994; Stevenson and Begg, 1994) substantiate the role for actin filaments in AJC disassembly. Furthermore, morphological analysis of actin filaments revealed rapid formation of apical F-actin rings in calcium-depleted cells (Figure 2). We observed that contraction of these rings resulted in deep invaginations of the plasma membrane at the level of the AJC and “pinched-off” areas in the brush-border (Figure 3). Such F-actin-driven membrane retraction is likely to be uneven, creating multiple retraction fibers at the cell apex (Figure 3). These fibers contain F-actin cables (Figures (Figures22 and and4A)4A) and remnants of TJs (Figure 4) and are responsible for residual contacts between adjacent cells at early times of calcium depletion (Figure 3).
We believe that actin-driven retraction of the plasma membrane provides mechanical forces that disassemble the AJC. As a result, AJ and TJ proteins translocate to a deepening furrow and assemble into novel ring-like structures associated with contractile F-actin rings (Figure 4A). It appears that translocation routes for transmembrane AJ and TJ proteins are different. In particular, E-cadherin becomes rapidly internalized after disruption of intercellular contacts and accumulates in a subapical cytosolic compartment (Figure 4B). In contrast, TJ remnants containing JAM-1 (and probably other TJ proteins) become initially reorganized at the cell surface (Figure 4B) and internalized at later times of calcium depletion. These data support our previous findings that internalized AJ and TJ proteins occupy adjacent but distinct parts of a cytosolic storage compartment (Ivanov et al., 2004). Interestingly, reorganization of apical F-actin and disassembly of the AJC in T84 cells are completely reversible after restoration of normal calcium concentration in the cell culture medium (our unpublished results).
Role for Actin Filament Turnover in Biogenesis of F-actin Rings
Contractile F-actin rings play critical roles in variety of biological processes including cell division (Field et al., 1999; Noguchi et al., 2001), wound closure (Bement et al., 1993, 1999; Mandato and Bement, 2001; Florian et al., 2002), endocytosis (Araki et al., 2002; Sokac et al., 2003), and extrusion of apoptotic cells from epithelial monolayers (Rosenblatt et al., 2001). Two different mechanisms account for generation of force required for ring contraction. One mechanism is due to dynamic depolymerization/repolymerization of actin filaments (Mandato and Bement, 2001; Henson et al., 2002; Pelham and Chang, 2002; Sokac et al., 2003). Another requires production of force by the motor protein, myosin II, (Bement et al., 1993, 1999; Mandato and Bement, 2001; Araki et al., 2002; Florian et al., 2002). Because both mechanisms have been implicated in the biogenesis of F-actin rings during cytokinesis and wound healing (Mandato and Bement, 2001; Noguchi et al., 2001), we analyzed whether they are involved in reorganization of apical F-actin in calcium-depleted epithelial cells.
Actin creates motile force via directed filament growth (Pantaloni et al., 2001; Pollard and Borisy, 2003). In this process, known as treadmilling, monomeric units are added to so-called “plus or barbed” ends of filaments and are removed from the opposite, “minus or pointed” ends (Pantaloni et al., 2001; Pollard and Borisy, 2003), thus rationalizing requirement of both polymerization and depolymerization for filament movement. In the present study, we have demonstrated that actin depolymerization is important for formation of contractile rings, because stabilization of F-actin with jasplakinolide drastically attenuated this process as well as disassembly of the AJC during calcium depletion (Figure 5). In a search for mechanisms involved in such actin depolymerization, we analyzed functional state of members of the ADF/cofilin family that accelerate disassembly of actin filaments in vivo (Moon and Drubin, 1995; Bamburg, 1999; Ono, 2003). Two members of this protein family, ADF and cofilin-1 are expressed in epithelial cells where they exist in nonphosphorylated and phosphorylated states (Bamburg, 1999). The nonphosphorylated state has much higher actin-depolymerizing activity (Bamburg, 1999; Ono, 2003). We found rapid dephosphorylation of cofilin-1 in calcium-depleted T84 cells (Figure 6, A and B), indicating activation of this protein. Furthermore, active, nonphosphorylated cofilin-1/ADF accumulated in contractile F-actin rings (Figure 6C). Similar decrease in phosphorylation and translocation of cofilin/ADF to areas of apical F-actin disassembly has been found in energy-depleted renal epithelial cells (Ashworth et al., 2001, 2003), where functional importance of these events was confirmed by expression of constitutive active and inactive forms of cofilin/ADF (Ashworth et al., 2003). We believe that activation of cofilin-1/ADF in calcium-depleted cells also has functional consequences by mediating disassembly of the apical F-actin.
