Abstract
Free full text

A Surviving Intact Branch Stabilizes Remaining Axon Architecture after Injury as Revealed by In Vivo Imaging in the Mouse Spinal Cord
Associated Data
SUMMARY
The complex morphology of axons presents a challenge in understanding axonal responses to injury and disease. By in vivo 2-photon imaging of spinal dorsal column sensory axons, we systematically examined the effect of injury location relative to the main bifurcation point on axon degeneration and regeneration following highly localized laser injuries. Retrograde but not anterograde degeneration was strongly blocked at the bifurcation point at both the acute and subacute phases. Eliminating either the ascending or descending branch led to a poor regenerative response while eliminating both led to a strong regenerative response. Thus, a surviving intact branch suppresses both retrograde degeneration and regeneration of the injured branch, thereby stabilizing the remaining axon architecture. Regenerating axons exhibited a dynamic pattern with alternating phases of regeneration and pruning over a chronic period. In vivo imaging continues to reveal new insights on axonal responses to injury in the mammalian spinal cord.
INTRODUCTION
The complexity in axonal morphology dictates complexities in axonal responses to injury. Despite the expanding knowledge on the molecular control of axon regeneration in the central nervous system (CNS) (Bradke and Marin, 2014), our understanding of how individual axons respond to injury in their native state remains incomplete. In particular, our knowledge on how an axon reacts to injuries at different locations along the axon remains rudimentary. Axons are highly complex structures. Axonal branching is a cardinal feature of axonal morphology underlying many of the intricate physiological properties of the nervous system. From simple bifurcation to multiple collateral formation to elaborate terminal arborization, axonal branching provides a way for a neuron to communicate with a multitude of synaptic partners often located in diverse areas of the nervous system (Gibson and Ma, 2011). A primary axonal branch may further branch, leading to secondary and higher order branches. This morphological complexity presents a challenge in understanding how an axon responds to injuries. Do injuries to different locations on the axon relative to a branch point elicit the same or different responses? If different, are there rules or logic in this differential response?
Studies of spinal axon regeneration have traditionally relied on animal models of spinal cord injury (Lee and Lee, 2013). Typically, a mechanical injury such as a transection, crush or contusion is applied to the cord; axons are labeled with a surgically applied and/or genetically encoded tracer, which is then detected and visualized on terminally collected samples. Such conventional experimental paradigms make it difficult, if not impossible, to systematically examine the effect of subtle changes in injury location on axonal responses.
In vivo optical imaging in the spinal cord represents a radically different approach to study axonal responses to injury as it allows for the examination of the same axons in living animals over time (Laskowski and Bradke, 2013). The first of such a study, using wide-field fluorescence microscopy in conjunction with a pinprick lesion, led to the discovery of acute axon degeneration and provided the first time-lapse recordings of axon regeneration in the injured mammalian CNS (Kerschensteiner et al., 2005). Since then, spinal cord in vivo imaging has been used to study microtubule stability in retraction bulb formation (Erturk et al., 2007), the conditioning lesion effect in chronically injured axons (Ylera et al., 2009), axon – blood vessel interaction during regeneration (Dray et al., 2009), the behavior of regenerating sensory axons after entering the spinal cord following a dorsal root crush (Di Maio et al., 2011), the phase-specific role of STAT3 in regeneration (Bareyre et al., 2011) and axon – macrophage interaction in subacute axon degeneration (Evans et al., 2014).
In collaboration with Davalos and Akassoglou, we previously described a method to repetitively image densely labeled cells and cellular processes in the spinal cord with 2-photon microscopy without disrupting dura or the need for intubation or image post-processing (Davalos et al., 2008). Here we use this in vivo imaging paradigm in conjunction with highly localized laser axotomies to systematically examine the effect of injury location relative to the main bifurcation point in the cord on axonal responses to injury. We discovered a suppressive effect of a surviving intact axonal branch on retrograde degeneration and regeneration of the injured branch. Our data start to reveal rules and logic in axonal responses to injuries at different parts of the axon and prompt new questions on the mechanisms of axon degeneration and regeneration after CNS injury.
RESULTS
We used the Thy1-YFP-H line (Feng et al., 2000) that strongly labels a subset of spinal axons with the yellow fluorescent protein (Fig. S1A) and is widely used in the literature, including injury and imaging studies in the spinal cord (Carter et al., 2008; Davalos et al., 2008; Farrar et al., 2012). We focused on the superficially located – and thus the most optically accessible – dorsal column sensory axons (boxed area in Fig. S1A). These large diameter axons arise from large sensory neurons (Di Maio et al., 2011) in the dorsal root ganglia (DRGs) outside the cord (Fig. S1B). Their primary axon bifurcates, with one peripheral branch and one central branch that extends into the cord. Inside the cord, the central branch bifurcates again, with one ascending branch extending rostral towards the brain in the dorsal column white matter and one descending branch coursing down the spinal cord (Fig. S1B. A = ascending branch, D = descending branch, M = main branch). Along the spinal cord, dorsal column sensory axons send out collaterals that innervate the grey matter. In this study, we limited our analyses to a small area surrounding the main bifurcation or branch point in the cord (BP, blue arrow in Fig. S1B). Peripheral myelin protein zero (P0) immunostaining indicated that the axons to be imaged are well within the CNS (Fig. S1C).
