Abstract
Free full text

Leptin Elongates Hypothalamic Neuronal Cilia via Transcriptional Regulation and Actin Destabilization*
Abstract
Terminally differentiated neurons have a single, primary cilium. The primary cilia of hypothalamic neurons play a critical role in sensing metabolic signals. We recently showed that mice with leptin deficiency or resistance have shorter cilia in the hypothalamic neurons, and leptin treatment elongates cilia in hypothalamic neurons. Here, we investigated the molecular mechanisms by which leptin controls ciliary length in hypothalamic neurons. In N1 hypothalamic neuronal cells, leptin treatment increased the expression of intraflagellar transport proteins. These effects occurred via phosphatase and tensin homolog/glycogen synthase kinase-3β-mediated inhibition of the transcriptional factor RFX1. Actin filament dynamics were also involved in leptin-promoted ciliary elongation. Both leptin and cytochalasin-D treatment induced F-actin disruption and cilium elongation in hypothalamic neurons that was completely abrogated by co-treatment with the F-actin polymerizer phalloidin. Our findings suggest that leptin elongates hypothalamic neuronal cilia by stimulating the production of intraflagellar transport proteins and destabilizing actin filaments.
Introduction
Substantial evidence suggests that immotile or primary (9 + 0) cilia serve as a signaling hub. Primary cilia coordinate a variety of signaling pathways, such as Shh, FGF, Notch, mammalian target of rapamycin, PDGF, and Hippo signaling (1, 2). Cilia formation is cell cycle-dependent. Cilia form in the G0 or G1 phase and resorb prior to M phase entry (3). Initiating ciliogenesis requires conversion of the mother centriole to the basal body, which serves as a template for the nucleation of cilary microtubules. Because cilia do not contain the machinery for protein synthesis, ciliary proteins are transported from their synthesis sites in the cell body to the cilium using a microtubule-based transport process known as intraflagellar transport (IFT).3 IFT is a process in which a large protein complex, known as an IFT particle or raft, is conveyed to the distal tip of the cilia by the heterotrimeric kinesin II complex and brought back to the base by a cytoplasmic dynein complex (4). IFT particles are composed of ≥17 polypeptides that are assembled in two complexes, A and B. When the cilia are being assembled, the ciliary proteins are transported to the ciliary tips by IFT complex B (5).
Terminally differentiated neurons have a single primary cilium. In particular, neurons in the hypothalamus, hippocampus, and periventricular area have long primary cilia (6). In the hippocampus, primary cilia regulate the proliferation of neuronal progenitors and the differentiation of newborn neurons (1). Although the role of neuronal cilia in neurobiology is not completely understood, it has been suggested that the neuronal cilium is an important extrasynaptic signaling organelle (7).
Interestingly, a strong association between genetic ciliopathy and obesity has been demonstrated in both humans and animals (8). Patients with human ciliopathies, such as Bardet-Biedl syndrome and Alström syndrome, commonly develop obesity (9). Similarly, mice lacking both Bardet-Biedl syndrome and the IFT proteins develop obesity and hyperphagia (10,–12). Selective inhibition of ciliogenesis in neurons and particularly in the proopiomelanocortin-producing hypothalamic neurons also causes obesity (12), thus indicating the critical role of hypothalamic neuronal cilia in the control of energy balance. We recently reported that obese mice with leptin deficiency demonstrate the short cilia phenotype in the hypothalamus, which is reversed by leptin treatment (13). Moreover, the remarkable shortening of hypothalamic neuronal primary cilia was observed in diet-induced obese mice with leptin resistance. In contrast, artificially inducing the short phenotype in the hypothalamic cilia of adult mice leads to increased food intake and body weight and an impaired anorexigenic response to physiological satiety signals, such as leptin, insulin, and glucose (13). These data suggest that ciliary length in hypothalamic neurons is important for regulating appetite and body weight. Here, we investigated the molecular mechanisms by which leptin regulates the lengths of the primary cilia in hypothalamic neurons.
Experimental Procedures
Cell Culture
N1 murine hypothalamic neuronal cells and SH-SY5Y human neuroblastoma cells were maintained in DMEM supplemented with 10% fetal bovine serum, penicillin, and streptomycin (100 units/ml each). Primary cultured hypothalamic neurons were prepared from rat fetal brain (embryonic day 18) and cultured as described previously (14). Human retinal pigmented epithelial (RPE-1) cells were maintained in DMEM/F-12 containing the same supplements. To induce ciliogenesis, the culture medium was replaced with medium without supplements when the cells were ~90% confluent. Cells were then cultured in serum-free medium for 48 h before ciliary analysis. Cells were treated with leptin (R&D Systems; 10–1000 nm), phalloidin (10 nm), or cytochalasin-D (0.2 μm) (all compounds obtained from Sigma) for the indicated time periods before analysis.
