Abstract
Free full text

Regulation of Immune Responses by Extracellular Vesicles
Abstract
Extracellular vesicles (EVs) including exosomes, are small membrane vesicles derived from multivesicular bodies or from the plasma membrane. Most, if not all, cell types release EVs that then enter the bodily fluids. These vesicles contain a subset of proteins, lipids and nucleic acids that are derived from the parent cell. It is postulated that EVs have important roles in intercellular communication, both locally and systemically, by transferring their contents, including protein, lipids and RNAs, between cells. EVs are involved in numerous physiological processes, and vesicles from both non-immune and immune cells have important roles in immune regulation. Moreover, EV-based therapeutics are being developed and tested clinically for treatment of inflammatory and autoimmune diseases and cancer. Given the tremendous therapeutic potential of EVs this review focuses on the role of EVs in modulating immune responses and the therapeutic applications.
1. Introduction
Almost all cells release different types of membrane microvesicles and nanovesicles, which have a variety of important physiological effects. Microvesicles differ from nanovesicles mainly by their size and mechanism of generation1–4. Microvesicles are released from the plasma membrane by shedding or budding, are usually larger than 0.2 µm in size and have been referred to as microparticles or ectosomes. By contrast, nanovesicles including exosomes are between 30–100 nm in diameter, characterized by an endocytic origin and formed by the reverse budding of the peripheral membrane of multi-vesicular bodies (MVBs) or late endosomes (BOX 1). However, certain nanovesicles appear to be derived from the plasma membrane5. The protein content of different types of EVs largely reflect that of the parent cells and are enriched in certain molecules, including adhesion molecules, membrane trafficking molecules, cytoskeleton molecules, heat-shock proteins, cytoplasmic enzymes, signal transduction proteins, cytokines, chemokines, proteinases and cell-specific antigens (Ags). Moreover, EVs contain messenger RNA (mRNAs), non-coding RNA (ncRNAs) including miRNAs and even extra-chromosomal DNA such as amplified c-Myc6. Almost all cell types release EVs that are found in plasma as well as other bodily fluids including breast milk, semen, saliva, urine and sputum. EVs participate in important biological functions, acting as a mode of communication between cells. This intercellular communication can be conferred by mediators expressed on the surface of the EVs or transported in its lumen.
EVs produced by both immune and non-immune cells have an important role in the regulation of immunity. They can mediate immune stimulation or suppression and drive inflammatory, autoimmune and infectious disease pathology. Thus, EVs have the potential to be used as therapeutic agents for modulating the immune system. In this review, we focus on the biology of the 30–100 nm EVs known as exosomes and the mechanisms through which they regulate the immune response. Moreover, the applications of these EVs for the treatment of inflammatory and autoimmune diseases as well as cancer will be discussed.
Biogenesis and traffic of exosomes
Exosomes are generated by inward budding of the endosomal membrane. The resulting invaginations are pinched off and released as intra-luminal vesicles (ILVs) inside the endosomes, which are then termed MVBs (Fig. 1)1,3. MVBs can then follow either the secretory or lysosomal pathways. In the secretory pathway, MVBs fuse with the cell membrane, which results in the release of ILVs as exosomes and incorporation of the peripheral membrane of the MVBs into the plasma membrane. In the lysosomal pathway, MVBs fuse with lysosomes releasing ILVs in the lysosomal lumen for degradation.
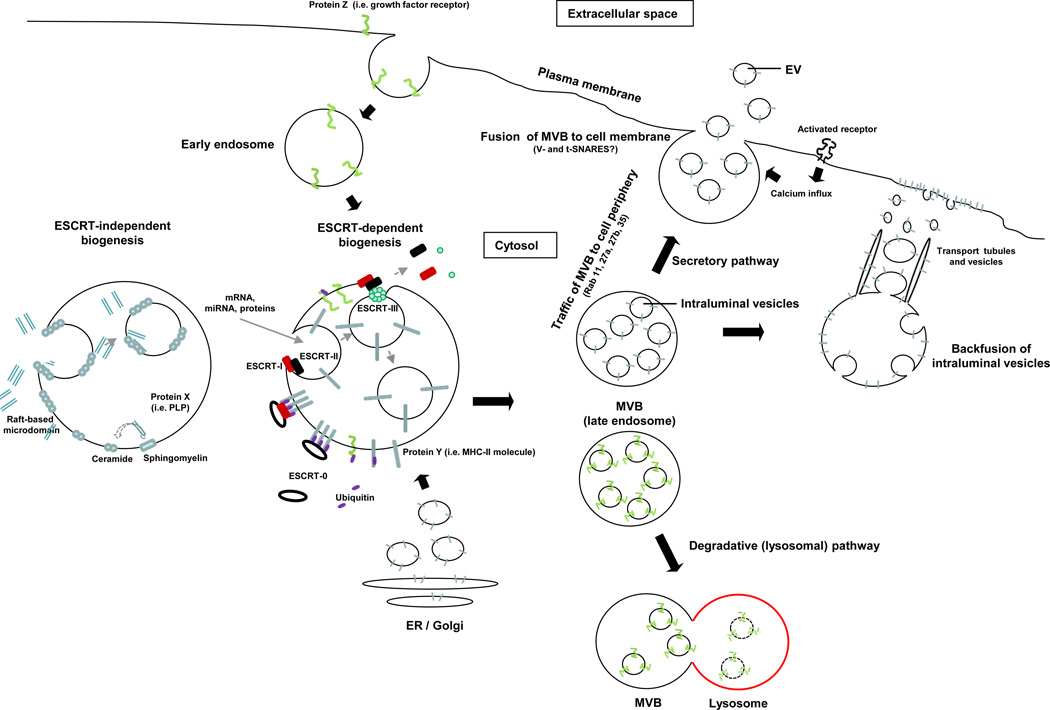
EVs are generated as ILVs in MVBs by ESCRT-dependent or –independent mechanisms. Proteins transported from the Golgi (i.e. MHC Class-II molecules), or internalized from the cell surface (i.e. activated growth factor receptors) are ubiquitylated on their cytosolic domains. However, not all proteins such as MHC Class-II required ubiquitinylation for targeting to vesicles. The ESCRT-0 complex recognizes the ubiquitylated proteins on the cytosolic side of the endosome / MVB membrane, segregates the proteins into microdomains, and binds the ESCRT-I complex, which in turn recruits ESCRT–II subunits. ESCRT-I and –II initiate the reverse budding of the nascent ILVs within MVBs. At this time, cytosolic RNAs and proteins have direct access into the interior of the forming vesicles. Next, the ESCRT-II complex recruits ESCRT-III subunits inside the neck of the nascent ILVs, which results in their cleavage into free vesicles. The free ubiquitin molecules and ESCRT subunits are released into the cytosol for recycling. Certain proteins (i.e. the proteolipid protein, PLP) are sorted into ILVs independently of the ESCRT machinery through raft-based microdomains rich in sphingolipids, from which ceramides are formed by sphingomyelinases. Ceramide induces coalescence of the microdomains and triggers ILV formation. The dashed line indicates that the role of ceramide in ILV formation is still controversial. The MVBs then follow either the secretory or degradative pathway. In the former, MVBs traffic to the cell periphery and fuse with the cell membrane, releasing the ILVs (now termed EV) constitutively, or following activation of surface receptors that trigger calcium influx. In the degradative route, MVBs released the ILVs into lysosomes. The lysosomal pathway is critical for limiting signaling of activated growth factor receptors. It is likely that differences in the MVBs confer the route of traffic.