We used the femtosecond 2-photon laser with the minimum laser power and duration necessary to produce highly localized laser axotomies without visibly affecting nearby labeled axons. To systematically study the effect of varying injury location relative to the main bifurcation point and to facilitate tracking of the injured axons, we directed laser axotomies near the branch point (~100-150 µm away, unless noted otherwise) either before or after an axon bifurcates in the cord (as marked by a red cross in Fig. S1B). At each imaging session, we first acquired a low magnification image of the densely labeled dorsal column sensory axons as a guiding roadmap (Fig. S1D). A chosen area of interest (boxed area in Fig. S1D) was then imaged at a higher magnification with 2-photon microscopy (Fig. 1A). Up to 3 axons were axotomized per mouse and imaged as often as every 5-15 minutes for ~3-5 hours after axotomy on Day 0; at later time points (5 days, 5 weeks, or 6 months), each imaging session was intended to capture a snapshot.
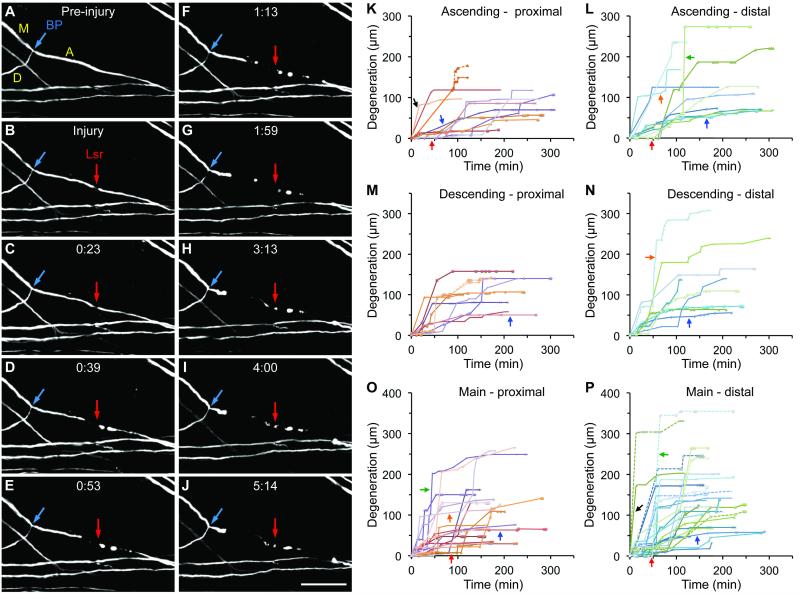
(A-J) Selected 2-photon images from a time-lapse series taken every ~5-15 min showing acute degeneration via fragmentation and retraction following an ascending branch axotomy. BP, branch point (blue arrow); M, main branch; A, ascending branch; D, descending branch; Lsr, Laser axotomy (red arrow). Rostral is always to the right. Acute times shown are in hours and minutes (h:mm); “Injury” is the time point immediately after axotomy. Note that in (H-J) the tip of the proximal segment resembles retraction bulb-like structures described in Kerschensteiner et al. (2005). Scale bar = 100 µm. (K-P) Acute degeneration profiles of individual axonal segments clustered around the injury location (ascending, descending or main branch) and the axonal segment (proximal or distal). See text for descriptions of colored arrows. In (K, M, P), a branch point breach is indicated by a solid line splitting into one solid line and one dotted line of the same color. See also Movie S1, Figures S1 and S2.
Consistent with previous studies with either a mechanical or larger laser injury (Farrar et al., 2012; Kerschensteiner et al., 2005), axons following highly localized laser axotomies also displayed bidirectional acute degeneration within minutes to hours regardless of whether an ascending (Fig. 1A-J, Movie S1; Fig. S2A-F), descending (Fig. S2G-I) or main branch (Fig. S2J-L) was axotomized. The proximal segment degenerated retrogradely and the distal segment degenerated anterogradely. Acute degeneration occurred via two non-mutually exclusive modes: fragmentation, where initial beading/blebbing quickly gave way to discontinuous fragments that could linger for hours, and retraction, where the axonal tip was seen slowly moving backward (away from the injury site) without leaving behind any visible axonal fragments. Many axonal segments exhibited a combination of fragmentation and retraction (Fig. 1), while some exhibited a dominant degeneration mode of either fragmentation (Fig. S2A-C) or retraction (Fig. S2D-F). Glial fibrillary acidic protein (GFAP) immunostaining indicated that there was minimal local astroglial reactivity (data not shown) as reported (Ylera et al., 2009).
Degeneration profiles of individual axonal segments, clustered based on the injury location (ascending, descending or main branch) and the axonal segment (proximal or distal), exhibited a high level of variability (Fig. 1K-P). Some initiated degeneration soon after axotomy (black arrows) while others exhibited an initial delay (red arrows). Sometimes degeneration slowly progressed over an extended period of time (blue arrows) while at other times there was a burst of degeneration as reflected by a precipitous slope (green arrows). A closer inspection of the imaged axons indicates that these bursts of degeneration were often associated with fragmentation. Still other axonal segments exhibited a more complex degeneration profile with multiple, alternating phases of slow and fast degeneration (orange arrows). Similar heterogeneity has been reported previously with in vivo imaging using the same YFP-H line but with a larger laser injury (Farrar et al., 2012). Despite this heterogeneity, acute degeneration typically subsided within 1-3 hours after axotomy.