Ciliary Staining
To stain the cilia of the neuronal cells, the cells were fixed in 4% paraformaldehyde for 15 min at room temperature and incubated with primary antibodies against acetylated α-tubulin (1:1000; mouse; Sigma) or adenylyl cyclase-3 (1:500; rabbit; Santa Cruz Biotechnology) at 4 °C for 48 h. After washing, the slides were incubated with Alexa Fluor-conjugated secondary antibody (1:1000; Invitrogen) at room temperature for 1 h. Nuclei were stained with DAPI for 10 min before mounting.
Measuring the Lengths and Numbers of Cilia
The number and length of the hypothalamic neuronal cilia were measured using Image-Pro Plus (Media Cybernetics Inc., Silver Spring, MD). The cilia of the neuronal cells were analyzed in randomly selected fields (≥100 cilia/well). The percentage of ciliated cells was calculated as the number of cilia divided by the number of DAPI-stained nuclei in 10 selected fields. Each treatment was performed in duplicate or triplicate and repeated in ≥3 independent batches.
Immunoblotting and Immunoprecipitation
Fifty micrograms of cell lysates were separated using 8% SDS-PAGE and transferred to PVDF membranes (GE Healthcare). After incubation in the blocking buffer, the membranes were incubated overnight at 4 °C with antibodies against PTEN (1:1000; rabbit; Cell Signaling Technology), GSK3β (1:1000; rabbit; Cell Signaling Technology), KIF3A (1:2000; rabbit; Abcam), IFT88 (1:1000; rabbit; Proteintech), or GAPDH (1:5000; rabbit; Bethyl Laboratories). Blots were developed using horseradish peroxidase (HRP)-linked secondary antibody and a chemiluminescent detection system (New England Biolabs). For the immunoprecipitation assay, 500 μg of protein extract was incubated overnight with 1 μg of primary antibodies against RFX1 (rabbit; Aviva Systems Biology) or an equivalent amount of rabbit immunoglobulin (IgG) before adding 100 μl of agarose beads (Millipore). Subsequently, the immunoprecipitate was subjected to immunoblotting using antibodies specific to RFX1 (1:1000; Aviva Systems Biology), GSK3β, phosphorylated serine/threonine (1:1000; Cell Signaling Technology), or β-actin (1:1000; mouse; Santa Cruz Biotechnology).
F-actin Staining
Cells were fixed with 4% paraformaldehyde for 15 min at room temperature. Cells were incubated with Alexa Fluor 488-phalloidin (1:400; Molecular Probes) at room temperature for 2 h and then with DAPI for 10 min. Fluorescence intensity was measured in 10 fields/well using ImageJ software and divided by the DAPI counts. Transfections or treatments were performed in duplicate, and experiments were repeated ≥3 times.
Real Time PCR
Cells were treated with leptin (10–100 nm) with or without LY294002 (1 nm) for 2 h or transfected with 1 μg of plasmids expressing PTEN and Gsk3β for 48 h before harvesting. Total RNA was extracted from the N1 cells using TRIzol reagent (Invitrogen) and quantified using spectrophotometry. Real time PCR analysis was performed using the primer sets in Table 1 to determine the mRNA levels of the ciliary proteins (KIF3A, KIF3B, IFT20, IFT27, IFT54, IFT88, IFT140, and IFT144) and transcription factors RFX1, -2, -3, and -4. The expression of each mRNA was normalized to GAPDH mRNA.