The generation of exosomes requires the sorting of the exosome-targeted proteins and lipids into the endosomal membrane, delivery of the exosome cargo into nascent ILVs, and excision of ILVs. Monoubiquitylation of cytosolic domains of transmembrane proteins internalized from the cell surface of transported from the trans-Golgi network functions as a sorting signal to direct them to ILVs (Fig. 1). The ubiquitylated proteins are captured by the endosomal sorting complex for transport (ESCRT) machinery7, 8. MVBs can still be generated in the absence of key subunits of all ESCRTs, indicating the existence of alternative mechanisms of exosome biogenesis9. Also, sorting of certain proteins, such as MHC Class II molecules, into ILVs is independent of ubiquitination. In oligodendrocytes, sorting of proteolipid protein into ILVs is ESCRT independent10 (Fig 1). Instead, it requires segregation in sphingolipid-containing lipid rafts in the MVB membrane. Sphingomyelinases then act on the sphingolipids to release ceramide, which is crucial for ILV budding10. Indeed, exosomes are enriched in sphingolipids, ceramide and the lipid raft component cholesterol10.
Stoorvogel and colleagues have postulated the existence of two pools of MVBs, one that is high in tetraspanin-enriched microdomains and associated lipids (cholesterol, sphingomyelin, ganglisode GM3), detergent-resistant, and lysobisphosphatidic acid-low and follows the exosome secretory pathway; and another that is cholesterol-low and lysobisphosphatidic acid-high and targeted to the lysosomal pathway11, 12. Indeed, these authors demonstrated that immature dendritic cells (DCs) sort MHC molecules in ILVs in MVBs for lysosomal degradation, whereas mature DCs sort MHC molecules into ILVs in MVBs enriched in the tetraspanin CD9 and targeted for secretion11, 12.
DCs and B cells increase exosome release following cognate T-cell interactions11, 13, 14, T cells secrete EVs upon T cell receptor (TCR) activation15, and mast cells augment EV release following FcεRI cross-linking or incubation with calcium ionophores16. In tumor cells, genotoxic stress upregulates expression of the tumor suppressor p53, which indirectly augments EV secretion17.
The mechanisms by which MVBs traffic to the cell periphery, fuse with the cell membrane and release their ILV cargo, require the coordinated action of the cytoskeleton, molecular motors and vesicle fusion machinery (Fig. 1). Similar to most intracellular transport pathways, MVB trafficking is controlled by the Rab family of small GTPases. Knock down of Rab2b, 9a, 5a, 27a or 27b inhibits exosome secretion in tumor cells18, 19. Rab27a functions in docking and fusion of MVBs with the cell membrane, whereas Rab27b participates in the transfer of vesicles from the Golgi to MVBs and mobilization of MVBs to the actin-rich cortex under the plasma membrane18. In K562 cells, Rab11 promotes the fusion of MVBs with the surface membrane in response to increased cytosolic calcium20. Although the mechanism is poorly characterized, the fusion of MVBs with the plasma membrane appears to depend on SNAREs, a superfamily of proteins that regulate fusion and target specificity in intracellular vesicle trafficking21.
Once secreted, EV bind neighboring cells or the extracellular matrix, or traffic passively through the bloodstream or organic fluids. Certain blood-borne EV are rapidly captured by marginal zone phagocytes of the spleen, liver Kupffer cells, and DCs and macrophages in the lung22, 23. The rapid clearance of EVs by phagocytic leukocytes could represent a problem for the use of therapeutic EVs targeted to other tissues.
Extracellular vesicles in Ag presentation to T cells
Direct Ag presentation
The finding that EVs released by B-cell lines carry MHC Class-II, co-stimulatory and adhesion molecules suggested that such vesicles could directly stimulate CD4 T-cell clones23. This idea received further support with the demonstration that vaccination of mice with tumor peptide-pulsed DC exosomes primes tumor-specific CTLs and suppresses tumor growth in a T-cell dependent manner24 (see below). Since then, numerous studies have unveiled the direct effects of EVs in T-cell activation.
EVs maintain the topology of the APC of origin, exposing the extracellular domain of MHC molecules at the vesicle surface. EVs released by antigen presenting cells (APCs) carry surface MHC Class-I and MHC Class-II molecules and therefore, potentially can stimulate directly CD8 and CD4 T cells, respectively. At relatively high concentrations, APC-derived EVs bearing peptide-MHC (p-MHC) complexes function as Ag-presenting vesicles for T-cell clones, lines and hybrids, and for primed T cells13, 14, 23, 25. Similarly, EVs pulsed with peptides from Epstein-Barr virus (EBV), cytomegalovirus and influenza virus have been shown to directly trigger in vitro IFNγ secretion by a small percentage of human peripheral CD8 T cells, probably memory T cells26. However, the T-cell stimulatory activity of free EVs appears to be 10–20 times less efficient than that of the parent APCs16, 24. This might explain the low capability of free APC-derived EV to stimulate in vitro naïve T cells14, 27, which require higher levels of TCR cross-linking and co-stimulation than T-cell clones, lines or hybrids, or activated/memory T cells. The weak T-cell stimulatory ability of free EVs in vitro is probably also due to their small size and vesicle dispersion caused by Brownian motion. Indeed, when APC-derived EVs are immobilized at high concentration on latex beads, or when the number of p-MHC complexes per vesicle is augmented by direct loading of the vesicles with peptide, EVs substantially increase their T-cell stimulatory capability in culture16, 28, 29.
Indirect Ag presentation through transfer to antigenic peptides to APCs
The capacity of free EVs to stimulate T cells, including naïve T cells, can be enhanced by the interaction of the vesicles with DCs16, 22, 27, 30–34. Real-time microscopy revealed that EV that are attached to cells move in a slow drifted mode, similar to the motion of integral membrane proteins that are connected to the underlying cytoskeleton, suggesting that EV bind surface receptors on target cells35. Indeed, EV harbor surface ligands that participate in binding to target cells36, 37 or extracellular matrix proteins38, 39.
The presence of certain adhesion molecules that are involved in exosome binding to DCs40, such as integrins and ICAM1 (also known as CD54), depends on the lineage and activation stage of the parent cells. Indeed, exosomes released by LPS-treated DCs (mature exosomes) bear more MHC Class-II, CD86 and ICAM1 molecules and exhibit a more potent T-cell stimulatory function than exosomes secreted by immature DCs22, 29, 31, 41. The increased stimulatory function of mature DC-derived exosomes is due, at least in part, to their higher ICAM1 content that could increase binding of the vesicles to APCs or enhance T-cell binding and/or activation during APC-T cell interaction. Indeed, DC mature exosomes transfer the ability to activate naïve T cells to non-professional APCs31. In addition, T-cell activation triggers a conformational change on the integrin LFA1 (also known as CD11a) that augments its affinity for ICAM1 on DC-derived exosomes13.
Other ligands, including milk fat globule E8 (MFGE8; also known as lactadherin), tetraspanins and externalized phosphatidylserine (PS), are constitutively present on EVs. These ligands and adhesion molecules participate directly or indirectly in the binding of EVs to DCs36, 37, 42.