Independent of injury location, fragmentation was the dominant mode of acute axon degeneration, as assessed by either the absolute degeneration distance (Fig. S2M) or the proportion of cumulative degeneration distance (Fig. S2N). Axonal segments that degenerated primarily via fragmentation (i.e. fragmentation accounting for ≥80% of the distance degenerated) exhibited a faster degeneration speed than those primarily via retraction; axonal segments with a more mixed mode (>20% but <80% for any one mode) fared in between (Fig. S2O). Regardless of injury location, there was a consistent trend for more fragmentation in the distal segments as compared with the proximal segments (Fig. S2P), providing the first hint that there may be a level of asymmetry in bidirectional acute degeneration.
Following ascending or descending branch axotomies, acute degeneration of the proximal segments often proceeded to (Fig. S2C) or near the branch point leaving a stub (Fig. 1J, ,2G,2G, S2F, I) but rarely breached the branch point. This prompted us to hypothesize that the branch point serves as a barrier for retrograde acute degeneration. The branch point remained resistant to degeneration even when the axotomy site was placed closer to the branch point, at ~50 µm away (e.g., Fig. 2A-C). To better illustrate this barrier effect, we plotted the degeneration distance against the distance between the axotomy site and the branch point, where any data point for a proximal segment (solid circle) above the 45°line represents an inc idence of branch point breach (Fig. 2I, J). Following ascending or descending branch axotomies placed ~50-150 µm from the branch point, only in ~11% (3/27) of the cases did retrograde acute degeneration of the proximal segment breach the branch point (purple arrows, Fig. 2I, J). In ~63% (17/27) of the cases retrograde degeneration stopped within 25 µm of the branch point, including ~33% (9/27) stopping exactly at the branch point. This close clustering of the degeneration endpoints is strong indication that the branch point acts as a barrier to retrograde degeneration. In contrast, anterograde degeneration of the corresponding distal segments (open triangles), where there was no branch point along the degeneration path, proceeded more freely: ~56% (15/27) degenerated beyond the distance equivalent to that represented by the 45° line, with the degeneration endpoints spreading out up to ~300 µm.

(A-D) Degeneration following a descending branch axotomy placed at ~50 µm from the branch point; (E-H) Degeneration following an ascending branch axotomy placed between 100-150 µm from the branch point. Yellow arrows, landmarks to track axons through multiple imaging sessions; white arrow, uninjured axon. Scale bar = 100 µm. (I-N) Degeneration distance is plotted against the distance between axotomy site and branch point. See text for data interpretation. Note that the data points marked by open circles in (K, L) are the same data points marked by solid circles in (I, J) respectively. Orange arrowhead, two data points coincidentally superimposed on one another.
Notably, the branch point remained a barrier even at the subacute time point, i.e., 5 days after axotomy (Fig. 2D, H, K, L). Proximal segments that had not degenerated to the branch point at the last observation time point on Day 0 had more or less degenerated further by Day 5, but none had breached the branch point (0% or 0/24; green triangles, Fig. 2K, L). In a vast majority of cases (~88% or 21/24), retrograde degeneration stopped at the branch point, as strikingly illustrated by the degeneration endpoints lining up the 45°line (green triangles). In the three cases where retrograde degeneration had breached the branch point on Day 0, further degeneration proceeded more freely by Day 5 (purple arrows, Fig. 2K, L).
In contrast, following main branch axotomies, anterograde degeneration of the distal segments was not blocked – not even temporarily – by the branch point (open triangles, Fig. 2M). In ~42% (8/19) of the cases anterograde degeneration of the distal segment breached the branch point within hours of a main branch axotomy. This frequency is indistinguishable from that of retrograde degeneration of the corresponding proximal segments proceeding beyond the equivalent distance (~42% or 8/19), where there was no branch point along the degeneration path (solid circles, Fig. 2M). Thus, the branch point is a barrier for retrograde but not anterograde acute degeneration and that it remains a potent barrier even at the subacute time point.
Placing the ascending branch axotomy site further away from the branch point (between ~500 and ~900 µm, averaged at ~700 µm) allowed a more extended distance for the proximal segment to degenerate retrogradely without encountering a branch point (Long Distance, or LD in Fig. S1B). Degeneration, either retrograde or anterograde, extended up to ~250 µm from the axotomy site (Fig. 2N) and none of the retrograde degeneration events breached the branch point even 5 days after injury (e.g., Fig. S3A-C). This intrinsic limit of degeneration distance (~250 µm) is comparable to that for distal degeneration after ascending/descending branch axotomies and proximal degeneration after main branch axotomies, where there was no branch point along the degeneration path (~300 µm) (Fig. 2I, J, M).
