Abstract
Free full text

The ribonuclease activity of SAMHD1 is required for HIV-1 restriction
Associated Data
Abstract
The HIV-1 restriction factor SAMHD11,2 is proposed to inhibit HIV-1 replication by depleting the intracellular dNTP pool3-5. However, the phosphorylation of SAMHD1 regulates its ability to restrict HIV-1 without decreasing cellular dNTP levels6-8, which is not consistent with a role for SAMHD1 dNTPase activity in HIV-1 restriction. Here, we show that SAMHD1 possesses RNase activity and that the RNase but not the dNTPase function is essential for HIV-1 restriction. By enzymatically characterizing Aicardi-Goutières syndrome (AGS)-associated SAMHD1 mutations and mutations in the allosteric dGTP-binding site of SAMHD1, we identify SAMHD1 mutants that are RNase-positive but dNTPase-negative (SAMHD1D137N) or RNase-negative but dNTPase-positive (SAMHD1Q548A). The allosteric mutant SAMHD1D137N is able to restrict HIV-1 infection, whereas the AGS mutant SAMHD1Q548A is defective for HIV-1 restriction. SAMHD1 associates with HIV-1 RNA and degrades it during the early phases of infection. SAMHD1 silencing in macrophages and CD4+ T cells from healthy donors increases HIV-1 RNA stability, rendering the cells permissive for HIV-1 infection. Furthermore, the phosphorylation of SAMHD1 at T592 negatively regulates its RNase activity in vivo and impedes HIV-1 restriction. Our results reveal that the RNase activity of SAMHD1 is responsible for preventing HIV-1 infection by directly degrading the HIV-1 RNA.
Mutations in SAMHD1 cause Aicardi-Goutières syndrome (AGS), which is a genetic autoimmune disorder that mimics a congenital viral infection9,10. SAMHD1 is a myeloid cell-specific HIV-1 restriction factor that is counteracted by Vpx1,2. SAMHD1 possesses a dGTP-dependent dNTPase activity11,12, and studies have proposed that SAMHD1 blocks HIV-1 infection by depleting the intracellular dNTP pool3-5. Contrary to the proposed role for dNTPase activity in SAMHD1-mediated restriction of HIV-1, the phosphorylation of SAMHD1 regulates the ability of this enzyme to restrict HIV-1 infection but does not alter the cellular dNTP levels6-8. In addition, SAMHD1 is a nucleic acid binding protein13-15 and exhibits a nuclease activity in vitro against diverse nucleic acid substrates16. Although the physiological relevance of SAMHD1 nuclease activity remains unknown, the aforementioned characteristics suggest that SAMHD1 might bind to HIV-1 RNA and degrade this RNA or reverse transcription intermediates.
In contrast to Beloglazova et al.16, other studies have not observed SAMHD1 nuclease activity11-13; therefore, we tested whether SAMHD1 possesses nuclease activity. We incubated full-length, wild-type human SAMHD1 (SAMHD1WT) (Supplementary Fig. 1) with various types of nucleic acid substrates. SAMHD1WT hydrolyzed both 20- and 30-mer single-stranded RNAs (ssRNA) processively (Fig. 1a and Supplementary Fig. 2a). No nuclease activity was detected on double-stranded RNA (dsRNA), single-stranded DNA (ssDNA), double-stranded DNA (dsDNA), and RNA-DNA hybrid substrates, whereas these substrates were digested by positive control nucleases (Supplementary Fig. 2b–e) (substrate sequences are shown in Supplementary Table 1). A previous study16 observed that SAMHD1 degrades ssRNA, ssDNA, and RNA in DNA-RNA hybrids; however, we observed that only ssRNAs, including in vitro-HIV-1 transcripts (Supplementary Fig. 3), were sensitive to SAMHD1 digestion when the experiments were repeated using the various types of 40-mer nucleic acid substrates described by Beloglazova et al.16 (Supplementary Fig. 4). These discrepancies might be due to differences in assay conditions or protein purification procedures; however, our results are consistent with the observation of Beloglazova et al.16 that SAMHD1 cleaves ssRNAs in vitro. The degradation of ssRNA by SAMHD1 required Mg2+ (Supplementary Fig. 5) and correlated with the presence of the SAMHD1WT protein across chromatographic fractions (Supplementary Fig. 6). Considering the preferential binding of SAMHD1 to ssRNA compared with ssDNA13,15 and no interaction between endogenous SAMHD1 and dsRNA, dsDNA or RNA-DNA hybrids15, we concluded that SAMHD1 is an RNase that digests ssRNA.