For example, MFG-E8, a secreted glycoprotein, binds externalized PS on EVs 1, 43 and can function as a bridge between the vesicles and target cells by binding αVβ3/β5 integrins on DCs and macrophages. However, EVs arising from MFG-E8-deficient DCs are still capable of transferring p-MHC complexes to DCs, suggesting the existence of redundancy in the mechanisms by which EVs bind to DCs44. PS is also recognized by T-cell immunoglobulin 1 (TIM1) and TIM4 on target leukocytes45, 46. Carbohydrates on the exosome surface also participate in recognition and uptake of the vesicles by phagocytes. The lectin galectin-5 impairs the uptake of reticulocyte-derived exosomes by macrophages through binding of β galactosides on the vesicles and inhibiting their recognition by macrophage lectin receptors47.
A percentage of the acquired EVs remains on the surface of the target cell, whereas the rest is internalized by phagocytosis or macropinocytosis22, 36, 48, 49. Immature DCs internalize EVs more efficiently than mature DCs, whereas mature DCs retain more EVs on the cell surface22. Once exosomes are internalized by DCs, the exosome-borne p-MHC complexes can be degraded by the APCs and used as a source of peptide to interact indirectly with T cells22, 32 (Fig. 2). For example, exosomes released by intestinal epithelial cells and bearing HLA-DR4 molecules loaded with the human serum albumin64–76 peptide activate specific T-cell hybrids only in the presence of HLA-DR4+ DCs, suggesting that the extracellular vesicles transfer the peptide from their HLA-DR4 molecules to HLA-DR4 molecules of the DCs32. Similar results have been reported in vivo, where EVs carrying IAb loaded with IEα allopeptide triggered proliferation of specific CD4 T cells in wild-type mice, but not in MHC Class-II-deficient hosts22.
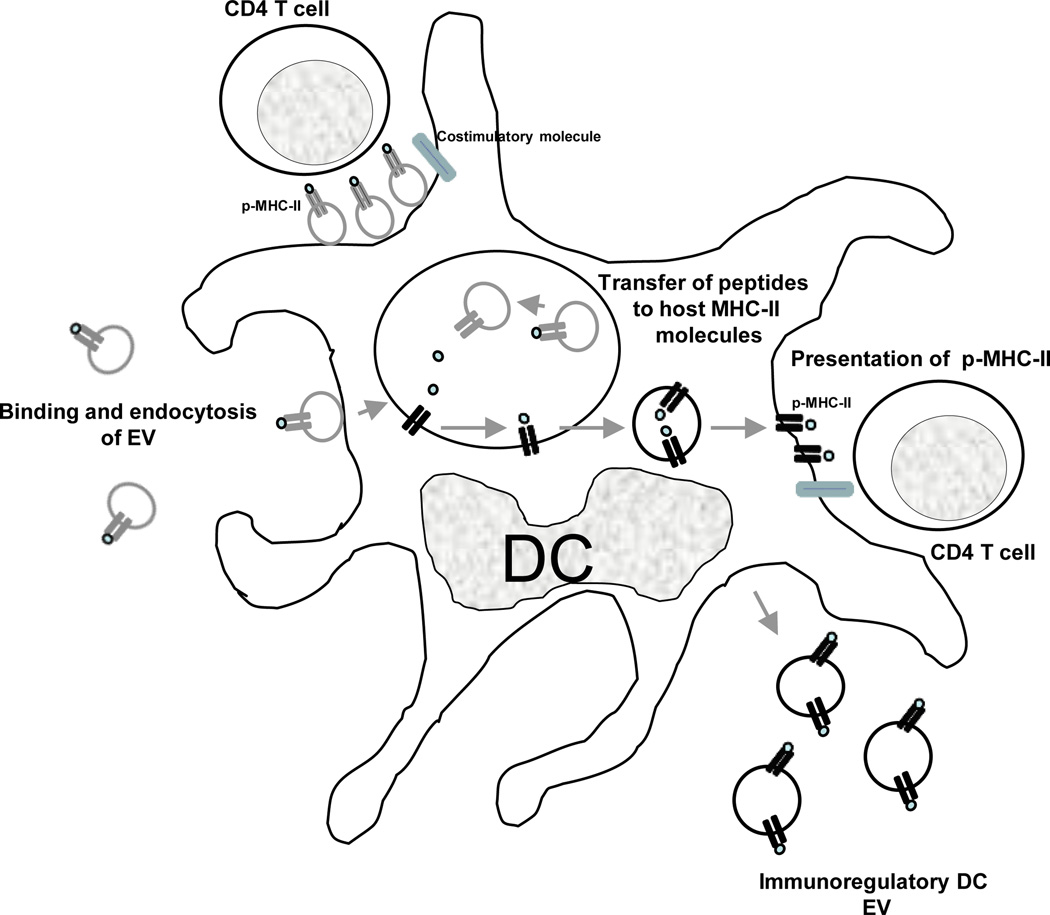
Professional APCs (i.e. DCs) present p-MHC complexes derived from captured exosomes. EVs retained on the APC surface present their p-MHC complexes directly to T cells, although the costimulatory molecules are provided by the APC. Alternatively, internalized EVs transfer their Ag-peptides to MHC molecules of the host APCs. The host MHC molecules loaded with the exosome-derived Ag-peptide are then transported to the APC surface for presentation to T cells. The APCs also release EVs able to regulate Ag-specific immune responses. Although for only MHC Class-II complexes are shown in fig. 2, a similar process occurs for exosomal MHC Class-I for regulation of CD8+ cells.
Indirect Ag presentation by cross-dressing APCs
p-MHC complexes of EVs attached (or fused) to APC surfaces can also be presented directly to T cells without the need for p-MHC reprocessing – through a mechanism known as cross-dressing (Fig. 2). The finding that optimal T-cell stimulation occurs when exosomes transfer pMHC complexes to mature DCs indicates that the acceptor APCs provide the required costimulatory molecules that are absent in the extracellular vesicles22, 27, 40. MHC Class-II (IAb)-deficient DCs incubated with exosomes bearing peptide-IAb complexes stimulate proliferation of specific CD4 T cells, demonstrating that the p-MHC complexes derived from the EVs, not the DCs. However, maximal T-cell stimulation triggered by EVs in the presence of MHC Class II-deficient DCs was less than that elicited in the presence of wild-type DCs, suggesting that part of the T-cell response was triggered by indirect presentation of the exosome-carried peptide and DC-derived IAb molecules3. Finally, there are several lines of evidence suggesting that, following allograft transplantation, cross-dressing of recipient’s APCs with donor MHC molecules could be mediated through exosome transfer50. By contrast, exosomes do not seem to participate in cross-dressing of APCs during priming of T cells after viral infections34, 51.
Extracellular vesicles as carriers of native Ags
Tumor Ags
EVs also transfer native Ags to APCs. Tumor-derived EVs containing native tumor-Ags can be efficiently taken up by DCs and the antigen processed and cross-presented to tumor-specific CTLs52, 53 (Fig. 3). Moreover, vaccination of mice with tumor-derived exosomes was shown to induce a potent CD8 T cell-mediated anti-tumor effect not only on the autologous tumor, but also against other related tumors expressing the same tumor-rejection Ag(s)52. Importantly, vaccination of mice with syngeneic or allogeneic tumor exosomes is equally effective, since cross-protection against tumors is mediated by processing of the exosome-derived tumor Ag by host DCs, independently of the MHC molecules of the vaccinated EVs52. Indeed, human tumor exosomes bearing the Mart1 tumor Ag and an irrelevant HLA haplotype promoted activation of an HLA-A2-restricted CTL clone specific for Mart-1, when EVs were taken up by HLA-A2+ DCs53. The possibility that tumor EVs efficiently deliver unknown tumor Ags to DCs for CTL cross-priming, makes exosome-based vaccines an attractive prophylactic or therapeutic approach against cancer.