Five days after main branch axotomies, many axons regenerated from the terminal end of the degenerated proximal segment (e.g., Fig. 3A-C’), consistent with previous observations (Kerschensteiner et al., 2005). Anterogradely, Wallerian degeneration had taken over, with fragmented remnants of the distal segment (brown arrows). Regeneration occurred via elongation, branching or a combination of both. Primary branches may give rise to secondary branches (Fig. 3C, C’). Growth cone-like structures could be seen at the tip of the newly growing axonal branches (magenta arrowheads, Fig. 3C’). Elongation may proceed in the reverse direction following a U-turn (Fig. 3C/C’, S3C’, ,4B).4B). In contrast to the smooth appearance of intact axons, regenerating axons had an irregular and often thinner axon diameter and took tortuous, winding paths in seemingly random directions.

(A-C’) An example of axon regeneration via branching and elongation observed on Day 5 after a main branch axotomy. Green arrows, newly regenerated axons; brown arrows, Wallerian degeneration; magenta arrowheads, structures with growth cone appearance. Scale Bar = 100 µm (A-C); 50 µm (C’). (D) Regeneration frequency on Day 5 after axotomy. ***p < 0.001, Chi-square and Fisher’s exact test. A, ascending; D, descending; M, main branch; Db, double branch axotomy; LD, Long Distance (on ascending branch). (E, F) Quantification of regeneration in terms of axonal growth (E) including maximum, total and average per branch, and branch numbers (F) for primary (1°) and secondary (2°) branches with t he same color coding as in (D). See also Figure S3.
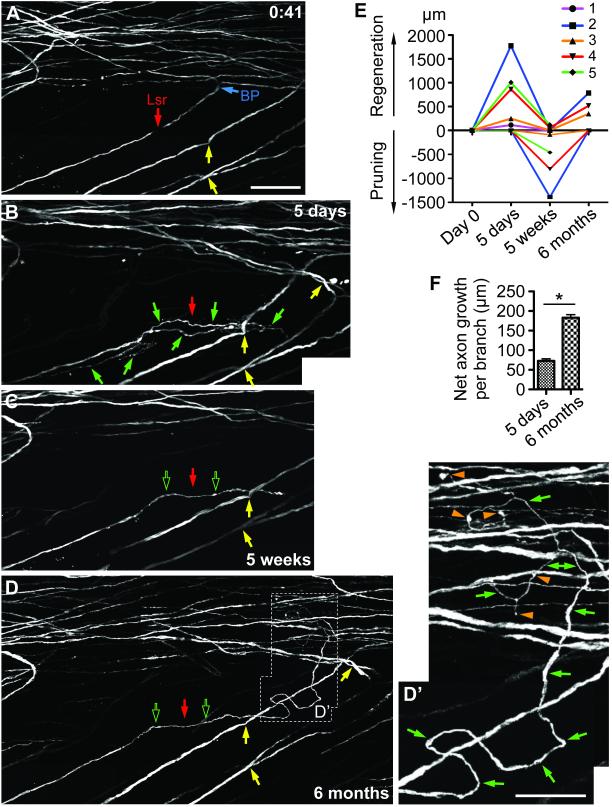
(A-D’) An example of main branch axotomy that led to dynamic regeneration, pruning and remodeling up to the 6-month time point. Note axon regeneration (including a U-turn) observed at 5 days, pruning at 5 weeks and new axon regeneration at 6 months. Solid green arrows, new axonal growth; hollow green arrows, previous axonal growth that sustained; orange arrowheads, varicosities. Scale bar = 100 µm (A-D); 50 µm (D’). (E) New axon regeneration and pruning at each observation time point for individual axons (not including degeneration on Day 0). Axons #1 and #2 were from the same mouse. (F) Net axon growth per branch. *p < 0.05, Student’s t-test, n = 3. See also Figure S4.
There was a significant effect of the injury location relative to the branch point on the regeneration frequency among main, ascending and descending branch axotomies (X2 = 25.95, df = 2, p < 0.0001). The frequency of regeneration following main branch axotomies (~89%, or 16/18) was substantially higher than that after ascending (~13%, or 2/16) or descending branch axotomies (~11%, or 1/9) (Fig. 3D). For ascending and descending branch axotomies, there was a 100% correlation between the incidence of branch point breach on Day 0 and a detectable regenerative response on Day 5: three out of 25 axotomies had both (e.g., Fig. S3D-F’) while 22 exhibited neither.
This injury location-specific effect on regeneration could be explained by the suppression of regeneration either by the branch point per se or the remaining intact branch. To distinguish these two possibilities, we axotomized both the ascending and descending branches simultaneously at ~100-150 µm from the branch point (double branch, or Db, axotomy, Fig. S1B). If a branch point per se suppresses regeneration, a higher regeneration frequency would be associated with a branch point breach. If the remaining intact branch suppresses regeneration, a higher regeneration frequency would be expected regardless of any branch point breach. After double branch axotomies, axons regenerated in ~67% (6/9) of the cases, approaching the regeneration frequency for main branch axotomies (~89%, Fig. 3D). When there was a branch point breach, regeneration occurred in 2 of 4 cases (~50%). When there was no branch point breach, regeneration occurred in 4 out of 5 cases (~80%) (exemplified in Fig. S3G-I’). Thus, compared with single ascending or descending branch axotomies, double branch axotomies led to a higher regeneration frequency regardless of a branch point breach. These data support the hypothesis that following ascending/descending branch axotomies, the remaining intact branch, rather than the branch point per se, suppresses regeneration.