In agreement with the idea that rapid turnover of actin filaments is involved in biogenesis of contractile F-actin rings in calcium-depleted cells, we demonstrated that de novo actin polymerization is essential for the rings formation and stability. Selective sequestration of G-actin with latrunculin A prevented rings formation (Figure 1) and resulted in rapid disassembly of intact ones (Figure 7A), indicating their dependence on a constant supply of monomeric actin. Furthermore, exogenous G-actin introduced into permeabilized calcium-depleted cells was rapidly incorporated into contractile F-actin rings (Figure 7B). Another group of evidence suggests a tentative mechanism for such de novo actin polymerization. Thus, we found that Arp3, the major component of the Arp2/3 complex that nucleates new actin filaments (Suetsugu et al., 2002; Welch and Mullins, 2002), colocalizes with the F-actin rings (Figure 8A). Similar colocalization was found for cortactin, which activates the Arp2/3 complex and targets it to actin filaments (Weed et al., 2000; Urino et al., 2001). Furthermore, treatment with BDM, that has been shown to displace the Arp2/3 complex from F-actin (Yarrow et al., 2003), attenuated formation of contractile F-actin rings and disassembly of apical junctions (Figure 8B). Taken together, these data suggest that the Arp2/3-assisted de novo actin polymerization is essential for biogenesis of contractile F-actin rings during calcium depletion.
Role for Myosin IIA Motor in Biogenesis of F-actin Rings
Turnover of F-actin filaments does not appear to be solely responsible for creation of contractile actin rings and disruption of the AJC in calcium-depleted epithelial cells. Our data demonstrate a vital role for MNMM II motor in these processes. Myosin-driven contraction of perijunctional actin belt has been intensively studied in isolated intestinal brush borders (see for review Mooseker, 1985), although mechanisms of such contractility in living cells are poorly understood. Previous functional studies implicating myosin II in contraction of F-actin ring in calcium-depleted MDCK and Caco-2 cells, were based primarily on effects of BDM, which was thought to be a general myosin inhibitor (Castillo et al., 1998; Ma et al., 2000b). However, several recent studies showed that BDM, although blocking activity of skeletal muscle myosin II, does not inhibit MNMM II or any other nonmuscle myosins tested (Cheung et al., 2002; Ostap, 2002; Yarrow et al., 2003). Inhibitory effects of BDM on cell motility have been explained by its interference with Arp2/3-dependent polymerization of F-actin (Yarrow et al., 2003), and caution was urged with interpretation of old BDM experiments (Ostap, 2002; Titus, 2003). In the present study, we used blebbistatin, a highly selective inhibitor of MNMM II (Straight et al., 2003) and demonstrated complete prevention of formation of contractile F-actin rings and disassembly of the AJC in calcium-depleted T84 cells (Figure 9A). Amazingly, inhibition of myosin II activity also largely preserved barrier properties of calcium-depleted T84 monolayers (Figure 9B). Of all pharmacological inhibitors of the AJC disassembly used in the present work and in our previous study (Ivanov et al., 2004), blebbistatin is the only agent that has been observed to significantly inhibit the drop in TEER caused by calcium removal.
Mammalian cells express three isoforms of nonmuscle myosin II designated as MNMM IIA, IIB, and IIC (Murakami et al., 1991; Phillips et al., 1995; Golomb et al., 2004). The MNMM II isoforms are widely expressed in embryonic and adult tissues (Golomb et al., 2004) and MNMM IIA and IIB play distinct roles in cell motility (Maupin et al., 1994; Wylie and Chantler, 2001; Kolega, 2003) and vesicle trafficking (Togo and Steinhardt, 2004). Our data suggest a critical role for MNMM IIA in formation and closure of contractile F-actin rings in calcium-depleted T84 cells. Not only did we observe MNMM IIA as the predominant isoform in T84 cells (Figure 10A), but we also found its colocalization with contractile F-actin rings (Figure 10B). In addition, we observed that the amount of MNMM IIA tightly associated with F-actin is increased in calcium-depleted cells (Figure 10C) and that it is likely to be activated by association with phosphorylated RMLCs, which accumulate in the areas of actin rings (Figure 11). Although the MNMM IIA isoform was previously implicated in formation of protrusive force during cell migration (Saitoh et al., 2001; Kolega, 2003), our data together with findings of MNMM IIA in the cleavage furrow during cytokinesis (Obungu et al., 2003; Maupin et al., 1994) suggest that this myosin II isoform is involved in creation of contractile/retraction force.
In conclusion, our present study demonstrates that reorganization of the apical actin cytoskeleton mediates disassembly of the AJC during calcium depletion of intestinal epithelial cells. This reorganization leads to formation of contractile F-actin rings that likely creates tension and results in disassembly of the AJC and internalization of AJ/TJ proteins. Two processes cooperate in the formation and contraction of F-actin rings. The first is a rapid reorganization of F-actin involving cofilin-1/ADF-driven depolymerization and Arp2/3-dependent repolymerization, and the second is a myosin IIA-dependent contraction of actin rings. This actomyosin-driven disruption of the AJC in calcium-depleted cells may represent an exaggerated version of mechanisms responsible for rapid disassembly of apical junctions during normal remodeling of intestinal epithelium and in intestinal inflammation.