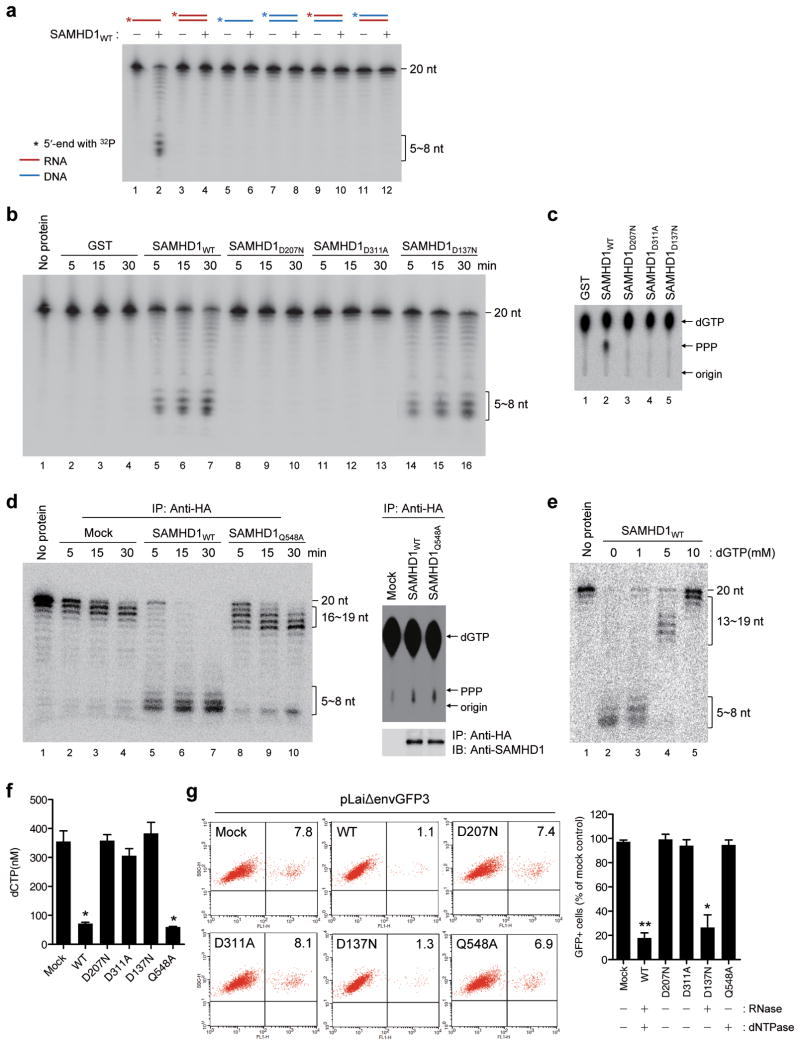
The RNase but not the dNTPase function of SAMHD1 is required for HIV-1 restriction. (a) Purified GST-SAMHD1WT (150 nM) was incubated for 30 min at 37 °C with 5′-end 32P labeled nucleic acid substrates (20-mer, 50 nM). The RNA and DNA are indicated by red and blue lines, respectively. (b) An RNase activity assay performed for SAMHD1 mutants as described in Fig. 1a using 20-mer ssRNA substrates. (c) A dGTP-triphosphohydrolase assay was performed for GST-SAMHD1 variants as described previously3. (d) The HA-tagged SAMHD1WT and SAMHD1Q548A proteins were purified from the PMA-differentiated U937 cells using HA-antibody. An RNase and a dGTP triphosphohydrolase assays were performed as described above. (e) Inhibition of the SAMHD1 RNase activity by dGTP. The assay was performed as in a in the presence of dGTP. (f) The intracellular dNTP pools were measured in U937 cells stably expressing SAMHD1 variants as described26. (g) U937 cells stably expressing SAMHD1mutants were treated with PMA and infected by pLaiΔenvGFP3, a HIV-1-GFP reporter virus with the LAI backbone27. After 48 h, the percentages of GFP-positive cells were analyzed using flow cytometry. The percentage of GFP-positive cells was calculated relative to the number of GFP-positive mock-transfected cells. In f and g, the data are presented as the mean ± s.d. of triplicate independent experiments. (* and ** indicate significant differences compared with the mock-transfected control at P < 0.05 and P < 0.001, respectively, using the two-tailed Student's t test).
Unlike dNTPase activity, RNase activity does not require the cofactor dGTP (Fig. 1a); therefore, we proposed that these two catalytic functions of SAMHD1 might be physically separable. We characterized AGS-associated SAMHD1 mutations and mutations near the allosteric sites for defects in RNase or dNTPase activity and identified several point mutations that caused loss of function. Mutations at residues 207 and 311 in the catalytic site abolished both RNase and dNTPase activities (Figs. 1b,c). The allosteric mutant SAMHD1D137N had no effect on the RNase activity but did abolish the dNTPase activity (Figs. 1b,c). The AGS mutant SAMHD1Q548A showed the opposite phenotype. Unlike the processive degradation seen in SAMHD1WT and SAMHD1D137N, a mutation at Q548 caused the enzyme to digest ssRNA in a distributive manner featured by accumulation of intermediate size fragments (16~19-mer) (Fig. 1d). Considering that a shift from a processive to distributive degradation often occurs under the conditions that decrease the affinity of exonuclease to the substrates17, this switch in mode may be because of a lowered affinity of SAMHD1Q548A to ssRNA. Indeed, recent studies have shown that SAMHD1Q548A exhibits impaired ssRNA-binding15,16. Notably, dGTP inhibited the RNase activity of SAMHD1 on ssRNA substrates, and this inhibition correlated with a shift from a processive to a distributive hydrolysis (Fig. 1e), suggesting that dGTP interferes with the binding of ssRNA substrates to SAMHD1. The identification of the SAMHD1 mutants that retain only one of two enzymatic activities enabled us to determine the discrete contribution of SAMHD1 RNase activity to HIV-1 restriction. For this analysis, we stably expressed SAMHD1 mutants in U937 pro-monocytic cells, which do not express endogenous SAMHD11,3,6,7 (Supplementary Fig. 7). Consistent with in vitro data (Fig. 1c,d), SAMHD1D207N, SAMHD1D311A, and SAMHD1D137N did not reduce the intracellular levels of dNTPs, whereas SAMHD1Q548A decreased the cellular levels of dNTPs (Fig. 1f). As expected, SAMHD1D207N and SAMHD1D311A did not restrict HIV-1 infection. Notably, SAMHD1D137N restricted HIV-1 infection to nearly the same extent as SAMHD1WT. SAMHD1Q548A was unable to block HIV-1 restriction despite its ability to decrease the cellular levels of dNTPs (Fig. 1g). These data suggest that the RNase but not the dNTPase function of SAMHD1 is essential for HIV-1 restriction.
The quantification of viral cDNA intermediates showed that SAMHD1D137N inhibited the synthesis of both the early and late viral cDNA products (Supplementary Fig. 8), indicating that SAMHD1 RNase functions at the early step of HIV-1 reverse transcription. Thus, we tested whether SAMHD1 RNase activity affects HIV-1 RNA levels. HIV-1 RNA was significantly reduced in cells expressing SAMHD1WT or SAMHD1D137N 3 and 6 h post-infection (hpi) (Fig. 2a), but SAMHD1D207N, SAMHD1D311A, and SAMHD1Q548A did not reduce the HIV-1 RNA level; these results were consistent with FACS analyses (Fig. 1g). To exclude primer-specificity bias, 12 pairs of primers spanning the entire HIV-1 genomic RNA (Supplementary Table 2) were used to amplify fragments from HIV-1-infected cells. The HIV-1 RNA level in SAMHD1WT-expressing cells was distinctly lower compared with the mock-transfected cells (Supplementary Fig. 9). To exclude the effect of the viral reverse transcriptase RNase H activity on the HIV-1 RNA level, we used RNase H-defective HIV-1 (HIV-1D443N) in which the Asp-443 of reverse transcriptase was mutated to Asn (D443N)18. The HIV-1D443N RNA level was lower in SAMHD1WT-expressing cells compared with mock-transfected cells (Fig. 2b), indicating that SAMHD1 can degrade HIV-1 RNA in the absence of reverse transcription.