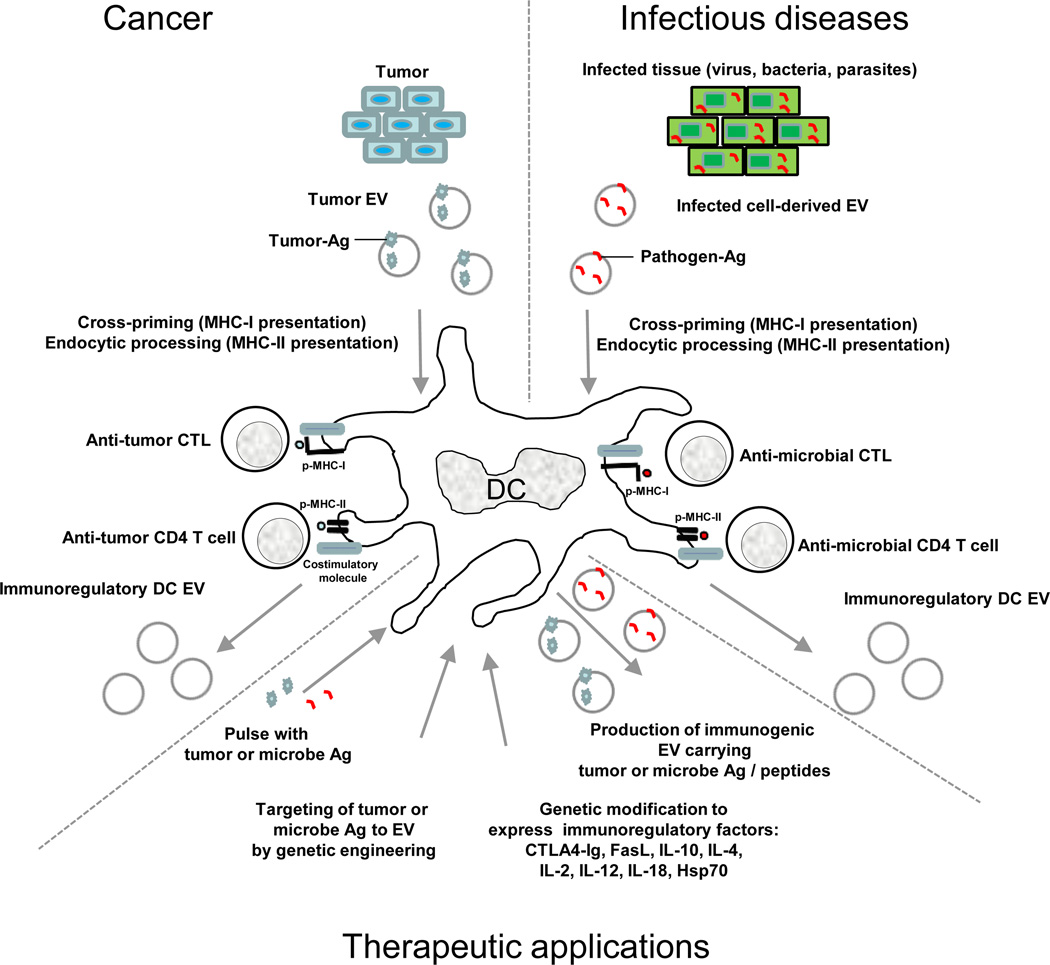
Professional APCs (i.e. DCs) process tumor- or microbe-derived Ags for presentation to CTLs or CD4 T cells. A similar approach can be employed in vitro for generation of immunogenic EVs for therapeutic applications, by pulsing the APCs with tumor- or pathogen-derived Ags, or by genetically engineered the APCs to target the desired-Ags to the exosome membrane. Similarly, the APC can be modified to express immunosuppressive or immunostimulatory cytokines or ligands, rendering the EVs released from the APCs able to suppress or stimulate Ag-specific immune responses.
Pathogenic Ags
Certain microbial components also can be present in EVs (Fig. 3). Mycobacterium bovis BCG-infected macrophages release EVs containing mycobacterial Ag that, in the presence of DCs, promote T-cell immunity in mice33. Vaccination with EVs released by Toxoplasma gondii-pulsed DCs confers protection against parasite infection in mice, suggesting that the EVs transport toxoplasma Ags54. Certain viral Ags are also targeted into the exosome pathway. T cells segregate the HIV Gag protein into plasma membrane-derived EVs5, and CMV-infected endothelial cells release EVs containing CMV gB protein that stimulate memory CD4 T cells in the presence of APCs55. EBV-infected cells release EVs carrying the oncogenic viral Ag Latent membrane protein 1 (LMP1)56. The influenza virus haemagglutinin (HA) Ag, due to its localization in raft domains, is sorted into the exosome membrane with its extracellular domain facing outward57. This incorporation of HA increases the ability of the EVs to bind acceptor cells through terminal sialic acid residues57. Indeed, oligodendrocyte-derived exosomes contain myelin oligodendrocyte glycoprotein, an auto-Ag that is implicated in multiple sclerosis49.
As exosomes originate in MVBs positioned along the endocytic route, they can transport Ags internalized by the parent cells, which could be of important for therapeutic applications58 (Fig. 3; Supplemental Table 1). Alternatively, by genetically engineering the parent cells, Ags that are not naturally present on EVs can be delivered into the vesicles59–61. Given their high immunogenic potential, Ag-associated EVs released by Ag-pulsed or genetically-engineered parent cells constitute a promising cell-free system for vaccination, in particular for peptides present in low frequency or endowed with weak immunogenicity59.
B-cell receptor Ags
B cells recognize conformational epitopes on native Ags, which are presented as immune complexes (ICs) on follicular DCs (FDCs) within B-cell follicles62. The ICs activate the complement system generating iC3b, C3dg and C3d fragments, which bind the complement receptor (CR) 1/2 (CD35/CD21) on FDCs. Multiple copies of native Ags arranged at regular intervals, together with iC3b, C3dg and C3d, are presented to B cells on FDC-derived iccosomes, which promote B-cell receptor (BCR) cross-linking and CR2-mediated B-cell activation, respectively. Increasing evidence suggests that EVs attached to FDCs could employ similar mechanisms to promote B-cell immunity.
FDCs do not synthesize MHC Class-II molecules, but can acquire pMHC-II complexes by capturing MHC-II+ EVs that are probably released by follicular B cells63. Since FDCs are non-phagocytic, the captured EVs likely remain on the cell surface for a long-term. Interestingly, FDCs synthesize MFG-E8 and express ICAM1 and CR1/2, which could participate in docking or binding of EVs through ligation to PS, CD11a, and/or C3-derived fragments62, 64. Indeed, B cells release EVs bearing C3-derived fragments as a mechanism of elimination of C3-fragments deposited on the cell membrane in physiological conditions65. Exosomes are protected from complement lysis due to their surface content of CD55 and CD59, which regulates C3b deposition and inhibits assembly of the membrane attack complex, respectively66.