Acknowledgments
The authors thank Drs. R. Adelstein, J. Bamburg, and M. Welch for generous donation of antibodies, S. Voss and R. Santoianni for the excellent technical assistance, D. Hunt for help in the manuscript preparation, and Drs. S. Ono, A. Hopkins, and K. Mandell for valuable comments on the manuscript. This work was supported by National Institute of Health Grants DK 61379 (to C.A.P.), DK 55679, and DK 59888 (to A.N.), a Digestive Diseases Minicenter grant DK 64399, and a Senior Investigator Award from the Crohns and Colitis Foundation (to A.N.).
Notes
Article published online ahead of print. Mol. Biol. Cell 10.1091/mbc.E04-02-0163. Article and publication date are available at www.molbiolcell.org/cgi/doi/10.1091/mbc.E04-02-0163.
Abbreviations used: ADF, actin-depolymerizing factor; AJ, adherens junction; Arp2/3, actin related proteins 2/3; BDM, 2,3-butanedione monoxime; JAM, junctional adhesion molecule, MNMM, mammalian nonmuscle myosin, PFA, paraformaldehyde; RMLC, regulatory myosin light chain; TEER, transepithelial electrical resistance; TJ, tight junction; TX-100, Triton X-100.
References
- Araki, N., Hatae, T., Furukawa, A., and Swanson, J.A. (2002). Phosphoinositide-3-kinase-independent contractile activities associated with Fcγ-receptormediated phagocytosis and macropinocytosis in macrophages. J. Cell Sci. 116, 247-257. [Abstract] [Google Scholar]
- Ashworth, S.L., Sandoval, R.M., Hosford, M., Bamburg, J.R., and Molitoris, B.A. (2001). Ischemic injury induces ADF relocalization to the apical domain of rat proximal tubule cells. Am. J. Physiol. Renal Physiol. 280, F886-F894. [Abstract] [Google Scholar]
- Ashworth, S.L., Southgate, E.L., Sandoval, R.M., Meberg, P.J., Bamburg, J.R., and Molitoris, B.A. (2003). ADF/cofilin mediates actin cytoskeletal alterations in LLC-PK cells during ATP depletion. Am. J. Physiol. Renal Physiol. 284, F852-F862. [Abstract] [Google Scholar]
- Bamburg, J.R. (1999). Proteins of the ADF/cofilin family: essential regulators of actin dynamics. Annu. Rev. Cell Dev. Biol. 15, 185-230. [Abstract] [Google Scholar]
- Basuroy, S., Sheth, P., Kuppuswamy, D., Balasubramanian, S., Ray, R.M., and Ray, R.K. (2003). Expression of kinase-inactive s-Src delays oxidative stress-induced disassembly and accelerates calcium-mediated reassembly of tight junctions in the Caco-2 cell monolayer. J. Biol. Chem. 278, 11916-11924. [Abstract] [Google Scholar]
- Bement, W.M., Forscher, P., and Mooseker, M.S. (1993). A novel cytoskeletal structure involved in purse string wound closure and cell polarity maintenance. J. Cell Biol. 121, 565-578. [Europe PMC free article] [Abstract] [Google Scholar]
- Bement, W.M., Mandato, C.A., and Kirsch, M.N. (1999). Wound-induced assembly and closure of an actomyosin purse string in Xenopus oocytes. Curr. Biol. 9, 579-587. [Abstract] [Google Scholar]
- Bresnick, A.R. (1999). Molecular mechanisms of nonmuscle myosin-II regulation. Curr. Opin. Cell Biol. 11, 26-33. [Abstract] [Google Scholar]
- Bubb, M.R., Senderowicz, A.M.J., Sausville, E.A., Duncan, K.L.K., and Korn, E.D. (1994). Jasplakinolide, a cytotoxic natural product, induces actin polymerization and competitively inhibits the binding of phalloidin to F-actin. J. Biol. Chem. 269, 14869-14871. [Abstract] [Google Scholar]
- Bubb, M.R., Spector, I., Beyer, B.B., and Fosen, K.M. (2000). Effects of jasplakinolide on the kinetics of actin polymerization. An explanation for certain in vivo observations. J. Biol. Chem. 275, 5163-5170. [Abstract] [Google Scholar]
- Caldwell, R.B., Wade, L.A., and McLaughlin, B.J. (1984). A quantitative study of intramembrane changes during cell junctional breakdown in the dystrophic rat retinal pigment epithelium. Exp. Cell Res. 150, 104-117. [Abstract] [Google Scholar]
- Castillo, A.M., Lagunes, R., Urban, M., Frixione, E., and Meza, I. (1998). Myosin II-actin interaction in MDCK cells: role in cell shape changes in response to Ca2+ variations. J. Muscle Res. Cell Motil. 19, 557-574. [Abstract] [Google Scholar]
- Cereijido, M., Robbins, E.S., Dolan, W.J., Rotunno, C.A., and Sabatini, D.D. (1978). Polarized monolayers formed by epithelial cells on a permeable and translucent support. J. Cell Biol. 77, 853-880. [Europe PMC free article] [Abstract] [Google Scholar]