Moving the axotomy site on the ascending branch further away from the branch point (~700 µm, LD axotomies, Fig. S1B) led to an intermediate regeneration frequency (~43%, or 3/7) (Fig. 3D, exemplified in Fig. S3A-C’). Quantitative analyses indicate that out of the three injury locations that gave a considerable regeneration frequency, main branch axotomies led to the most regeneration in both the distances regenerated (maximum, total, average per branch) and the branch numbers (total, secondary), while double branch axotomies in many respects resembled or approached main branch axotomies. Thus, out of the two conditions that favor regeneration the most (main branch and double branch axotomies), no intact axonal branch (ascending or descending) had remained.
To address whether regeneration observed on Day 5 (subacute) would sustain or abort at later time points, we followed 5 axons to 5 weeks (subchronic), 3 of which were further followed to 6 months (chronic) after main branch axotomies. The small numbers of axons examined reflected the technical challenges of imaging at these later time points. Remarkably, all 5 axons examined at 5 weeks exhibited some pruning (e.g., Fig. S4 and 4A-C). There were also instances of modest new growth at 5 weeks in addition to the more noticeable pruning (Fig. S4C). Most remarkably, all three axons examined at 6 months exhibited new regeneration (e.g., Fig. 4D, D’). Varicosities could be observed along the regenerated axons and at their terminal tips (orange arrowheads). Whether these represent presynaptic-like structures reported recently on injured axons in the spinal cord (Di Maio et al., 2011; Filous et al., 2014) requires further investigation.
Quantitative analysis on new regeneration vs. pruning of individual axons at different time points indicated a dynamic temporal pattern with alternating phases of regeneration and pruning (Fig. 4E). Individual axons varied in their dynamics, with more dynamic axons exhibiting more regeneration as well as more pruning and vice versa (e.g., compare axons #2 and #3), indicating that the dynamics of an axon’s injury response reflect its intrinsic properties. The length of net axon growth per branch at 6 months was significantly longer than that at 5 days, suggesting that there was a switch from a more branching phenotype at the subacute time point towards a more elongating phenotype at the chronic time point (Fig. 4F). Together, these data illustrate that axonal regeneration, pruning and remodeling continue weeks and even months after axotomy.
DISCUSSION
The ability to pinpoint the axotomy site with laser precision and to visualize axonal behavior over time in vivo allowed us to systematically examine, for the first time in modern neurobiology, the effect of injury location relative to a branch point on axon degeneration and regeneration in the adult mammalian CNS. Our data support the hypothesis that a surviving intact branch blocks retrograde degeneration of the injured branch from breaching the branch point and also suppresses its regeneration, thus stabilizing the remaining axon architecture. Because collateral sprouting, a form of axonal branching, is widely recognized as an underlying mechanism for some spontaneous recovery after CNS injury (Chen and Zheng, 2014), our finding that an intact axonal branch directly impacts the degenerative and regenerative responses of an injured branch is of heightened significance.
When the axotomy site was placed relatively close to the bifurcation point, retrograde acute degeneration of the proximal segment was consistently blocked by the main bifurcation point with few exceptions. Even at the subacute time point, the bifurcation point remained a potent barrier. Indeed, this barrier effect was most pronounced at 5 days after injury when most degenerative events had stopped at the branch point. The fact that anterograde acute degeneration of the distal segment was not blocked by the branch point after main branch axotomies provides strong evidence that it is not the branch point per se but rather the presence of a surviving intact branch that suppresses further degeneration.
The differential effect of a branch point on the proximal vs. distal segment illustrates a previously unrecognized asymmetry in the bidirectional acute axon degeneration. This asymmetry implicates a protective cell body influence even at this early stage after axotomy. Alternatively, synaptic activities in the distal segment may accelerate acute degeneration. However, a recent study in Drosophila showed that following axotomy the distal stump does not fire action potentials (Mishra et al., 2013), arguing against a major role for nerve excitability or synaptic activity in distal degeneration.
Injury location relative to the bifurcation point also impacts regeneration. Axotomizing the main branch led to a high regeneration frequency. Axotomizing the ascending or descending branch alone led to a low regeneration frequency; even when there was regeneration, in our study a branch point breach had invariably occurred, leading to the elimination of both branches. Importantly, double branch axotomies led to a higher regeneration frequency irrespective of a branch point breach. Thus, just as in degeneration, it is not the branch point per se but a surviving intact branch that suppresses the regeneration of the injured branch. Intriguingly, moving the axotomy site further away from the branch point (as in Long Distance axotomies) increased the regeneration frequency, implying that the length of the injured branch that remains also influences its fate.