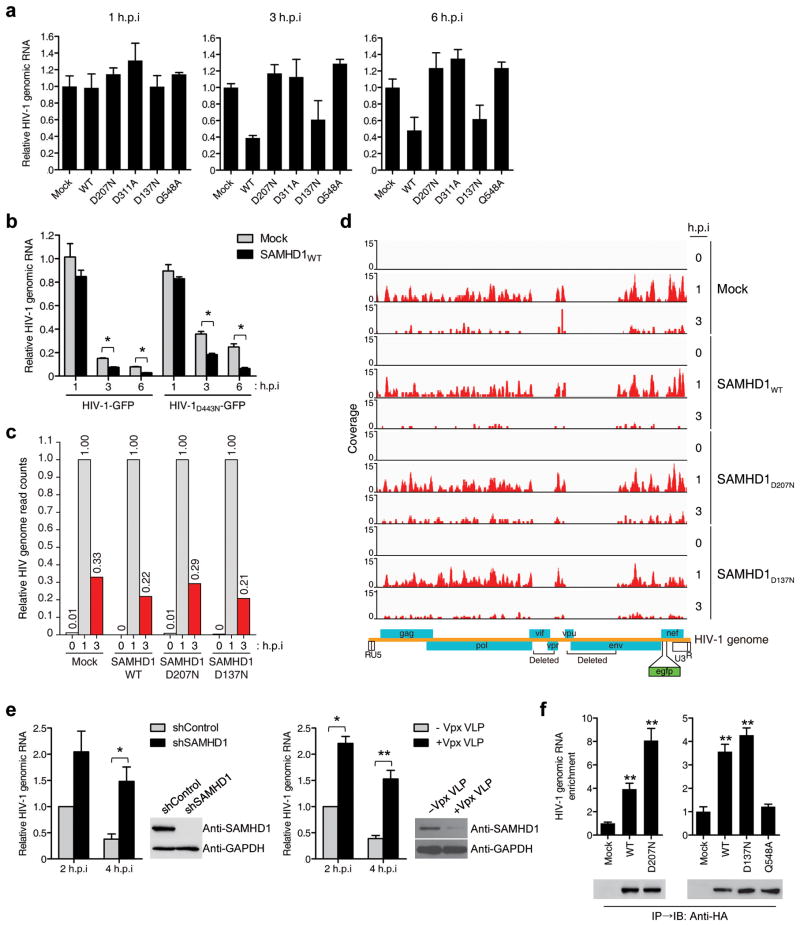
SAMHD1 directly degrades HIV-1 RNA in human monocytic cells. (a) The total cellular RNA was extracted from SAMHD1-expressing, HIV-1-GFP-infected U937 cells. The HIV-1 RNA content was quantified by qRT-PCR using HIV-1 gag-specific primers. The data were normalized to an internal β-actin. (b) Mock- and SAMHD1WT-expressing U937 cells were infected with HIV-1-GFP or HIV-1D443N-GFP. The HIV-1 RNA content was quantified by qRT-PCR using HIV-1 gfp-specific primers. (c) Relative HIV-1 genome abundance in reads per kilobase per million mapped reads (RPKM) calculated from RNA-Seq data. (d) Coverage of the RNA-Seq data across the HIV-1 genome in U937 cells expressing SAMHD1WT, SAMHD1D207N and SAMHD1D137N after HIV-1-GFP infection. Data are representative of two independent experiments (c,d). (e) shRNA-mediated silencing of SAMHD1 (left) or Vpx-mediated degradation of SAMHD1 (right) in THP-1 cells increases HIV-1 RNA stability. THP-1 cells expressing shRNAs, Vpx or negative control were treated with PMA for 24 h followed by infection with HIV-1-GFP. The total viral RNA was quantified by qRT-PCR at the indicated times post-infection. Knockdown efficiency was determined by immunoblot analysis. (f) Enrichment of HIV-1 genomic RNA in the SAMHD1 immunoprecipitates. U937 cells expressing HA-tagged SAMHD1 variants were infected for 90 min with HIV-1-GFP. The purified RNAs in the anti-HA immunoprecipitates were quantified by qRT-PCR using HIV-1 gag-specific primers. In a, b, e, and f, data are presented as the mean ± s.d. of triplicate independent experiments. (*P < 0.05 and **P < 0.001 versus Mock or shControl, two-tailed Student's t test).
RNA-Seq analysis also confirmed the degradation of HIV-1 RNA by SAMHD1. At 3 hpi, the relative read count of HIV-1 RNA in SAMHD1WT and SAMHD1D137N-expressing cells was lower than that in mock-transfected or SAMHD1D207N-expressing cells despite the considerable reduction of HIV-1 RNA levels in all cell types compared with the levels observed at 1 hpi (Figs. 2c,d). shRNA-mediated knockdown of SAMHD1 or Vpx-mediated degradation of endogenous SAMHD1 in THP-1 cells also caused an approximately four-fold increase in the HIV-1 RNA level (Fig. 2e). RNA immunoprecipitation (RIP) analysis19 revealed that a significant enrichment of HIV-1 RNA was observed in the immunoprecipitates from cells expressing SAMHD1WT-HA. The HIV-1 RNA enrichment was more pronounced for the SAMHD1D207N-HA catalytic mutant with an approximately eight-fold increase (Fig. 2f), suggesting that SAMHD1 associates with HIV-1 genomic RNA. In contrast, interaction of SAMHD1Q548A-HA with HIV-1 RNA was significantly reduced when compared with the SAMHD1WT-HA (Fig. 2f), supporting that impaired binding of SAMHD1Q548A-HA to HIV-1 RNA accounts for its inability to cleave short ssRNA and HIV-1 RNA (Figs. 1d and and2a2a).