TABLE 1
Primer sequences used in the real time PCR analysis of the ciliary proteins
Gene | Primer (5′ to 3′) |
---|---|
Kif3a | |
![]() ![]() ![]() ![]() | AGCTGCGATAATGTGAAGGTG |
![]() ![]() ![]() ![]() | GTTCCCCTCATTTCATCCACG |
Kif3b | |
![]() ![]() ![]() ![]() | GGACTGTGTGTGGAGCCTTG |
![]() ![]() ![]() ![]() | CTGACAGAGGCAGGCACCTA |
Ift20 | |
![]() ![]() ![]() ![]() | AGGCAGGGCTGCATTTTGAT |
![]() ![]() ![]() ![]() | CAAGCTCAATTAGACCACCAACA |
Ift27 | |
![]() ![]() ![]() ![]() | GGGACCCATTTCCAGAAGAA |
![]() ![]() ![]() ![]() | CCGGCTGAGTCAAAAATGAA |
Ift54 | |
![]() ![]() ![]() ![]() | TGTGTAAGAGTGCGCTTCCC |
![]() ![]() ![]() ![]() | GCATGCTGCCTGTTCTCACT |
Ift88 | |
![]() ![]() ![]() ![]() | GCAGTGACAGTGGCCAGAAC |
![]() ![]() ![]() ![]() | AAGGTTCATCTGTCCCAGGC |
Ift140 | |
![]() ![]() ![]() ![]() | CTTCAGACCCCTACTGAGCTT |
![]() ![]() ![]() ![]() | GTGTGACGACATGGCTTGC |
Ift144 | |
![]() ![]() ![]() ![]() | CAGTATTCACGGGCACTAAAG |
![]() ![]() ![]() ![]() | GTACTTGGCGTCCTTTGGC |
Rfx1 | |
![]() ![]() ![]() ![]() | TACCACTATTACGGCCTGCG |
![]() ![]() ![]() ![]() | ATGGGCTTCAGCCTCTGTTT |
Rfx2 | |
![]() ![]() ![]() ![]() | ACCTCATCCGGCTGCTCTAT |
![]() ![]() ![]() ![]() | GCCTCACTGCTGTGTCCATC |
Rfx3 | |
![]() ![]() ![]() ![]() | CGAGAGCACTGTGAGGCAAT |
![]() ![]() ![]() ![]() | TTGCTTGATTCCGTGATGGT |
Gapdh | |
![]() ![]() ![]() ![]() | ACTCTTCCACCTTCGATGC |
![]() ![]() ![]() ![]() | CCTGTTGCTGTAGCCGTAT |
Promoter Analysis
The promoter regions of the genes encoding human KIF3A (nucleotide positions −1067 to +64), mouse Ift88 (nucleotide positions −913 to +81), and mouse Ift20 (nucleotide positions −1111 to +60) were cloned and ligated into the pGL3 vector (Promega). N1 cells were plated onto 12-well plates and transfected with promoter-reporter constructs (50 ng) and expression plasmids for Gsk3β (10–100 ng), wild-type and mutant RFX1 (100 or 500 ng), and CMV-β-gal (25 ng) using Lipofectamine (Invitrogen). After 48 h of transfection, the cells were harvested to assay luciferase and β-galactosidase activities. Luciferase activity was normalized to β-galactosidase activity. Data are shown as -fold increases over the controls. Transfections were performed in triplicate, and the experiments were repeated ≥2 times.
Chromatin Immunoprecipitation (ChIP)
SH-SY5Y human neuroblastoma cells were transfected with either the expression vectors for PTEN and Gsk3β or a mock plasmid (5 μg). After 48 h of transfection, the mock DNA-transfected cells were treated with 100 nm leptin for 1 h prior to fixation with formaldehyde, then lysed, and sonicated. The mock DNA-transfected cells not treated with leptin were used as the control. Soluble chromatin was co-immunoprecipitated with 1 μg of anti-RFX1 antibody (goat; Santa Cruz Biotechnology) or an equivalent amount of rabbit IgG. After decross-linking DNA, the chromatin samples were subjected to PCR to amplify the X-box binding region of the human KIF3A gene (nucleotide positions −362 to +44) using the following primers: 5′-CTCTGTGCCAGGCTCTGTGT-3′ (forward) and 5′-AAGCCCAGTCTCAGGCTCTC-3′ (reverse).
RNA Silencing
Cells were transfected with 50 nm siRNA for control or siRNAs specific to murine Pten, Gsk3β, and Rfx1 (Dharmacon) (all at 50 nm) using Lipofectamine.
Site-specific Mutagenesis
Potential GSK3β phosphorylation sites on RFX1 were predicted using Scansite (15). RFX1 phosphorylation mutants were generated from human RFX1 using the QuikChange site-directed mutagenesis kit (Stratagene) and confirmed by DNA sequencing.
Statistical Analysis
Data are presented as the means ± S.E. Statistical analysis was performed using SPSS-PC13 (SPSS, Chicago, IL). Statistical significance between groups was tested using one-way or two-way analysis of variance, followed by the post hoc least significant difference test. Significance was defined as p < 0.05.