- Chen, J., and Wagner, M.C. (2001). Altered membrane-cytoskeleton linkage and membrane blebbing in energy-depleted renal proximal tubular cells. Am. J. Physiol. Renal Physiol. 280, F619-F627. [Abstract] [Google Scholar]
- Cheung, A., Dantzig, J.A., Hollingworth, S., Baylor, S.M., Goldman, Y.E., Mitchison, T.J., and Straight, A.F. (2002). A small-molecule inhibitor of skeletal muscle myosin II. Nat. Cell Biol. 4, 83-88. [Abstract] [Google Scholar]
- Citi, S., Volberg, T., Bershadsky, A.D., Denisenko, N., and Geiger, B. (1994). Cytoskeletal involvement in the modulation of cell-cell junctions by the protein kinase inhibitor H-7. J. Cell Sci. 107, 683-692. [Abstract] [Google Scholar]
- Cramer, L.P., Briggs, L.J., and Dawe, H.R. (2002). Use of fluorescently labelled deoxyribonuclease I to spatially measure G-actin levels in migrating and non-migrating cells. Cell Motil. Cytoskel. 51, 27-38. [Abstract] [Google Scholar]
- Decker, R.S. (1981). Disassembly of the zonula occludens during amphibian neurulation. Dev. Biol. 81, 12-22. [Abstract] [Google Scholar]
- Drubin, D.G., and Nelson, W.J. (1996). Origin of cell polarity. Cell 84, 335-344. [Abstract] [Google Scholar]
- Fanning, A.S. (2001). Organization and regulation of the tight junction by the actin-myosin cytoskeleton. In: Tight Junctions, 2nd ed., ed. M. Cereijido and J. Anderson, Boca Raton, FL: CRC Press, 265-284.
- Farquhar, M.G., and Palade, G.E. (1963). Junctional complexes in various epithelia. J. Cell Biol. 17, 375-412. [Europe PMC free article] [Abstract] [Google Scholar]
- Field, C., Li, R., and Oegema, K. (1999). Cytokinesis in eukaryotes: a mechanistic comparison. Curr. Opin. Cell Biol. 11, 68-80. [Abstract] [Google Scholar]
- Florian, P., Schoneberg, T., Schulzke, J.D., Fromm, M., and Gitter, A.H. (2002). Single-cell epithelial defects close rapidly by an actinomyosin purse string mechanism with functional tight junctions. J. Physiol. 545, 485-499. [Abstract] [Google Scholar]
- Fukuhara, A., Irie, K., Yamada, A., Katata, T., Honda, T., Shimizu, K., Nakanishi, H., and Takai, Y. (2002). Role of nectin in organization of tight junctions in epithelial cells. Genes Cells 7, 1059-1072. [Abstract] [Google Scholar]
- Golomb, E. et al. (2004). Identification and characterization of nonmuscle myosin II-C, a new member of the myosin II family. J. Biol. Chem. 279, 2800-2808. [Abstract] [Google Scholar]
- Gonzáles-Mariscal, L., Betanzos, A., Nava, P., and Jaramillo, B.E. (2003). Tight junction proteins. Prog. Biophys. Mol. Biol. 81, 1-44. [Abstract] [Google Scholar]
- Gumbiner, B.M. (1996). Cell adhesion: the molecular basis of tissue architecture and morphogenesis. Cell 84, 345-357. [Abstract] [Google Scholar]
- Han, X., Fink, M.P., and Delude, R.L. (2003). Proinflammatory cytokines cause NO-dependent and independent changes in expression and localization of tight junction proteins in intestinal epithelial cells. Shock 19, 229-237. [Abstract] [Google Scholar]
- Harhaj, N.S., Barber, A.J., and Antonetti, D.A. (2002). Platelet-derived growth factor mediates tight junction redistribution and increases permeability in MDCK cells. J. Cell. Physiol. 193, 349-364. [Abstract] [Google Scholar]
- Henson, J.H., Nazarian, R., Schulberg, K.L., Trabosh, V.A., Kolnik, S.E., Burns, A.R., and McPartland, K.J. (2002). Wound closure in the lamellipodia of single cells: mediation by actin polymerization in the absence of an actomyosin purse string. Mol. Biol. Cell 13, 1001-1014. [Europe PMC free article] [Abstract] [Google Scholar]
- Hopkins, A.M., Walsh, S.V., Verkade, P., Boquet, P., and Nusrat, A. (2003). Constitutive activation of Rho proteins by CNF-1 influences tight junction structure and epithelial barrier function. J. Cell Sci. 116, 725-742. [Abstract] [Google Scholar]
- Ivanov, A.I., Nusrat, A., and Parkos, C.A. (2004). Endocytosis of epithelial apical junctional proteins by a clathrin-mediated pathway into a unique storage compartment. Mol. Biol. Cell 15, 176-188. [Europe PMC free article] [Abstract] [Google Scholar]