Next, we analyzed the role of SAMHD1 RNase in degrading HIV-1 RNA in primary human monocyte-derived macrophages (MDMs) and CD4+ T cells. The differentiation of MDM and activation state of CD4+ T cells were analyzed by monitoring their surface markers (Supplementary Figs. 10 and 11). SAMHD1 silencing in MDMs markedly reduced the cellular SAMHD1 levels (Fig. 3a, left) and rendered MDMs more permissive to HIV-1 infection (Fig. 3a and Supplementary Fig. 12), a result that is correlated with the increased stability of HIV-1 RNA. The enhancement of HIV-1 RNA stability ranged from approximately two to four-fold, depending on the donor (Fig. 3a, middle). Similar to the results obtained using MDMs, SAMHD1 silencing in resting CD4+ T cells increased the infectivity of HIV-1 (Fig. 3b and Supplementary Fig. 12). In these cells, increased HIV-1 RNA stability was observed 3 hpi (Fig. 3b, middle). The degradation of SAMHD1 by Vpx could also stabilize the viral RNAs of HIV-1 and HIV-1D443N in resting CD4+ T cells (Fig. 3c). These results show that the antiviral activity of SAMHD1 correlates with its RNase activity in primary MDMs and resting CD4+ T cells. Biochemical fractionation revealed that SAMHD1 is localized in both the cytoplasm and nucleus of MDMs and monocytes (Supplementary Fig. 13), which is consistent with previous observations4,13. Considering that cytoplasmic SAMHD1 can block HIV-1 infection20,21, these data suggest that after virus uncoating, SAMHD1 associates with and degrades HIV-1 RNA in the cytoplasm.
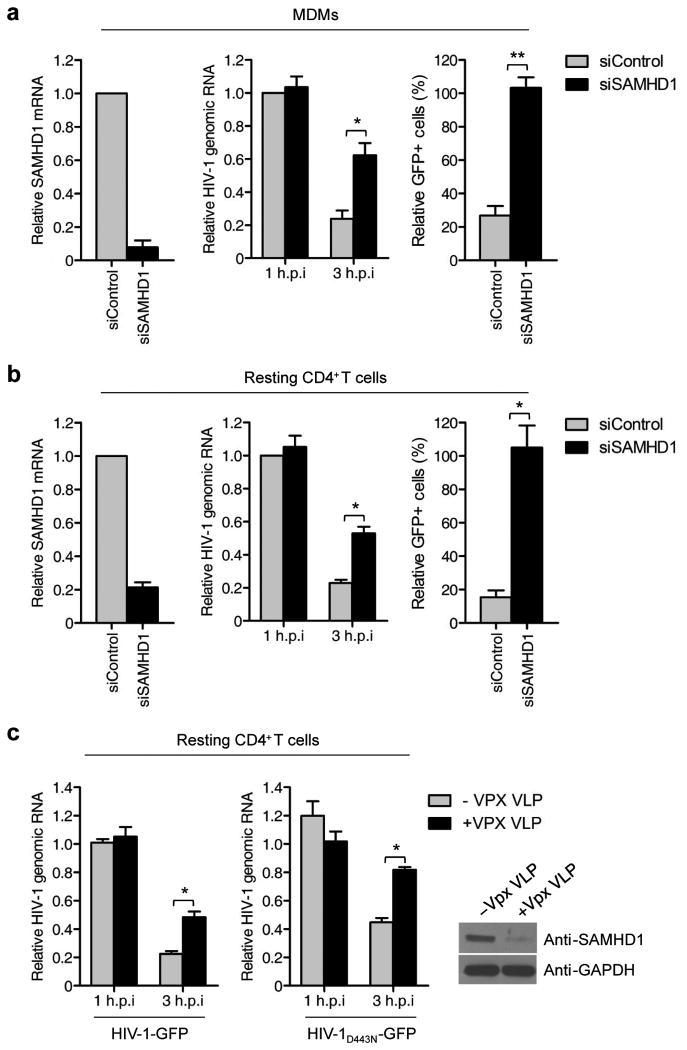
SAMHD1 degrades HIV-1 RNA in primary human MDMs and CD4+ T cells. (a) CD14+ monocytes isolated from three donors were differentiated into MDMs. MDMs were transfected with siRNA to SAMHD1 or control siRNA and then infected with 100 ng of HIV-1-GFP. The efficiency of SAMHD1 knockdown was evaluated by qRT-PCR (left). Total viral RNA was quantified at the indicated times post-infection by qRT-PCR using gfp-specific primers. The data were normalized to an internal β-actin (middle). Quantification of GFP-positive cells by flow cytometry of MDMs (right). (b) Post-activated resting CD4+ T cells from three donors were transfected with siRNA to SAMHD1 or control siRNA and then infected with 100 ng of HIV-1-GFP. The efficiency of SAMHD1 knockdown (left), HIV-1 RNA levels (middle) and HIV-1 infectivity (right) were analyzed as in a. (c) Effect of Vpx treatment on the HIV-1 RNA stability in resting CD4+ T cells. Resting CD4+ T cells were challenged with HIV-1-GFP or HIV-1-GFPD443N in the presence of Vpx VLP. HIV-1 RNA levels were quantified as in a. In a-c, data are presented as the mean ± s.d. of three experiments. (* and ** indicate significant differences compared with siControl or –Vpx VLP control at P < 0.05 and P < 0.001, respectively, using the two-tailed Student's t test).
The phosphorylation of SAMHD1 at T592 causes the loss of HIV-1 restriction without decreasing cellular dNTP levels6-8. We tested whether the phosphorylation of SAMHD1 affects its RNase activity in HIV-1 restriction. In PMA-treated U937 cells where SAMHD1WT is not phosphorylated on T5926,7, SAMHD1WT blocked HIV-1 infection. In contrast, SAMHD1T592D containing the phosphomimetic D residue was unable to inhibit HIV-1 infection (Fig. 4a), confirming previous results6. Notably, the stability of HIV-1 RNA was enhanced by three to four-fold in cells expressing SAMHD1T592D compared with cells expressing SAMHD1WT (Fig. 4b). In contrast to this in vivo observation, the recombinant SAMHD1T592D protein was as effective as the SAMHD1WT and the nonphosphorable SAMHD1T592V in degrading ssRNA substrates in vitro (Fig. 4c). RIP analysis revealed that SAMHD1WT and SAMHD1T592D bound to HIV-1 genomic RNA with similar affinities, indicating that phosphorylation of SAMHD1 T592 does not interfere with substrate binding (Fig. 4d). The discrepancy between the in vivo and in vitro RNase activities suggests that the phosphorylation of SAMHD1 might promote the recruitment of cellular factors that negatively regulate its RNase activity or interfere with the interaction of SAMHD1 with unidentified cofactors. Our results reveal that the phosphorylation of SAMHD1 at T592 is a mechanism that negatively regulates its RNase activity in vivo and impedes HIV-1 restriction.