After immunization of mice with exosomes from DCs loaded with OVA Ag, the generation of T-cell immunity required Ag-specific B-cell assistance29. Because B cells recognize conformational epitopes, the findings suggest that to be effective immunogens, EV must carry B-cell epitopes as native or partially-degraded Ags29. On the other hand, resting B cells increase the release of EVs upon stimulation with anti-IgM Ab plus CD40-signaling14, a condition that mimics the activation of B cells by FDCs in vivo62. EVs released by B cells undergoing activation bear MHC molecules loaded with peptides derived from the internalized native Ags. Therefore, it is likely that at the FDC surface, EVs-bearing copies of native Ags stimulate Ag-specific B cells, and EVs carrying pMHC-II complexes (released by follicular B cells undergoing activation) attract/stimulate follicular helper T cells specific for that particular pMHC complex. This scenario would lead to B-T cell interaction in an Ag-specific manner and development of B-cell immunity. B cells also augment the release of exosomes following cognate interactions with CD4 T cells14, 67. At later stages of B-cell differentiation, ex vivo experiments suggest that upon stimulation, allergen-specific B cells release exosomes that activate Th2 cells25.
Extracellular vesicles in transfer of RNAs
As well as carriers of protein Ags, EVs constitute a mechanism for the intercellular passage of genetic information in the form of RNAs, which makes tissue-targeted EVs potential delivery platforms for gene therapy (see below)2, 60, 68. EVs secreted by normal or cancer cells have been found to contain functional mRNAs and small ncRNAs, including miRNAs69–76, but generally not DNA (with the exception of amplified cMYC extra-chromosomal DNA found in certain tumor derived EVs6) or ribosomal RNAs70. The RNAs transported by EVs, termed in the case of exosomes, as exosome shuttle RNAs, are protected from degradation by RNases70. Some mRNAs and miRNAs are detected in both EVs and parent cells, whereas others are identified in either EVs or parent cells, which suggests a preferential sorting of certain RNAs sequences into EVs 70, 77.
The passage of RNAs from the lumen of the EVs into the target cells requires the release of the vesicle contents into acceptor cells (Fig. 4). This involves the docking of EVs on target cells (predominantly at cholesterol-rich microdomains) and fusion of their membranes, as demonstrated by incubating DCs with EVs labeled with a lipophilic probe78, 79. The delivery of exosome contents into the cytosol of target cells has been shown by content-mixing assays in which DCs expressing transgenic luciferase in the cytoplasm were incubated with luciferin-loaded EVs 78 (Fig. 4).
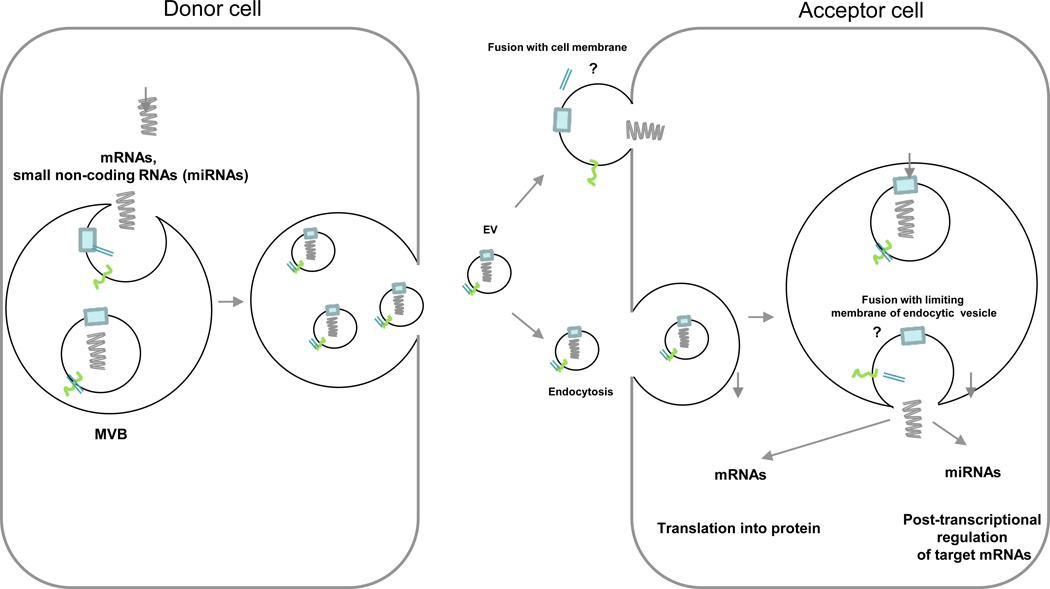
mRNAs and small non-coding RNAs, including miRNAs are transported inside the lumen of secreted EVs. Once released, the EVs are trapped by acceptor cells. Release of the vesicular RNAs into the cytosol of the target cell requires fusion of the exosome membrane with the plasma membrane or more likely with the limiting membrane of endocytic vesicles, once the EVs are internalized.
The finding that miRNAs miR-148a and miR-451 delivered by DC-derived exosomes are functional in miRNA reporter assays in acceptor DCs suggests that this phenomenon represents a means of horizontal propagation of post-transcriptional regulation among APCs78, or between APCs and other cell types (Fig. 4). Indeed, Ag-induced assembly of the immunological synapse triggers polarization of T-cell MVBs to the point of APC-T cell contact, and promotes the transfer of exosomal miR-335 that is functional in miRNA reporter assays in acceptor APCs77. EBV-infected B cells transfer exosome shuttle viral miRNAs to DCs that silence mRNA transcripts encoding immune-stimulatory molecules80.
Activation of immune responses by extracellular vesicles
Increasing evidence strongly suggests that EVs not only transfer Ags to APCs, but also signals that may promote activation of the acceptor cells into immunogenic APCs. Mast cell-derived EVs, which contain relatively high content of the heat shock proteins HSP60 and HSC70, promote DC maturation in mice58. Macrophages infected with Mycobacterium tuberculosis, Salmonella typhimurium, or Toxoplasma gondii release EVs carrying microbial Ags plus pathogen-associated molecular patterns that promote an inflammatory response by macrophages in a Toll-like receptor-dependent manner81. This intrinsic adjuvant effect, together with their vesicular nature, may explain why EVs are more efficient than soluble peptides at transferring Ag between APCs.
IL-1β, a cytokine that lacks the leader sequence needed for secretion via the classical pathway, is released by DCs and macrophages inside and EVs 82–84. How IL-1β is then released from the vesicle lumen remains unknown. Unlike IL-1β, other cytokines are transported on the EV surface. TNF superfamily members, including FasL, TRAIL and CD154 (CD40L) are sorted into the exosome membrane. CTLs, NK cells and DCs kill target cells through polarized release of FasL-carrying EVs 85–88. Tumor cells and parenchymal cells of immune-privilege tissues also secrete FasL via EVs as a mechanism of immunoescape89, 90. Mast cells release CD154-bearing EVs91 and platelets secrete vesicles that deliver CD154-CD40 signaling92. Release of TNF superfamily molecules through EVs decreases their degradation by surface proteases, augments their local concentration in the extracellular milieu, and favours their aggregation into trimers increasing their biological activity87. DC- and macrophage-derived exosomes carry enzymes that synthesize of leukotriene B4 (LTB4) and LTC493. At inflammation sites, instable TLA4 released by neutrophils could be converted into pro-inflammatory LTB4 and LTC4 by APC-derived EVs.