- Jarrett, O., Stow, J.L., Yap, A.S., and Key, B. (2002). Dynamin-dependent endocytosis is necessary for convergent-extension movements in Xenopus animal cap explants. Int. J. Dev. Biol. 46, 467-473. [Abstract] [Google Scholar]
- Kamei, T., Matozaki, T., Sakisaka, T., Kodama, A., Yokoyama, S., Peng, Y.F., Nakano, K., Takaishi, K., and Takai, Y. (1999). Coendocytosis of cadherin and c-Met coupled to disruption of cell-cell adhesion in MDCK cells—regulation by Rho, Rac and Rab small G proteins. Oncogene 18, 6776-6784. [Abstract] [Google Scholar]
- Kartenbeck, J., Schmelz, M., Franke, W.W., and Geiger, B. (1991). Endocytosis of junctional cadherins in bovine kidney epithelial (MDBK) cells cultured in low Ca2+ ion medium. J. Cell Biol. 113, 881-892. [Europe PMC free article] [Abstract] [Google Scholar]
- Kolega, J. (2003). Asymmetric distribution of myosin IIB in migrating endothelial cells is regulated by a Rho-dependent kinase and contributes to tail retraction. Mol. Biol. Cell 14, 4745-4757. [Europe PMC free article] [Abstract] [Google Scholar]
- Lappalainen, P., and Drubin, D.G. (1997). Cofilin promotes rapid actin filament turnover in vivo. Nature 388, 78-82. [Abstract] [Google Scholar]
- Le, T.L., Yap, A.S., and Stow, J.L. (1999). Recycling of E-cadherin: a potential mechanism for regulating cadherin dynamics. J. Cell Biol. 146, 219-232. [Europe PMC free article] [Abstract] [Google Scholar]
- Liu, Y., Nusrat, A., Schnell, F.J., Reaves, T.A., Walsh, S., Pochet, M., and Parkos, C.A. (2000). Human junctional adhesion molecules regulate tight junction resealing in epithelia. J. Cell Sci. 113, 2363-2374. [Abstract] [Google Scholar]
- Ma, T.Y., Hoa, N.T., Tran, D.D., Bui, V., Pedram, A., Mills, S., and Merryfield, M. (2000a). Cytochalasin B modulation of Caco-2 tight junction barrier: role of myosin light chain kinase. Am. J. Physiol. Gastrointest. Liver Physiol. 279, G875-G885. [Abstract] [Google Scholar]
- Ma, T.Y., Tran, D., Hoa, N., Nguyen, D., Merryfield, M., and Tarnawski, A. (2000b). Mechanism of extracellular calcium regulation of intestinal epithelial tight junction permeability: role of cytoskeletal involvement. Microsc. Res. Tech. 51, 156-168. [Abstract] [Google Scholar]
- Madara, J.L., Stafford, J., Barenberg, D., and Carlson, S. (1988). Functional coupling of tight junctions and microfilaments in T84 monolayers. Am. J. Physiol. Gastrointest. Liver Physiol. 254, G416-G423. [Abstract] [Google Scholar]
- Madara, J.L. (1990). Maintenance of the macromolecular barrier at cell extrusion sites in intestinal epithelium: physiological rearrangement of tight junctions. J. Membr. Biol. 116, 177-184. [Abstract] [Google Scholar]
- Madara, J.L. (1998). Regulation of the movement of solutes across tight junctions. Annu. Rev. Physiol. 60, 143-159. [Abstract] [Google Scholar]
- Mandato, C.A., and Bement, W.M. (2001). Contraction and polymerization cooperate to assemble and close actomyosin rings around Xenopus oocyte wounds. J. Cell Biol. 154, 785-797. [Europe PMC free article] [Abstract] [Google Scholar]
- Matsuda, M., Kubo, A., Furuse, M., and Tsukita, S. (2004). A peculiar internalization of claudins, tight junction-specific adhesion molecules, during the intercellular movement of epithelial cells. J. Cell Sci. 117, 1247-1257. [Abstract] [Google Scholar]
- Matter, K., and Balda, M.S. (2003). Signaling to and from tight junctions. Nat. Rev. Mol. Cell. Biol. 4, 225-236. [Abstract] [Google Scholar]
- Maupin, P., Phillips, C.L., Adelstein, R.S., and Pollard, T.D. (1994). Differential localization of myosin-II isozymes in human cultured cells and blood cells. J. Cell Sci. 107, 3077-3090. [Abstract] [Google Scholar]
- Meberg, P.J., Ono, S., Minamide, L.S., Takahashi, M., and Bamburg, J.R. (1998). Actin deploymerizing factor and cofilin phosphorylation dynamics: responses to signals that regulate neurite extension. Cell Motil. Cytoskel. 39, 172-190. [Abstract] [Google Scholar]
- Miller, J.R., and McClay, D.R. (1997). Characterization of the role of cadherin in regulating cell adhesion during sea urchin development. Dev. Biol. 192, 323-339. [Abstract] [Google Scholar]