The dual role of an intact branch in suppressing both further retrograde degeneration and regeneration of the injured branch makes economic sense for an injured neuron. After one axonal branch is injured, the remaining intact branch still has synaptic contacts, which may serve as a stabilizer. By actively suppressing retrograde degeneration of the injured branch from breaching the branch point, the intact branch would maximize structural and functional preservation. When both branches are severed, loss of all synaptic contacts may prompt the axon to devote energy and resource to regenerate. Interestingly, the presence of a synaptic branch also inhibits axon regeneration in C. elegans (Wu et al., 2007), suggesting that this is an evolutionarily conserved mechanism in regulating axon regeneration. In addition to the loss of synaptic contacts, a stronger injury signal (Cho et al., 2013) following main or double branch axotomies may also contribute to a stronger regenerative response.
Unbeknownst to us when we initially wrote this paper, it was Cajal who first noted the phenomenon of complete degeneration of axotomized terminal branches (Ramón y Cajal, 1928). By studying histological samples of experimental spinal cord injury, Cajal noted, “the well-known bifurcation into an ascending and a descending branch” of dorsal column sensory axons was transformed “into arciform fibres which penetrate into the grey matter… The traumatism has rendered useless the descending branch… This branch is radically destroyed, so that after a month and a half it is impossible to see any sign of bifurcation”. He then explained, “Traumatic degeneration represents a curious mechanism of reaction of an exquisitely economical and utilitarian character. Thanks to it, nature gets rid, so to speak, of useless mouths of protoplasmic segments that serve no useful purpose”. Cajal even proposed the inhibitory effect of a surviving collateral branch on degeneration: ”the maintenance of the connections, and therefore the continued functioning, of a collateral, constitute a hindrance to traumatic degeneration”.
Using time-lapse in vivo imaging, our study provided direct, conclusive evidence for branch elimination after axotomy. While Cajal observed complete retrograde degeneration even when injury was localized at some distance from the branch point, we observed this only when axotomy was placed relatively close to the branch point. Whether this reflected differences in lesion severity remains to be tested in future studies. It is conceivable that a larger, more clinically relevant injury than the laser injury applied here would elicit a more robust, sustained degenerative response including both an earlier, neuron-intrinsic phase and a later, macrophage-mediated phase (Evans et al., 2014). Most importantly, we advanced the concept of a surviving intact branch serving as a stabilizer by showing that it also suppresses the regeneration of the injured branch.
Regenerating axons exhibited a dynamic temporal pattern with alternating phases of regeneration and pruning over a chronic period. Similar dynamic axonal behaviors over a chronic period after axotomy have been documented by in vivo imaging in the brain (Canty et al., 2013). This implicates a wider therapeutic window for promoting CNS axon regeneration than previously envisioned. Future studies may benefit from increased duration and frequency by using a chronic window or chamber over the imaged area (Farrar et al., 2012; Fenrich et al., 2012). We anticipate that in vivo spinal cord imaging will continue to provide novel insights and generate new testable hypotheses on axonal responses to injury.
EXPERIMENTAL PROCEDURES
As described (Davalos et al., 2008), an anesthetic mix of ketamine-xylazine-acepromazine and stabilizing spinal column, head and tail clamps were used to minimize movement-associated artifacts. After a T9 laminectomy, a well of artificial CSF was held with a rim of petroleum jelly to allow for the preservation of the cord and the immersion of the objective lens. After each imaging session, the wound was closed and skin sutured. Each subsequent imaging session involved reopening of the cord and clearing of any obstructing tissue prior to imaging. We used an FV300 Olympus microscope with a Spectra Physics Mai-Tai laser tuned to 920 nm for imaging and 820 nm for laser axotomy. Stacks of images were collapsed to 2D for presentation and quantification of degeneration, with 3D data analyzed for regeneration using ImageJ software.
ACKNOWLEDGEMENT
Supported by grants from the Dana Foundation, NIH/NINDS (R01NS054734) and the Roman Reed Fund to B.Z.; NRSA predoctoral fellowship F31NS074867 to A.O.L.; NRSA postdoctoral fellowship F32NS056697 to J.K.L. In vivo imaging was conducted at the UCSD Neuroscience Microscopy Imaging Core (P30NS047101). We thank Dimitrios Davalos for demoing laser ablation techniques; Jeffrey Kwan, Andrea Chan, Ben Gallarda, Omeed Ghassemi and Pearl Shih for technical assistance; Mark Lawson for advice on statistic analyses; Le Ma, Meifan Chen and Jessica Meves for helpful comments on the manuscript.
Footnotes
Publisher's Disclaimer: This is a PDF file of an unedited manuscript that has been accepted for publication. As a service to our customers we are providing this early version of the manuscript. The manuscript will undergo copyediting, typesetting, and review of the resulting proof before it is published in its final citable form. Please note that during the production process errors may be discovered which could affect the content, and all legal disclaimers that apply to the journal pertain.