EVs also seem to have a role in mediating inflammatory and autoimmune diseases. For example, EVs in the serum and derived from synovial fibroblasts of patients with rheumatoid arthritis (RA) have higher levels of a membrane-bound form of TNF, a key therapeutic target in the treatment of RA, than EVs from healthy controls94. Interestingly, these TNF-positive EVs rendered activated T cells resistant to apoptosis, which could contribute to the T-cell mediated pathogenesis of RA. In addition, citrullinated proteins and the nuclear protein DEK, known to be auto-Ags in RA, were detected in EVs purified from the synovial fluids of patients with RA and juvenile arthritis, respectively95. Also, EVs found in the articular cartilage of osteoarthritis patients mediate mineral formation and destruction of articular cartilage96.
Immunosuppression by extracellular vesicles
Tolerosomes
Besides their clear roles in promoting immune responses, endogenous EVs have also been shown to have immunosuppressive effects following Ag immunization via certain routes and in tumor models. Oral administration of ovalbumin (OVA) resulted in the generation of MHC Class-II positive vesicles in the serum that could suppress OVA-specific immune responses, and were therefore termed tolerosomes97. These MHC Class-II positive circulating vesicles appear to be derived from intestinal epithelial cells that acquire Ag after feeding97. Similarly, following intradermal immunization with OVA, EVs could be isolated from plasma that suppress an OVA-specific delayed type hypersensitivity (DTH) response in the footpad98. The OVA-specific, circulating suppressive EVs were MHC Class-II positive and required both MHC Class-I and FasL for their suppressive effects. Interestingly, the number and/or activity of OVA-specific, immunosuppressive EVs in the blood peaked 14 days post immunization. Thus, it has been hypothesized that the functional relevance of blood-borne EVs is to dampen an active immune response to Ag encountered through certain immunization routes98. It is suggested that these suppressive EVs would prevent peripheral self-Ags and commonly encountered foreign Ags from causing chronic inflammation and autoimmunity. Exosomes isolated from bronchoalveolar fluid of mice previously tolerized to the allergen Ole e 1 by repetitive intranasal inoculation, prevented allergic reactions against Ole e 1 in a mouse model, suggesting that other bodily fluids also contain Ag-specific, immunosuppressive EVs99.
Tumor-derived extracellular vesicles
Tumor-derived EVs have been reported both to stimulate and suppress tumor specific and non-specific immune responses. As described above, given that the protein composition of EVs is similar to the parental cell type, tumor-derived EVs contain tumor-specific Ags. In fact, certain tumor-derived EVs are enriched for tumor Ags such melan-A, carcinoembryonic antigen (CEA) and mesothelin compared to the parental tumor cells52, 53, 100. Therefore, tumor-derived EVs have been used as a source of tumor Ags to stimulate an anti-tumor response (see below). For example, treatment of DCs with tumor-derived EVs resulted in DCs able to induce CD8 T cell-dependent anti-tumor effects in mice and in patients with malignant gliomas101.
However, despite the preclinical and clinical data suggesting that tumor EVs are a rich source of tumor Ags, there is substantial evidence that the role of tumor-derives EVs is primarily to suppress Ag-specific and non-specific anti-tumor responses. For example, tumor-derived EVs are enriched for FasL, TRAIL, or galectin-9, which can promote T-cell apoptosis89, 102, 103. Moreover, tumor-derived EVs suppress CD3-ζ chain expression in T cells and block NKG2D-dependent cytotoxicity of NK cells and CD8 T cells104.
In addition to anti-T and -NK cell effects of tumor-derived EVs numerous effects on APC function have been reported. For example, monocyte differentiation can be altered to favor the generation of myeloid-derived suppressor cells (MDSCs), implicated in inhibiting the anti-tumor immune response105. Here prostaglandin E2, TGF-β, Hsp70 and miRNAs contained in tumor-derived vesicles play important roles in driving monocytes differentiation towards MDSCs106–108. These MDSCs, in turn, induce or support the function of regulatory T cells (Tregs). In addition, tumor-derived EVs can directly affect Tregs, both enhancing their function and inhibiting their apoptosis. Tumor-derived EVs also can block maturation of DCs and macrophages in vivo and in culture through a TGF-β1-dependent mechanism109.
Given that tumor-derived EVs contain both tumor Ags and immunosuppressive mediators, it is not surprising that they are able to suppress tumor Ag-specific immune responses through modulation of both DC and macrophage function. Using OVA as a model tumor Ag, EVs derived from an OVA-expressing melanoma and containing full length OVA protein effectively suppress an OVA-specific immune response110. In addition, in tumor-bearing mice, blood-borne EVs positive for CD11b suppress tumor Ag-specific responses through a MHC Class-II dependent, but MHC Class-I independent mechanism111. These observations suggest that tumor-derived EVs initially modulate the function of CD11b positive APCs in the tumor microenvironment, which in turn release immunosuppressive MHC Class-II, CD11b positive vesicles into the circulation. How these endogenous vesicles suppress tumor Ag-specific responses is unclear, but they likely play an important role in tumor immunoescape.
Although tumor-derived EVs can suppress tumor-specific immune responses, vesicles also play a role in facilitating tumor invasion and metastasis. Tumor-derived EVs have been demonstrated to play an important role in the establishment of the pre-metastatic niche through the generation of a suitable microenvironment in distant metastatic sites19, 112–115. In addition, melanoma-derived EVs injected in peripheral tissues preferentially home to sentinel lymph nodes to prepare lymph nodes to become remote niches for metastatic tumor growth19. Taken together, it appears as if tumor-derived EVs play important roles at multiple stages of tumor pathogenesis, from suppressing the anti-tumor specific immune response to facilitating formation of the metastatic niche.
Immunosuppression by stem cell-derived extracellular vesicles
Adult stem cells, including mesenchymal stem cells (MSCs), from different sources, confer regenerative effects in animal models of disease and tissue injury and are in the phase I and II trials for limb ischemia, congestive heart failure and acute myocardial infarction116. In addition, MSCs also confer immunosuppressive effects with positive results observed in extensive clinical trials for Crohn’s and graft-versus-host diseases. However, in many of cases where therapeutic effects have been observed with MSCs, the transplanted stem cells have not been documented to persist following injection or contribute to regenerating tissue117, 118. Thus, it appears as if the predominant, immunosuppressive therapeutic effects of adult stem cells are caused through paracrine mechanisms, mediated by secreted factors including EVs. In certain cases, injection of conditioned media from MSCs has been confirmed to function as efficiently as the stem cells, implicating the secretion of immunosuppressive cytokines and other factors in conferring the anti-inflammatory effects of stem cells119–124. MSCs express a number of chemokines to attract different T-cell subsets as well as express immunosuppressive factors such as indoleamine 2,3-dioxygenase (IDO), TGF-β1, PGE-2 and IL-10118. Interestingly, analysis of MSC-derived EVs in animal models of inflammation suggests that the stem cell-derived vesicles are also immunosuppressive, likely through transfer of both RNA and proteins carried by the EVs124, 125. Thus, EVs released from MSCs, which can be rapidly isolated by methods of ultra-centrifugation and filtration, could be administered easily and safely for treatment of autoimmune and inflammatory diseases in addition to tissue regeneration. Indeed, clinical studies have been initiated to treat graft versus host disease with MSC-derived EVs.