- Moon, A., and Drubin, D.G. (1995). The ADF/cofilin proteins: stimulus-responsive modulators of actin dynamics. Mol. Biol. Cell 6, 1423-1431. [Europe PMC free article] [Abstract] [Google Scholar]
- Mooseker, M.S. (1985). Organization, chemistry, and assembly of the cytoskeletal apparatus of the intestinal brush border. Annu. Rev. Cell Biol. 1, 209-241. [Abstract] [Google Scholar]
- Morgan, T.E., Lockerbie, R.O., Minamide, L.S., Browning, M.D., and Bamburg, J.R. (1993). Isolation and characterization of a regulated form of actin depolymerizing factor. J. Cell Biol. 122, 623-633. [Europe PMC free article] [Abstract] [Google Scholar]
- Morton, W.M., Ayscough, K.R., and McLaughlin, P.J. (2000). Latrunculin alters the actin-monomer subunit interface to prevent polymerization. Nat. Cell Biol. 2, 376-378. [Abstract] [Google Scholar]
- Murakami, N., Mehta, P., and Elzinga, M. (1991). Studies on the distribution of cellular myosin with antibodies to isoform-specific synthetic peptides. FEBS Lett. 288, 23-25. [Abstract] [Google Scholar]
- Noguchi, T., Arai, R., Motegi, F., Nakano, K., and Mabuchi, I. (2001). Contractile ring formation in Xenopus egg and fission yeast. Cell Struct. Funct. 26, 545-554. [Abstract] [Google Scholar]
- Nusrat, A., von Eichel-Streiber, C., Turner, J.R., Verkade, P., Madara, J.L., and Parkos, C.A. (2001). Clostridium difficile toxins disrupt epithelial barrier function by altering membrane microdomain localization of tight junction proteins. Infect. Immun. 69, 1329-1336. [Europe PMC free article] [Abstract] [Google Scholar]
- Obungu, V.H., Burns, A.L., Agarwal, S.K., Chandrasekharapa, S.C., Adelstein, R.S., and Marx, S.J. (2003). Menin, a tumor suppressor, associates with nonmuscle myosin II-A heavy chain. Oncogene 22, 6347-6358. [Abstract] [Google Scholar]
- Ono, S. (2003). Regulation of actin filament dynamics by actin depolymerizing factor/cofilin and actin-interacting protein 1, new blades for twisted filaments. Biochemistry 42, 13363-13370. [Abstract] [Google Scholar]
- Ostap, E.M. (2002). 2,3-Butanedione monoxime (BDM) as a myosin inhibitor. J. Muscle Res. Cell Motil. 23, 305-308. [Abstract] [Google Scholar]
- Pantaloni, D., Le Clainche, C., and Carlier, M-F. (2001). Mechanism of actin based motility. Science 292, 1502-1506. [Abstract] [Google Scholar]
- Peifer, M., and Yap, A.S. (2003). Traffic control: p120 catenin acts as a gatekeeper to control the fate of classical cadherins in mammalian cells. J. Cell Biol. 163, 437-440. [Europe PMC free article] [Abstract] [Google Scholar]
- Pelham, R.J., and Chang, F. (2002). Actin dynamics in the contractile ring during cytokinesis in fission yeast. Nature 419, 82-86. [Abstract] [Google Scholar]
- Phillips, C.L., Yamakawa, K., and Adelstein, R.S. (1995). Cloning of the cDNA encoding human nonmuscle myosin heavy chain-B and analysis of human tissues with isoform-specific antibodies. J. Muscle Res. Cell Motil. 16, 379-389. [Abstract] [Google Scholar]
- Pitelka, D.R., Taggart, B.N., and Hamamoto, S.T. (1983). Effect of extracellular calcium depletion on membrane topography and occluding junctions of mammary epithelial cells in culture. J. Cell Biol. 96, 613-624. [Europe PMC free article] [Abstract] [Google Scholar]
- Pollard, T.D., and Borisy, G.G. (2003). Cellular motility driven by assembly and disassembly of actin filaments. Cell 112, 453-465. [Abstract] [Google Scholar]
- Rosenblatt, J., Raff, M.C., and Cramer, L.P. (2001). An epithelial cell destined for apoptosis signals its neighbors to extrude it by an actin- and myosindependent mechanism. Curr. Biol. 11, 1847-1857. [Abstract] [Google Scholar]
- Saitoh, T., Takemura, S., Ueda, K., Hosoya, H., Nagayama, M., Haga, H., Kawabata, K., Yamagishi, A., and Takahashi, M. (2001). Differential localization of non-muscle myosin II isoforms and phosphorylated regulatory light chains in human MRC-5 fibroblasts. FEBS Lett. 509, 365-369. [Abstract] [Google Scholar]
- Scott, K.G.E., Meddings, J.B., Kirk, D.R., Lees-Miller, S.P., and Buret, A.G. (2002). Intestinal infection with Giardia spp. reduces epithelial barrier function in a myosin light chain kinase-dependent fashion. Gastroenterology 123, 1179-1190. [Abstract] [Google Scholar]