(a–c) U937 cells stably expressing an empty vector (mock) or Flag-tagged SAMHD1 variants were treated with PMA and infected with 100 ng of pLaiΔenvGFP3. (a) Flow cytometric analysis 48 hpi to measure the percentage of GFP-positive cells. The percentage of GFP-positive cells was calculated relative to the number of GFP-positive mock-transfected cells (right). Western blot analysis of cell extracts using the anti–Flag antibody. GAPDH was used as a loading control. (b) HIV-1 genomic RNA content was quantified by qRT-PCR using HIV-1 gfp-specific primers. The data were normalized to an internal β-actin. (c) Recombinant SAMHD1T592V and SAMHD1T592D proteins were purified from E. coli. The RNase activity assay was performed as described in Fig. 1 using 5′-end 32P labeled 20-mer ssRNA substrates. (d) Effect of SAMHD1 T592 phosphorylation on substrate binding. RIP analysis was performed as described in Fig. 2f, except that cell lysates were immunoprecipitated using an anti-Flag antibody. In a, b, and d, data are presented as the mean ± s.d. of triplicate experiments. (* and ** indicate significant differences compared with the mock-transfected control at P < 0.05 and P < 0.001, respectively, using the two-tailed Student's t test). e. Model for anti-HIV-1 activity mediated by SAMHD1 RNase function.
The crystal structure of SAMHD1 reveals that this protein is dimeric and indicates a structural basis for allosteric activation of catalytic activity against dNTPs by dGTP binding11. By contrast, recent studies have demonstrated that SAMHD1 oligomerizes in a dGTP-dependent manner up to a tetramer and that the tetramerization of SAMHD1 is required for its dNTPase and anti-HIV-1 activities22-24. Notably, SAMHD1 with a mutation at the allosteric dGTP-binding site D137 did not undergo oligomerization and was mostly monomeric in the presence of dGTP22. The mutation of D137 (SAMHD1D137N) abolishes SAMHD1 dNTPase but not RNase activity (Fig. 1), indicating that unlike the dNTPase activity, the tetramerization of SAMHD1 is not necessary for its RNase activity. Based on previous data and our results, we propose that SAMHD1 is a dual-function enzyme whose mutually exclusive and predominant activities depend on the dGTP pool. At low dGTP levels, SAMHD1 exists as monomers or dimers that harbor active RNase but not dNTPase activity, enabling HIV-1 restriction through the cleavage of HIV-1 RNA. At high dGTP levels, SAMHD1 tetramerizes, yielding an enzyme with an inactive RNase but active dNTPase function (Fig. 4e). An alternative but not mutually exclusive possibility is that the ssRNA and dNTP substrates might compete with each other for a common active site on the enzyme. dGTP is both a substrate and an activator of the SAMHD1 dNTPase function11,12; this model is consistent with the observations that high levels of dGTP impair the binding of SAMHD1 to nucleic acid substrates in vitro6,16, that the switch from a processive to distributive degradation of ssRNA in dGTP-dependent manner (Fig. 1e) is indicative of competitive inhibition of ssRNA-binding by dGTP, and that mutations in the catalytic site abolish both RNase and dNTPase activities (Fig. 1). In this respect, the partial alleviation of SAMHD1-mediated HIV-1 restriction by the addition of exogenous dNs3 might be an indirect consequence of impaired RNA substrate binding by increased dGTP levels. These two hypotheses may also explain why SAMHD1 blocks HIV-1 infection only in non-cycling cells, such as DCs and macrophages with low dNTP levels1, but not in cycling cells with high dNTP levels4,6,25. Another explanation might be that in non-cycling cells, dNTP limitation would slow down reverse transcription, and this could allow a sufficient time window for the action of SAMHD1 RNase against HIV-1 RNA. The identification of SAMHD1 as an RNase suggests that SAMHD1 is involved in nucleic acid metabolism related to the prevention of an innate immune response; therefore, our results provide insight into the mechanisms underlying HIV-1 restriction and the pathogenesis of AGS.
Online Methods
Ethics statement
Adult blood samples were anonymously provided by the Blood Center of the Korean Red Cross, Seoul, under the approval of the Institutional Review Board of Korean Red Cross with consent for research use. Written informed consent was obtained from the blood donors with the approval of the Ethics Committee of the Korean Red Cross. Experiments involving human blood were approved by the Institutional Review Board at Seoul National University (SNUIRB No. E1304-001-023).
Plasmids
Full-length human SAMHD1 was amplified by PCR from cDNA generated by the reverse transcription of RNA from HeLa cells, and the PCR product was inserted into a pGEX-4T-1 vector and a pMSCV-puro vector. The SAMHD1D207N, SAMHD1D311A, SAMHD1D137N and SAMHD1Q548A mutants were generated using the nPfu-forte DNA Polymerase Kit (Enzynomics). Retroviral vectors encoding the wild-type or T592 phosphorylation variants SAMHD1 were described previously6. HIV-1-GFP, pLaiΔenvGFP3, and HCMV-VSV-G were described previously27.
Protein expression and purification
The GST-tagged, full-length, human SAMHD1 proteins (GST-SAMHD1) were expressed in E. coli Rosetta (λDE3) (Novagen). The Rosetta cells were grown in Terrific broth containing ampicillin (100 μg ml-1) at 37 °C to an optical density (OD)600 of 2.0, and the cells were rapidly cooled on ice to 16 °C. After induction with 0.1 mM isopropyl-β-D-thiogalactopyranoside (IPTG; Ducheba), the cells were allowed to grow for 16 h at 16 °C. The E. coli pellet containing the GST fusion protein was lysed with PBS, and the protein was purified using glutathione-Sepharose column chromatography, as previously described 28.