REFERENCES
- Bareyre FM, Garzorz N, Lang C, Misgeld T, Buning H, Kerschensteiner M. In vivo imaging reveals a phase-specific role of STAT3 during central and peripheral nervous system axon regeneration. Proc Natl Acad Sci U S A. 2011;108:6282–6287. [Europe PMC free article] [Abstract] [Google Scholar]
- Bradke F, Marin O. Editorial overview: development and regeneration: nervous system development and regeneration. Curr Opin Neurobiol. 2014;27:iv–vi. [Abstract] [Google Scholar]
- Canty AJ, Huang L, Jackson JS, Little GE, Knott G, Maco B, De Paola V. In-vivo single neuron axotomy triggers axon regeneration to restore synaptic density in specific cortical circuits. Nat Commun. 2013;4:2038. [Abstract] [Google Scholar]
- Carter LM, Starkey ML, Akrimi SF, Davies M, McMahon SB, Bradbury EJ. The yellow fluorescent protein (YFP-H) mouse reveals neuroprotection as a novel mechanism underlying chondroitinase ABC-mediated repair after spinal cord injury. J Neurosci. 2008;28:14107–14120. [Europe PMC free article] [Abstract] [Google Scholar]
- Chen M, Zheng B. Axon plasticity in the mammalian central nervous system after injury. Trends Neurosci. 2014;37:583–593. [Europe PMC free article] [Abstract] [Google Scholar]
- Cho Y, Sloutsky R, Naegle KM, Cavalli V. Injury-induced HDAC5 nuclear export is essential for axon regeneration. Cell. 2013;155:894–908. [Europe PMC free article] [Abstract] [Google Scholar]
- Davalos D, Lee JK, Smith WB, Brinkman B, Ellisman MH, Zheng B, Akassoglou K. Stable in vivo imaging of densely populated glia, axons and blood vessels in the mouse spinal cord using two-photon microscopy. J Neurosci Methods. 2008;169:1–7. [Europe PMC free article] [Abstract] [Google Scholar]
- Di Maio A, Skuba A, Himes BT, Bhagat SL, Hyun JK, Tessler A, Bishop D, Son YJ. In vivo imaging of dorsal root regeneration: rapid immobilization and presynaptic differentiation at the CNS/PNS border. J Neurosci. 2011;31:4569–4582. [Europe PMC free article] [Abstract] [Google Scholar]
- Dray C, Rougon G, Debarbieux F. Quantitative analysis by in vivo imaging of the dynamics of vascular and axonal networks in injured mouse spinal cord. Proc Natl Acad Sci U S A. 2009;106:9459–9464. [Europe PMC free article] [Abstract] [Google Scholar]
- Erturk A, Hellal F, Enes J, Bradke F. Disorganized microtubules underlie the formation of retraction bulbs and the failure of axonal regeneration. J Neurosci. 2007;27:9169–9180. [Europe PMC free article] [Abstract] [Google Scholar]
- Evans TA, Barkauskas DS, Myers JT, Hare EG, You JQ, Ransohoff RM, Huang AY, Silver J. High-resolution intravital imaging reveals that blood-derived macrophages but not resident microglia facilitate secondary axonal dieback in traumatic spinal cord injury. Exp Neurol. 2014;254:109–120. [Europe PMC free article] [Abstract] [Google Scholar]
- Farrar MJ, Bernstein IM, Schlafer DH, Cleland TA, Fetcho JR, Schaffer CB. Chronic in vivo imaging in the mouse spinal cord using an implanted chamber. Nat Methods. 2012;9:297–302. [Europe PMC free article] [Abstract] [Google Scholar]
- Feng G, Mellor RH, Bernstein M, Keller-Peck C, Nguyen QT, Wallace M, Nerbonne JM, Lichtman JW, Sanes JR. Imaging neuronal subsets in transgenic mice expressing multiple spectral variants of GFP. Neuron. 2000;28:41–51. [Abstract] [Google Scholar]
- Fenrich KK, Weber P, Hocine M, Zalc M, Rougon G, Debarbieux F. Long-term in vivo imaging of normal and pathological mouse spinal cord with subcellular resolution using implanted glass windows. J Physiol. 2012;590:3665–3675. [Abstract] [Google Scholar]
- Filous AR, Tran A, Howell CJ, Busch SA, Evans TA, Stallcup WB, Kang SH, Bergles DE, Lee SI, Levine JM, et al. Entrapment via synaptic-like connections between NG2 proteoglycan+ cells and dystrophic axons in the lesion plays a role in regeneration failure after spinal cord injury. J Neurosci. 2014;34:16369–16384. [Europe PMC free article] [Abstract] [Google Scholar]
- Gibson DA, Ma L. Developmental regulation of axon branching in the vertebrate nervous system. Development. 2011;138:183–195. [Europe PMC free article] [Abstract] [Google Scholar]
- Kerschensteiner M, Schwab ME, Lichtman JW, Misgeld T. In vivo imaging of axonal degeneration and regeneration in the injured spinal cord. Nat Med. 2005;11:572–577. [Abstract] [Google Scholar]
- Laskowski CJ, Bradke F. In vivo imaging: a dynamic imaging approach to study spinal cord regeneration. Exp Neurol. 2013;242:11–17. [Abstract] [Google Scholar]
- Lee DH, Lee JK. Animal models of axon regeneration after spinal cord injury. Neurosci Bull. 2013;29:436–444. [Europe PMC free article] [Abstract] [Google Scholar]
- Mishra B, Carson R, Hume RI, Collins CA. Sodium and potassium currents influence Wallerian degeneration of injured Drosophila axons. J Neurosci. 2013;33:18728–18739. [Europe PMC free article] [Abstract] [Google Scholar]
- Ramón y Cajal S. Degeneration and Regeneration of the Nervous System. Hafner; New York: 1928. [Google Scholar]
- Wu Z, Ghosh-Roy A, Yanik MF, Zhang JZ, Jin Y, Chisholm AD. Caenorhabditis elegans neuronal regeneration is influenced by life stage, ephrin signaling, and synaptic branching. Proc Natl Acad Sci U S A. 2007;104:15132–15137. [Europe PMC free article] [Abstract] [Google Scholar]
- Ylera B, Erturk A, Hellal F, Nadrigny F, Hurtado A, Tahirovic S, Oudega M, Kirchhoff F, Bradke F. Chronically CNS-injured adult sensory neurons gain regenerative competence upon a lesion of their peripheral axon. Curr Biol. 2009;19:930–936. [Abstract] [Google Scholar]
Full text links
Read article at publisher's site: https://doi.org/10.1016/j.neuron.2015.03.061
Read article for free, from open access legal sources, via Unpaywall:
http://www.cell.com/article/S0896627315002858/pdf
Citations & impact
Impact metrics
Citations of article over time
Alternative metrics
Smart citations by scite.ai
Explore citation contexts and check if this article has been
supported or disputed.