- Siliciano, J.D., and Goodenough, D.A. (1988). Localization of the tight junction protein, ZO-1, is modulated by extracellular calcium and cell-cell contacts in Madin-Darby canine kidney epithelial cells. J. Cell Biol. 107, 2389-2399. [Europe PMC free article] [Abstract] [Google Scholar]
- Sokac, A.M., Co, C., Taunton, J., and Bement, W. (2003). Cdc42-dependent actin polymerization during compensatory endocytosis in Xenopus eggs. Nat. Cell. Biol. 5, 727-732. [Abstract] [Google Scholar]
- Spudich, J.A. (2001). The myosin swinging cross-bridge model. Nat. Rev. Mol. Cell Biol. 2, 387-392. [Abstract] [Google Scholar]
- Stevenson, B.R., and Begg, D.A. (1994). Concentration-dependent effects of cytochalasin D on tight junctions and actin filaments in MDCK epithelial cells. J. Cell Sci. 107, 367-375. [Abstract] [Google Scholar]
- Straight, A.F., Cheung, A., Limouze, J., Chen, I., Westwood, N.J., Sellers, J.R., and Mitchison, T.J. (2003). Dissecting temporal and spatial control of cytokinesis with a myosin II inhibitor. Science 299, 1743-1747. [Abstract] [Google Scholar]
- Suetsugu, S., Miki, H., and Takenawa, T. (2002). Spatial and temporal regulation of actin polymerization for cytoskeleton formation through Arp2/3 complex and WASP/WAVE proteins. Cell Motil. Cytoskel. 51, 113-122. [Abstract] [Google Scholar]
- Symons, M.H., and Mitchison, T.J. (1991). Control of actin polymerization in live and permeabilized fibroblasts. J. Cell Biol. 114, 503-513. [Europe PMC free article] [Abstract] [Google Scholar]
- Takai, Y., and Nakanishi, H. (2003). Nectin and afadin: novel organizers of intercellular junctions. J. Cell Sci. 116, 17-27. [Abstract] [Google Scholar]
- Tan, J.L., Ravid, S., and Spudich, J.A. (1992). Control of nonmuscle myosins by phosphorylation. Annu. Rev. Biochem. 61, 721-759. [Abstract] [Google Scholar]
- Titus, M.A. (2003). Caveat experimentor—is your myosin really inhibited? Nat. Cell Biol. 5, 95. [Abstract] [Google Scholar]
- Togo, T., and Steinhardt, R.A. (2004). Nonmuscle myosin IIA and IIB have distinct functions in the exocytosis-dependent process of cell membrane repair. Mol. Biol. Cell 15, 688-695. [Europe PMC free article] [Abstract] [Google Scholar]
- Troyanovsky, R.B., Klingelhofer, J., and Troyanovsky, S. (1999). Removal of calcium triggers a novel type of intercadherin interaction. J. Cell Sci. 112, 4379-4387. [Abstract] [Google Scholar]
- Tsukita, S., Furuse, M., and Itoh, M. (2001). Multifunctional strands in tight junctions. Nat. Rev. Mol. Cell. Biol. 2, 285-293. [Abstract] [Google Scholar]
- Turner, J.R. (2000). `Putting the squeeze' on the tight junction: understanding cytoskeletal regulation. Semin. Cell Dev. Biol. 11, 301-308. [Abstract] [Google Scholar]
- Turner, J.R., Black, E.D., Ward, J., Tse, C.M., Uchwat, F.A., Alli, H.A., Donowitz, M., Madara, J.L., and Angle, J.M. (2000). Transepithelial resistance can be regulated by the intestinal brush-border Na+/H+ exchanger NHE3. Am. J. Physiol. Cell Physiol. 279, C1918-C1924. [Abstract] [Google Scholar]
- Urbanik, E., and Ware, B.R. (1989). Actin filament capping and cleaving activity of cytochalasins B, D, E, and H. Arch. Biochem. Biophys. 269, 181-187. [Abstract] [Google Scholar]
- Urino, T., Liu, J., Zhang, P., Fan, Y.X., Egile, C., Li, R., Mueller, S.C., and Zhan, X. (2001). Activation of Arp2/3 complex-mediated actin polymerization by cortactin. Nat. Cell Biol. 3, 259-266. [Abstract] [Google Scholar]
- Vasioukhin, V., and Fuchs, E. (2001). Actin dynamics and cell-cell adhesion in epithelia. Curr. Opin. Cell Biol. 13, 76-84. [Abstract] [Google Scholar]
- Vleminckx, K., and Kemler, R. (1999). Cadherins and tissue formation: integrating adhesion and signaling. BioEssays 21, 211-220. [Abstract] [Google Scholar]
- Volberg, T., Geiger, B., Kartenbeck, J., and Franke, W.W. (1986). Changes in membrane-microfilament interaction in intercellular adherens junctions upon removal of extracellular Ca2+ ions. J. Cell Biol. 102, 1832-1842. [Europe PMC free article] [Abstract] [Google Scholar]