Therapeutic applications of immunoregulatory extracellular vesicles
APC-derived extracellular vesicles
The ability of EVs, especially those derived from APCs, to regulate the immune response can be enhanced through both pharmacological and biologic treatments including gene transfer. APCs, in particular DCs, can regulate Ag-specific and non-specific immune responses both positively and negatively (Fig. 3; Supplemental Table 1). The differential immune regulation depends, in part, upon the level of expression of MHC Class-II and MHC Class-I and co-stimulatory molecules such as CD80, CD86, and PD-L1/2126. Immature DCs express a low ratio of co-stimulatory versus co-regulatory molecules on their surface and therefore are immunosuppressive. Treatment of in vitro generated DCs with immunosuppressive drugs or cytokines or genetically modified to express immunosuppressive agents (see below) renders the DCs immunosuppressive. For example, bone marrow-derived DCs treated with IL-10 or IL-4 resulted in DCs able to suppress established collagen induced arthritis (CIA) and prevent onset of hyperglycemia in NOD mice127–130. Interestingly, treatment with EVs derived from these immunosuppressive DCs generated in vitro also reversed early onset CIA as or more effectively than the parental cells130–133. Although the mechanisms of immunosuppression have not been completely elucidated, the suppressive DC-derived EVs were internalized in vivo by DCs and macrophages of the spleen, liver and draining lymph nodes. Here, it is likely that the immunosuppressive DC-derived EVs modify the behavior of endogenous immune cells such as DCs and macrophages, thereby conferring a systemic suppressive/anti-inflammatory effect. The fact that there is rapid clearance of injected EVs by phagocytic leukocytes likely increases the therapeutic efficacy of this approach. In addition, the suppressive activity of the EVs was MHC Class-II dependent in that extracellular vesicles from MHC Class-II-deficient DCs had no suppressive activity whereas MHC Class-I-deficient EVs retained their function132. Interestingly, the suppressive effect was also dependent upon the presence of Fas in the host mice and FasL in the EVs132. However, no increase in the level of T-cell apoptosis was observed following injection of EVs, suggesting a different mechanism of action for Fas/FasL.
Immunosuppressive exosomes also have been used in transplantation. Donor-specific allograft immunosuppressive was induced by co-treatment with donor DC-derived exosomes with a drug that block maturation of recipient DCs134. Similarly, treatment of recipient mice with exosomes released by immature donor DCs combined with a suboptimal dose of rapamycin before and after transplant prolonged significantly survival of cardiac allografts in a mouse model135.
The therapeutic effects of APCs and, in particular, APC-derived EVs also can be enhanced efficiently by gene transfer. Here, APCs can be modified by non-viral methods including liposomes and even more efficiently by viral gene transfer including lentiviral and adenoviral vectors. Transfer of genes encoding immune-regulatory cytokines such as IL-4, IL-10 or TGF-β1 resulted in release of APC-derived EVs that are as immunosuppressive as the parent APCs. Here, it is possible that expression of the cytokines in genetically modified APC results in more immunosuppressive APCs and thus their EVs. Alternatively, it is possible that a low level of cytokines contained within the vesicles contributes to the suppressive effects. Similarly, gene transfer of IDO to APCs resulted in the release of immunosuppressive EVs133. Although IDO could be found in the APC-derived EVs, treatment of the genetically modified APCs with an IDO inhibitor reduced the suppressive effects of the EVs133. This suggests that it is the activity of IDO in the APCs that renders the EVs immunosuppressive, but the mechanism is unclear. In addition, gene transfer of FasL to APCs results in highly immunosuppressive vesicles. Here, the presence of exogenously expressed FasL132 on the surface of the vesicles is required for immunosuppression. Similar approaches could be used to genetically modify MSCs to render the MSC-derived EVs more immunosuppressive.
Naturally occurring immunosuppressive extracellular vesicles
In addition to EVs derived from immunosuppressive APCs, there are naturally-occurring immunosuppressive EVs that could be used therapeutically. Autologous EVs isolated from plasma shortly after Ag feeding or following Ag inoculation could be used to induce Ag-specific immunosuppression97, 98. In addition, EVs isolated from the bronchoalveolar lavage fluid following exposure to specific Ags could be used to prevent Ag-specific allergic reaction99, 136. Similarly, EVs present in human breast milk and colostrum, which can increase the number of Treg cells, could be used to suppress immune responses137, 138. Finally, pregnancy has been shown to alleviate the severity of RA and multiple sclerosis. Thus, trophoblast cell EVs present in the maternal peripheral circulation could be used to inhibit T cell signaling139. The isolation and enrichment of these different types of either autologous or allogeneic vesicles have clinical utility to control auto- or allo-immunity, or inflammatory diseases. Indeed, blood based therapeutics such as platelet rich plasma (PRP) are already used routinely. In an unblinded clinical study, EVs from a blood-based therapeutic similar to PRP showed evidence of efficacy in patients with severe RA, demonstrating the feasibility of using autologous EVs therapeutically.
Enhancing immunostimulation by tumor-derived extracellular vesicles
Although tumor-derived EVs appear to be predominantly immunosuppressive, they clearly represent a source of tumor Ags. APCs pulsed with EVs derived from tumor cells cross-present the Ags to Ag-specific cytotoxic T lymphocytes. The ability of APC-treated with tumor-derived EVs to stimulate T cell responses has resulted in several clinical trials with some evidence of immunostimulation140, 141. In a clinical trial for malignant glioma, tumor exosome-loaded DCs were shown to stimulate a tumor-specific CD8+ CTL response against autologous tumor cells101. However, in general, the antitumor efficacy observed in these clinical studies has been limited, likely due to the need to render the tumor-derived EVs or the tumor exosome treated DCs more immunostimulatory. Here, different approaches including treatment of the DCs with IFN-γ142 and gene transfer can be used to enhance the immunostimulatory capacity of the tumor-derived vesicles. The ability of tumor-derived EVs to induce anti-tumor immunity has been enhanced by gene transfer of cytokines such as IL-12 or IL-2143, 144, membrane-bound tumor Ags or heat shock proteins59, 145–147. In addition, heat shock of tumor cells resulted in the release of EVs containing DC- and T-cell chemokines146, a process that could be further enhanced by genetically modifying the tumor cells. Similar approaches also can be used to elicit immune response to viral and bacterial Ags.
Several miRNAs and other ncRNAs contained within EVs likely contributing to their biological effects2. The ability to alter the RNA composition of the vesicles by either transfer to the parent cell of a specific gene or non-coding ncRNAs or by direct delivery of miRNA or siRNA to the vesicle allows for vesicles to be used as carriers of therapeutic RNAs. The feasibility of this approach has been demonstrated using tumor-derived EVs to deliver a therapeutic siRNA, but in theory, this approach could be used for a variety of EVs 68.