https://scite.ai/reports/10.1016/j.neuron.2015.03.061
Article citations
In vivo imaging in mouse spinal cord reveals that microglia prevent degeneration of injured axons.
Nat Commun, 15(1):8837, 13 Oct 2024
Cited by: 0 articles | PMID: 39397028 | PMCID: PMC11471772
Neuronal maturation and axon regeneration: unfixing circuitry to enable repair.
Nat Rev Neurosci, 25(10):649-667, 20 Aug 2024
Cited by: 0 articles | PMID: 39164450
Review
In vivo imaging of the neuronal response to spinal cord injury: a narrative review.
Neural Regen Res, 19(4):811-817, 01 Apr 2024
Cited by: 0 articles | PMID: 37843216 | PMCID: PMC10664102
Review Free full text in Europe PMC
Diversity in homeostatic calcium set points predicts retinal ganglion cell survival following optic nerve injury in vivo.
Cell Rep, 42(10):113165, 25 Sep 2023
Cited by: 1 article | PMID: 37751356 | PMCID: PMC10947246
PTEN knockout using retrogradely transported AAVs transiently restores locomotor abilities in both acute and chronic spinal cord injury.
Exp Neurol, 368:114502, 08 Aug 2023
Cited by: 3 articles | PMID: 37558155 | PMCID: PMC10498341
Go to all (44) article citations
Data
Data behind the article
This data has been text mined from the article, or deposited into data resources.
BioStudies: supplemental material and supporting data
Similar Articles
To arrive at the top five similar articles we use a word-weighted algorithm to compare words from the Title and Abstract of each citation.
Understanding the axonal response to injury by in vivo imaging in the mouse spinal cord: A tale of two branches.
Exp Neurol, 318:277-285, 12 Apr 2019
Cited by: 13 articles | PMID: 30986398 | PMCID: PMC6588497
Review Free full text in Europe PMC
In vivo imaging of axonal degeneration and regeneration in the injured spinal cord.
Nat Med, 11(5):572-577, 10 Apr 2005
Cited by: 365 articles | PMID: 15821747
AxonTracer: a novel ImageJ plugin for automated quantification of axon regeneration in spinal cord tissue.
BMC Neurosci, 19(1):8, 09 Mar 2018
Cited by: 12 articles | PMID: 29523078 | PMCID: PMC5845359
The Dorsal Column Lesion Model of Spinal Cord Injury and Its Use in Deciphering the Neuron-Intrinsic Injury Response.
Dev Neurobiol, 78(10):926-951, 11 May 2018
Cited by: 17 articles | PMID: 29717546 | PMCID: PMC6221129
Review Free full text in Europe PMC
Funding
Funders who supported this work.
Dana Foundation (4)
Grant ID: R01NS054734
Grant ID: R21NS088536
Grant ID: F31NS074867
Grant ID: F32NS056697
Israel National Road Safety Authority (4)
Grant ID: F31NS074867
Grant ID: F32NS056697
Grant ID: R01NS054734
Grant ID: R21NS088536
NINDS NIH HHS (11)
Grant ID: R21 NS088536
Grant ID: R01 NS093055
Grant ID: R01 NS054734
Grant ID: F31 NS074867
Grant ID: F31NS074867
Grant ID: F32NS056697
Grant ID: P30NS047101
Grant ID: R01NS054734
Grant ID: R21NS088536
Grant ID: F32 NS056697
Grant ID: P30 NS047101
National Institute of Neurological Disorders and Stroke (4)
Grant ID: R01NS054734
Grant ID: F32NS056697
Grant ID: F31NS074867
Grant ID: R21NS088536
National Institutes of Health (4)
Grant ID: F32NS056697
Grant ID: R21NS088536
Grant ID: F31NS074867
Grant ID: R01NS054734