- Weed, S.A., Karginov, A.V., Schafer, D.A., Weaver, A.M., Kinley, A.W., Cooper, J.A., and Parsons, J.T. (2000). Cortactin localization to sites of actin assembly in lamellipodia requires interactions with F-actin and the Arp2/3 complex. J. Cell Biol. 151, 29-40. [Europe PMC free article] [Abstract] [Google Scholar]
- Welch, M.D., and Mullins, R.D. (2002). Cellular control of actin nucleation. Annu. Rev. Cell Dev. Biol. 18, 247-288. [Abstract] [Google Scholar]
- Wylie, S.R., and Chantler, P.D. (2001). Separate but linked functions of conventional myosins modulate adhesion and neurite outgrowth. Nat. Cell Biol. 3, 88-92. [Abstract] [Google Scholar]
- Yap, A.S., Brieher, W.M., and Gumbiner, B.M. (1997). Molecular and functional analysis of cadherin-based adherens junctions. Annu. Rev. Cell Dev. Biol. 13, 119-146. [Abstract] [Google Scholar]
- Yarrow, J.C., Lechler, T., Li, R., and Mitchison, T.J. (2003). Rapid de-localization of actin leading edge components with BDM treatment. BMC Cell Biol. 4, 5. [Europe PMC free article] [Abstract] [Google Scholar]
- Yeaman, C., Grindstaff, K.K., and Nelson, W.J. (1999). New perspectives on mechanisms involved in generating epithelial cell polarity. Physiol. Rev. 79, 73-98. [Abstract] [Google Scholar]
- Zahrebelski, G., Nieminen, A.L., al-Ghoul, K., Qian, T., Herman, B., and Lemasters, J.J. (1995). Progression of subcellular changes during chemical hypoxia to cultured rat hepatocytes: a laser scanning confocal microscopic study. Hepatology 21, 1361-1372. [Abstract] [Google Scholar]
Articles from Molecular Biology of the Cell are provided here courtesy of American Society for Cell Biology
Full text links
Read article at publisher's site: https://doi.org/10.1091/mbc.e04-02-0163
Read article for free, from open access legal sources, via Unpaywall:
https://europepmc.org/articles/pmc420089?pdf=render
Citations & impact
Impact metrics
Citations of article over time
Article citations
Whole Genome Resequencing Reveals Selection Signals Related to Wool Color in Sheep.
Animals (Basel), 13(20):3265, 19 Oct 2023
Cited by: 5 articles | PMID: 37893989 | PMCID: PMC10603731
Actin-dependent recruitment of AGO2 to the zonula adherens.
Mol Biol Cell, 34(13):ar129, 11 Oct 2023
Cited by: 2 articles | PMID: 37819702 | PMCID: PMC10848941
Inflammation modulates intercellular adhesion and mechanotransduction in human epidermis via ROCK2.
iScience, 26(3):106195, 14 Feb 2023
Cited by: 3 articles | PMID: 36890793 | PMCID: PMC9986521
Understanding disruption of the gut barrier during inflammation: Should we abandon traditional epithelial cell lines and switch to intestinal organoids?
Front Immunol, 14:1108289, 16 Feb 2023
Cited by: 17 articles | PMID: 36875103 | PMCID: PMC9983034
Review Free full text in Europe PMC
Identification of lysophosphatidic acid in serum as a factor that promotes epithelial apical junctional complex organization.
J Biol Chem, 298(10):102426, 27 Aug 2022
Cited by: 2 articles | PMID: 36030821 | PMCID: PMC9520027
Go to all (141) article citations
Similar Articles
To arrive at the top five similar articles we use a word-weighted algorithm to compare words from the Title and Abstract of each citation.
Differential roles for actin polymerization and a myosin II motor in assembly of the epithelial apical junctional complex.
Mol Biol Cell, 16(6):2636-2650, 30 Mar 2005
Cited by: 156 articles | PMID: 15800060 | PMCID: PMC1142412
Microtubules regulate disassembly of epithelial apical junctions.
BMC Cell Biol, 7:12, 01 Mar 2006
Cited by: 56 articles | PMID: 16509970 | PMCID: PMC1444913
Constitutive activation of Rho proteins by CNF-1 influences tight junction structure and epithelial barrier function.
J Cell Sci, 116(pt 4):725-742, 01 Feb 2003
Cited by: 139 articles | PMID: 12538773
RhoGTPases, actomyosin signaling and regulation of the epithelial Apical Junctional Complex.
Semin Cell Dev Biol, 36:194-203, 16 Sep 2014
Cited by: 64 articles | PMID: 25223584 | PMCID: PMC5054512
Review Free full text in Europe PMC
Funding
Funders who supported this work.
NIDDK NIH HHS (9)
Grant ID: DK59888
Grant ID: R29 DK055679
Grant ID: R01 DK059888
Grant ID: R01 DK061379
Grant ID: R24 DK064399
Grant ID: DK 55679
Grant ID: DK 61379
Grant ID: DK 64399
Grant ID: R01 DK055679