Concluding remarks
Almost, if not all, cells release EVs containing mRNAs, miRNAs, ncRNAs, proteins, lipids, carbohydrates and even metabolites. Thus EVs, which are similar in several aspects to enveloped viral particles, concentrate, transport and deliver molecules implicated in important biological processes. EVs from both immune and non-immune cells modulate the immune response both positively and negatively. In addition to the role of EVs in the regulation of the immune response, it is likely that EVs participate in age-related tissue degeneration, modulation of microbial infection, autoimmune and inflammatory disease pathology, and tumor initiation and progression. However, it is important to note that although EVs have been shown to exert effects on leukocytes in vitro or on innate and adaptive immunity following injection of purified EVs released by cells in culture, very little is known about the immunoregulatory role of these vesicles in vivo. There are only a few examples where the effect of inhibiting the secretion of EVs from a certain cell type has been examined in vivo148. Understanding how the EVs from different immune cell types function to regulate immunity should result in the development of new therapeutic approaches. For example, inhibiting tumor-derived exosome function should result in reduced metastasis and a better anti-tumor immune response. Alternatively, it could be possible to deplete EVs from the blood of cancer patients by apheresis. In contrast, modifying APC or MSCs function in vivo or in vitro to facilitate release of more immunosuppressive EVs could be used for treating autoimmune, inflammatory and graft versus host diseases as well as facilitate transplantation. The feasibility and safety of using EVs clinically has been demonstrated in several clinical studies using autologous, blood-derived EVs or EVs from MSCs. Clearly, EVs are important mediators and regulators of immunity for which there are and will be widespread therapeutic applications.
Glossary terms
Tetraspanins | Evolutionary conserved four trans-membrane domain proteins that associate in cis with other tetraspanins, integrins, MHC molecules, or signaling receptors in tetraspanin-enriched domains, which regulate multiple cell functions including cell adhesion, morphogenesis, proliferation, differentiation, synapse formation, and tumor invasion. |
microRNAs (miRNAs) | Small (~22 nucleotides) noncoding RNAs that regulate post-transcriptionally gene expression by binding seed sequences on target mRNAs, which results in mRNA degradation or mRNA translation inhibition. |
Cross-dressing | Passage of intact preformed p-MHC Class-I or -II complexes from a donor cell(s) to the surface membrane of an acceptor APC(s) or another type of target cell(s). The transferred p-MHC complexes are recognized as such by the TCRs of T cells, without the need of further Ag-processing. |
Cross-presentation | Transfer of biosynthesized Ag in a complex form (apoptotic cell fragments, HSP-associated, etc) from donor (i.e. infected, tumor) cells to the cytosol of acceptor APCs for proteasome and TAP-dependent processing and presentation through MHC Class-I molecules to CD8 T cells. In certain cases, the acquired Ag can be presented through MHC Class-I via a TAP-independent mechanism that involves delivery of the Ag to MHC Class-I containing phagolysosomes. |
RISC (miRNA-induced silencing complex) | Assembly of single-stranded miRNAs based-paired with the target mRNAs associated to accessory proteins (i.e. Argonaute, GW182) required for the regulatory function of miRNAs. |
Delayed-type hypersensitivity (DTH) model | A CD4+ T-cell-driven, Ag-specific immune response induced first by intra-dermal Ag-immunization followed by injection of the Ag in the skin of the ear or the footpad. The immune response is measured by swelling and redness. |
Iccosomes (immune complex coated bodies) | Membrane vesicles (0.25–0.38µm in diameter) pinched off from the delicate processes of FDCs. Iccosomes carry ICs with native Ags and C3-derived complement fragments on the surface, which activate B cells. During B-cell activation, iccosomes are recognized and internalized by follicular B cells in an Ag-specific manner. |
Myeloid-derived suppressor cells | heterogeneous population of leukocytes of myeloid lineage that increase in number during cancer and inflammation, and suppress T-cell responses. They include myeloid progenitor cells, immature macrophages, granulocytes and DCs. |
Mesenchymal stem cells | multipotent stromal cells that can differentiate into a variety of cell types derived from the mesoderm including osteoblasts, chondrocytes and adipocytes, but not hematopoietic cells. |
References
Full text links
Read article at publisher's site: https://doi.org/10.1038/nri3622
Read article for free, from open access legal sources, via Unpaywall:
https://europepmc.org/articles/pmc4350779?pdf=render
Citations & impact
Impact metrics
Citations of article over time
Alternative metrics
Smart citations by scite.ai
Explore citation contexts and check if this article has been
supported or disputed.
https://scite.ai/reports/10.1038/nri3622
Article citations
Exosome therapeutics for non-small cell lung cancer tumorigenesis.
Cancer Cell Int, 24(1):360, 30 Oct 2024
Cited by: 0 articles | PMID: 39478574 | PMCID: PMC11523890
Review Free full text in Europe PMC
Proteomic analysis of plasma total exosomes and placenta-derived exosomes in patients with gestational diabetes mellitus in the first and second trimesters.
BMC Pregnancy Childbirth, 24(1):713, 30 Oct 2024
Cited by: 0 articles | PMID: 39478498 | PMCID: PMC11523606
miRNA packaging into small extracellular vesicles and implications in pain.
Pain Rep, 9(6):e1198, 23 Oct 2024
Cited by: 0 articles | PMID: 39450410 | PMCID: PMC11500789
Review Free full text in Europe PMC
Evolving understanding of autoimmune mechanisms and new therapeutic strategies of autoimmune disorders.
Signal Transduct Target Ther, 9(1):263, 04 Oct 2024
Cited by: 0 articles | PMID: 39362875 | PMCID: PMC11452214
Review Free full text in Europe PMC
Novel insights into the role of immunomodulatory extracellular vesicles in the pathogenesis of liver fibrosis.
Biomark Res, 12(1):119, 12 Oct 2024
Cited by: 0 articles | PMID: 39396032 | PMCID: PMC11470730
Review Free full text in Europe PMC
Go to all (1,237) article citations
Data
Data behind the article
This data has been text mined from the article, or deposited into data resources.
BioStudies: supplemental material and supporting data
Similar Articles
To arrive at the top five similar articles we use a word-weighted algorithm to compare words from the Title and Abstract of each citation.
Exosomes: small vesicles participating in intercellular communication.
Int J Biochem Cell Biol, 44(1):11-15, 19 Oct 2011
Cited by: 285 articles | PMID: 22024155
Review
Exosomes and Ectosomes in Intercellular Communication.
Curr Biol, 28(8):R435-R444, 01 Apr 2018
Cited by: 436 articles | PMID: 29689228
Review
Emerging roles of tetraspanins in plant inter-cellular and inter-kingdom communication.
Plant Signal Behav, 14(4):e1581559, 04 Mar 2019
Cited by: 18 articles | PMID: 30829110 | PMCID: PMC6512927
Review Free full text in Europe PMC
Extracellular vesicles in physiological and pathological conditions.
Blood Rev, 27(1):31-39, 20 Dec 2012
Cited by: 322 articles | PMID: 23261067
Review
Funding
Funders who supported this work.
NHLBI NIH HHS (4)
Grant ID: HL075512
Grant ID: HL077545
Grant ID: R01 HL075512
Grant ID: R01 HL077545
NIA NIH HHS (6)
Grant ID: AG024827
Grant ID: P30 AG024827
Grant ID: AG033907
Grant ID: AG043376
Grant ID: P01 AG043376
Grant ID: R21 AG033907
NIAMS NIH HHS (4)
Grant ID: AR051456
Grant ID: AR055373
Grant ID: R01 AR055373
Grant ID: R01 AR051456