Immunoprecipitation for in vitro nuclease assay
Cell pellets derived from the stable U937 cell lines expressing mock and HA-tagged SAMHD1 were resuspended in lysis buffer (25 mM Tris-HCl, pH 7.5, 100 mM KCl, 1 mM DTT, 2 mM EDTA, 0.5 mM PMSF, 0.05% NP-40) containing an RNase inhibitor and were incubated for 30 min on ice, sonicated, and centrifuged at 13,000 × g at 4 °C for 30 min. Monoclonal mouse antibody to HA coupled to protein A–agarose beads (Sigma) was added, and precipitates were washed twice with buffer A (500 mM NaCl, 10 mM Tris-HCl, pH 7.5, 0.05% NP-40, RNase inhibitor) and twice with buffer B (150 mM NaCl, 10 mM Tris-HCl, pH 7.5, 0.05% NP-40, RNase inhibitor). Cell extracts were incubated with the antibody-coupled beads for 1 h at 4 °C. Immunoprecipitates were washed five times with buffer B followed by elution with HA-peptide.
Preparation of DNA and RNA substrates
Fragments of the HIV-1 5′ LTR, gag, and 3′ LTR were transcribed in vitro using T7 RNA polymerase (Enzynomics). The transcripts were purified using denaturing polyacrylamide gel electrophoresis (PAGE; 5% polyacrylamide, 8 M urea). Synthetic DNA and RNA oligonucleotides were 5′-end labeled with 32P using T4 polynucleotide kinase and [γ-32P]ATP. The nucleotides were precipitated by the addition of 3 M ammonium acetate and 100% (vol/vol) ethanol. The nucleotides pellets were washed with 75% ethanol, dried and resuspended in 10 mM Tris-Cl (pH 7.8) containing 1 mM EDTA. The synthetic dsDNAs and dsRNAs (Supplementary Table 1) were prepared by annealing the oligonucleotides.
In vitro nuclease assay
Assays were performed in 20-μl reaction mixtures containing PBS supplemented with 5 mM MgCl2, 2 mM DTT, 10% glycerol, 0.01% NP-40, 32P-labeled nucleic acid substrates, and purified recombinant proteins (150 nM) or immunoprecipitated proteins at 37 °C for the indicated times. The reactions were stopped by the addition of an equal volume of formamide loading buffer and then boiled. The products were separated in 15% polyacrylamide gels containing 8 M urea and buffered with 0.5 × Tris-borate-EDTA (TBE) and then analyzed by autoradiography using a phosphorimager (BAS2500, Fujifilm).
dGTP-triphosphohydrolase assay
An enzymatic assay based on thin layer chromatography was performed as described previously3. The purified recombinant protein (1 μM) was incubated in 50 mM Tris-HCl (pH 8.0), 20 mM KCl, 5 mM MgCl2, 0.1 μCi [α-32P]dGTP, and 200 μM cold dGTP for 3 h at 37 °C. The reactions were stopped by heat-inactivation at 70 °C for 10 min. The reaction mixtures were spotted along with dGMP, dGDP, and dGTP standards onto polyethyleneimine (PEI)-cellulose plates (Sigma-Aldrich) and subsequently separated using a mobile phase of 1.2 M LiCl. After separation, the α-32P-labeled reaction products were visualized using a phosphorimager, and the migration indicators were detected by UV-C (254 nm).
Cells and reagents
U937 and THP1 cells were cultured in RPMI 1640 supplemented with 10% fetal bovine serum (Hyclone), 2 mM GlutaMAX-I, 100 U ml-1 penicillin, and 100 μg ml-1 streptomycin (Invitrogen). 293T cells and Phoenix Ampho cells were cultured in DMEM supplemented as described for RPMI 1640. Stable U937 cells expressing SAMHD1 proteins were obtained by retroviral infection. The pMSCV-puro construct was used to transfect Phoenix Ampho cells using the calcium phosphate method. Two days later, the retrovirus-containing supernatant was used to transduce U937 cells by spin infection with 8 μg ml-1 polybrene. The transduced cells were selected in 1 μg ml-1 puromycin. Peripheral blood mononuclear cells (PBMCs) were obtained from human blood by density gradient centrifugation using Ficoll-Paque Plus™ (Amersham Healthcare). Monocytes were isolated from the PBMCs using a magnetic bead-based positive selection kit (IMag™) and anti-human CD14 beads (cat. 557769, BD Biosciences). This procedure routinely yielded over 90% pure CD14-positive cells, as verified by flow cytometry. MDMs were obtained from monocytes by culturing the cells for 3 d with GM-CSF (10 ng ml-1) and M-CSF (20 ng ml-1). The differentiation state of CD14+ cells was analyzed by monitoring the differentiation markers CD71 (cat. 555536, BD Biosciences), CD80 (cat. 557226, BD Biosciences), and CD86 (cat. 555657, BD Biosciences). CD4+ T cells were isolated from the PBMCs using a magnetic bead-based positive selection kit (IMag™) and anti-human CD4 beads (cat. 557767, BD Biosciences). T cells were activated, and post-activation resting T cells were obtained as previously described4. Resting CD4+ T cells (2 × 106 cells ml-1) were treated overnight with PHA-L (2 mg ml-1). The next day, the medium was exchanged and cells were incubated in RPMI medium with 20 IU ml-1 IL-2 for 3 d. Activated CD4+ T cells were electroporated with SAMHD1-specific siRNA or non-specific siRNAs. The siRNA-treated cells were cultivated in RPMI medium with gradually reducing concentrations of IL-2. The activation state of CD4+ T cells was analyzed by monitoring the surface expression of the activation markers CD25 (cat. 555434, BD Biosciences) and CD69 (cat. 555533, BD Biosciences). The experimental design for the time course analysis of MDMs and CD4+ T cells is shown in Supplementary Figs. 10 and 11.
RNA interference
siRNA targeting SAMHD1 was purchased from Dharmacon. MDMs and CD4+ T cells (2 × 106 cells) were electroporated in 100-μl reactions with siRNA (10 nM) using the Neon Transfection System (Invitrogen). Transfections with ON-TARGETplus Non-targeting pool (Dharmacon) were performed in parallel as a negative control. The MDMs and CD4+ T cells were pulsed once each for 20 ms at 2,150 and 2,100 volts, respectively.
Viruses and VLP production
293T cells were transfected with 10 μg of HIV-1-GFP, HIV-1D443N-GFP or pLaiΔenvGFP3 and 2 μg of HCMV-VSV-G using the calcium phosphate method. Vpx-containing and control VLPs were generated by the transfection of 293T cells with pSIV3+ or pSIV3+ Δvpx. Culture supernatants were collected and filtered (0.45 μm) 48 h after transfection. U937 cells (0.5 × 106 cells ml-1) were seeded in 12-well plates and differentiated for 20 h in PMA (30 ng ml-1). The cells were infected with 100 ng of pseudotyped viruses for 2 h. The cells were then washed and cultured for 48 h. Infected cells were analyzed by flow cytometry or qRT-PCR.
Quantitative real-time PCR
VSV-G-pseudotyped HIV-1-GFP-infected cells were harvested at various time points, and DNA was isolated using the QIAamp Blood DNA Minikit (Qiagen). qPCR was performed with the iCycler iQ real-time PCR detection system (BioRad) using HIV-1-specific primers: R/U5 (forward, GCCTCAATAAAGCTTGCCTTGA; reverse, TGACTAAAAGGGTCTGAGGGATCT) and U5/ψ (forward, TGTGTGCCCGTCTGTTGTGT; reverse, GAGTCCTGCGTCGAGAGATC). RNA isolation from HIV-1-GFP-infected cells was performed at various time points according to the manufacturer's instructions (Invitrogen). One microgram of RNA was treated with DNase I prior to qPCR analysis and isolated by phenol:chloroform extraction. The data were normalized to an internal control gene, MDM2 or β-actin. The purified RNA was reverse transcribed using random primers, and real-time PCR was performed with the iCycler iQ real-time PCR detection system (BioRad) using HIV-1-specific primers: gag (forward, CTAGAACGATTCGCAGTTAATCCT; reverse, CTATCCTTTGA TGCACACAATAGAG) and egfp (forward, CAACAGCCACAACGTCTATATCATG; reverse, ATGTTGTGGCGGATCTTGAAG).
RNA-Seq Analysis
Total RNA was extracted from mock- and SAMHD1-expressing U937 cells uninfected or infected with HIV-1 using TRIzol reagent (Invitrogen) by manufacturer's instruction followed by addition of spike-in poly(A)-tailed RNA (GeneChip Eukaryotic Poly-A RNA control Kit, Affymetrix) to reduce background noise and allow comparison between samples. After ribosomal RNA depletion using Epicentre's Ribo-Zero™ Kits, RNA was fragmented using RNA fragmentation reagent (Ambion). After dephosphorylation by Antarctic phosphatase (NEB), 5′ end of fragmented and dephosphorylated RNA was phospho-labeled using [γ-32P]ATP and T4 polynucleotide kinase (TaKaRa). 35–60 nucleotide RNA was extracted in a polyacrylamide, 7 M urea, TBE gel. 3′ adaptor (5′/rApp/TGGAATTCTCGGGTGCCAAGG/ddC/-3′, IDT) was ligated using T4 RNA ligase truncated K227Q (NEB) followed by ligation of 5′ adaptor (5′ Solexa linker, 100-M; 5′rGrUrUrCrArGrArGrUrUrCrUrArCrArGrUrCrCrGrArCrGrA rUrC-3′, IDT) using T4 RNA ligase (TaKaRa). The 5′ and 3′ adaptor-ligated RNA was reverse transcribed using RNA RT Primer (RTP; 5′GCCTTGGCACCCGAGAATTCCA-3′, IDT) and SuperScript™ III reverse transcriptase (Invitrogen). Each sample was amplified by PCR using Phusion polymerase (Thermo Scientific), 5′ end Illumina RNA PCR Primer (RP1) and 3′ end Illumina RNA PCR Primer (Index 1–12). RNA-Seq library was sequenced with 51-bp single-end sequencing in an Illumina HiSeq2500 sequencer. Illumina standard pipeline and software-CASAVA were employed for processing of raw imaging, base calling and generating FASTQ sequence reads. Before aligning reads to the reference sequences, we filtered low quality reads, adapter-containing sequences, and artifact reads using in house software and FASTX-Toolkit (FASTQ/A short-reads pre-processing tools, hannonlab.cshl.edu/fastx toolkit). The read sequences were then aligned to the UCSC hg19 human reference genome and HIV-1 NL4-3 genome using bowtie2-2.1.029. The abundance of transcripts was measured as the score of RPKM (Reads Per Kilobase of exon model per Million mapped reads). We applied spike-in normalization to all 12 samples to reduce variations across samples.
RNA immunoprecipitation (RIP)
The RNA immunoprecipitation protocol19 was adapted to analyze the interactions between SAMHD1 and HIV-1-GFP genomic RNA. HIV-1-GFP-infected cells were cross-linked by 1% formaldehyde for 10 min at room temperature. The crosslinking reaction was stopped by addition of glycine (1 M, pH 7.0) to a final concentration of 0.25 M followed by incubation at room temperature for 5 min. The cells were washed with ice-cold PBS and resuspended in RIPA buffer (50 mM Tris-HCl, pH 7.4, 1% NP40, 0.5% sodium deoxycholate, 0.05% SDS, 1 mM EDTA, 150 mM NaCl) containing protease inhibitors and an RNase inhibitor. The cell suspension was sonicated and centrifuged for 10 min at 9,000 × g, and the resulting supernatant was pre-cleared by incubation with protein G-agarose beads. The pre-cleared supernatant was incubated with anti-HA (A2095, Sigma) or anti-Flag antibody-conjugated beads (A2220, Sigma) for 2 h at 4 °C. The beads were washed with RIPA buffer and resuspended with reversal buffer (50 mM Tris-HCl, pH 7.0, 5 mM EDTA, 10 mM DTT, 1% SDS) followed by an incubation for 45 min at 70 °C to reverse the crosslinks. The immunoprecipitated RNAs were isolated according to the manufacturer's protocol (Invitrogen).
Cell fractionation
For nuclear-cytoplasmic fractionation, monocytes and MDMs were washed in cold PBS and resuspended with lysis buffer (10 mM HEPES, pH 7.9, 0.1 mM EDTA, 2 mM MgCl2, 0.1 mM EGTA, 10 mM KCl, 0.1 mM DTT, and 0.5 mM PMSF). NP-40 was added to obtain a final concentration of 1% and mixed by gentle inversion. The cytoplasmic extracts were separated from intact nuclei by low-speed centrifugation at 4 °C and 1400 × g for 30 s. The nuclear pellet was resuspended in buffer (20 mM HEPES, pH 7.9, 0.1 mM EDTA, 0.1 mM EGTA, 0.4 M NaCl, 1 mM DTT, and 1 mM PMSF). The nuclear and cytoplasmic fractions were analyzed by immunoblotting.
Supplementary Material
Supplementary Figure
Supplementary Table 1: Synthetic nucleic acids used in nuclease assays
Supplementary Table 2: HIV-1 specific primers used in qRT-PCR analysis
Supplementary Figure 1: Purification of recombinant SAMHD1 proteins
Supplementary Figure 2: Characterization of SAMHD1 RNase activity
Supplementary Figure 3: SAMHD1 degrades in vitro-transcribed HIV-1 RNAs
Supplementary Figure 4: SAMHD1 has ssRNA-specific nuclease activity
Supplementary Figure 5: Metal specificity of SAMHD1
Supplementary Figure 6: Correlation of the presence of the SAMHD1WT protein with RNase activity
Supplementary Figure 7: Expression level of SAMHD1 variants in U937 cells
Supplementary Figure 8: SAMHD1 RNase functions at the early step of HIV-1 reverse transcription
Supplementary Figure 9: SAMHD1 degrades multiple regions of the HIV-1 genomic RNA during infection
Supplementary Figure 10: Differentiation of monocytes into MDM
Supplementary Figure 11: Activation state of CD4+ T cells
Supplementary Figure 12: Knockdown of SAMHD1 in MDMs and resting CD4+ T cells increases HIV-1 infectivity
Supplementary Figure 13: Cellular distribution of SAMHD1 in both the cytoplasm and nucleus
Acknowledgments
We are grateful to the members of our laboratory for discussion and technical help. We thank D. Littman, N. Manel and A. Cimarelli for reagents. This work was supported by the US National Institutes of Health (R01 AI087390 and R21 AI102824 to F.D.-G. and GM104198 and AI049781 to B.K.), the Korean Institute for Basic Science (EM1402 to D.B.), the Korean Basic Science Research Program (2011-0014523 to D.B.), the Korean Creative Research Initiative Program (Research Center for Antigen Presentation, 2006-0050689 to K.A.) and BK21 plus fellowship to J.C., S.K., and M.S. from the National Research Foundation (NRF) grant funded by the Ministry of Education, Science, and Technology (MEST) of Korea.
Footnotes
Author Contributions: J.R. C.O. S-C.K, and K.A. designed the study and wrote the manuscript. J.R., C.O., J.C., S-C.K. S-Y. K. M.S., and J.H. performed the experiments and analyses. J.K., D.S., B.K., D.B., and C.Y. analyzed the data. T.W., A.B.-N. and F.D.-G. provided materials. All authors discussed the data.
References
Full text links
Read article at publisher's site: https://doi.org/10.1038/nm.3626
Read article for free, from open access legal sources, via Unpaywall:
https://europepmc.org/articles/pmc4318684?pdf=render
Citations & impact
Impact metrics
Citations of article over time
Alternative metrics
Article citations
Acetylation of SAMHD1 at lysine 580 is crucial for blocking HIV-1 infection.
mBio, 15(9):e0195824, 20 Aug 2024
Cited by: 0 articles | PMID: 39162568 | PMCID: PMC11389391
Interactions between HIV proteins and host restriction factors: implications for potential therapeutic intervention in HIV infection.
Front Immunol, 15:1390650, 16 Aug 2024
Cited by: 0 articles | PMID: 39221250 | PMCID: PMC11361988
Review Free full text in Europe PMC
Unveiling the Connection: Viral Infections and Genes in dNTP Metabolism.
Viruses, 16(9):1412, 03 Sep 2024
Cited by: 1 article | PMID: 39339888 | PMCID: PMC11437409
Review Free full text in Europe PMC
Human papillomavirus 16 replication converts SAMHD1 into a homologous recombination factor and promotes its recruitment to replicating viral DNA.
J Virol, 98(9):e0082624, 28 Aug 2024
Cited by: 1 article | PMID: 39194246 | PMCID: PMC11406955
TMEFF1 is a neuron-specific restriction factor for herpes simplex virus.
Nature, 632(8024):383-389, 24 Jul 2024
Cited by: 0 articles | PMID: 39048823
Go to all (193) article citations
Other citations
Wikipedia
Data
Data behind the article
This data has been text mined from the article, or deposited into data resources.
BioStudies: supplemental material and supporting data
Similar Articles
To arrive at the top five similar articles we use a word-weighted algorithm to compare words from the Title and Abstract of each citation.
A Highly Active Isoform of Lentivirus Restriction Factor SAMHD1 in Mouse.
J Biol Chem, 292(3):1068-1080, 05 Dec 2016
Cited by: 14 articles | PMID: 27920203 | PMCID: PMC5247641
A Cyclin-Binding Motif in Human SAMHD1 Is Required for Its HIV-1 Restriction, dNTPase Activity, Tetramer Formation, and Efficient Phosphorylation.
J Virol, 92(6):e01787-17, 26 Feb 2018
Cited by: 12 articles | PMID: 29321329 | PMCID: PMC5827405
A new activity for SAMHD1 in HIV restriction.
Nat Med, 20(8):808-809, 01 Aug 2014
Cited by: 6 articles | PMID: 25100520
SAMHD1 host restriction factor: a link with innate immune sensing of retrovirus infection.
J Mol Biol, 425(24):4981-4994, 23 Oct 2013
Cited by: 42 articles | PMID: 24161438
Review
Funding
Funders who supported this work.
NIAID NIH HHS (4)
Grant ID: R56 AI049781
Grant ID: R21 AI102824
Grant ID: R01 AI049781
Grant ID: R01 AI087390
NIGMS NIH HHS (1)
Grant ID: R01 GM104198