Results
Leptin Treatment Elongates Hypothalamic Neuronal Cilia under Serum-free Conditions
We first investigated the effects of leptin on hypothalamic neuronal cilia using N1 hypothalamic neuronal cells as these cells are well ciliated and express functional leptin receptors (13). Upon 90% confluence, N1 cells were further cultured in DMEM with or without serum (10% FBS) for 48 h until ciliary analysis. Cilia were analyzed using immunostaining for the antibody against acetylated α-tubulin. In N1 cells cultured in serum-containing medium, the average ciliary length was 1.3 μm, and the percentage of ciliated cells was ~48% (Fig. 1, A and B). Serum starvation significantly increased both ciliary length (~1.9 μm) and the percentage of ciliated cells (~64%).

Leptin treatment elongates primary cilia in serum-starved hypothalamic neuronal cells. A–C, effects of leptin on the primary cilia in hypothalamic neuronal cells. N1 hypothalamic neuronal cells were cultured in DMEM with or without serum (10% FBS) for 48 h and then treated with leptin (100 nm) for 6 or 12 h before ciliary analysis. Cilia were stained using antibodies specific to acetylated α-tubulin (A and B) or adenylyl cyclase-3 (C). D, leptin treatment (100 nm) did not affect the ciliary lengths of human RPE-1 cells. *, p < 0.01 between the indicated groups. Data shown are the means ± S.E. (error bars). Independent experiments were performed in duplicate and repeated ≥3 times. Scale bars, 25 μm.
Consistent with the results of our previous study (13), leptin treatment (100 nm for 6 or 12 h) increased the ciliary lengths of the N1 cells up to ~3.0 μm under serum-free conditions (Fig. 1A), although treatment did not alter the number of ciliated cells (Fig. 1B). These results indicate that leptin enhances the growth of preformed cilia but not ciliogenesis in N1 cells. Interestingly, these effects were not observed in the cells that were cultured in serum-containing medium (Fig. 1A). Ciliary analysis using another ciliary marker, adenylyl cyclase-3 (8, 10), confirmed the effects of leptin on ciliary lengths in serum-starved N1 cells (Fig. 1C). In RPE-1 cells, leptin treatment (100 nm for 6–12 h) increased neither the lengths of the cilia nor the percentage of ciliated cells (Fig. 1D), indicating that leptin regulation of ciliary length may be specific to hypothalamic neuronal cells.
Leptin Regulates the IFTmRNA and Protein Expression by Inhibiting PTEN and GSK3β
To investigate the molecular mechanisms involved in leptin-stimulated elongation of cilia, we assessed whether leptin affects the expression levels of the IFT motor subunits Kif3a and Kif3b and the IFT cargo complex B components Ift88, Ift20, Ift27, and Ift54. All of these components are known mediators of anterograde IFT. Treating N1 cells with leptin (100 nm for 2 h) significantly increased the mRNA levels of these IFT proteins (Fig. 2A). We also determined the effects of leptin on the expression of IFT complex A components Ift140 and Ift144, which are involved in retrograde IFT. Interestingly, leptin did not alter the expression of complex A components (Fig. 2A).
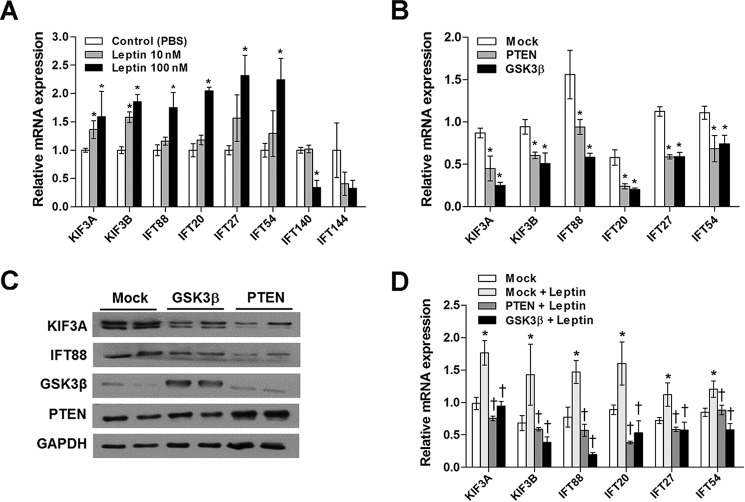
Leptin increases IFT protein expression by inhibiting PTEN and GSK3β. A, real time PCR analysis showing the effects of leptin treatment (2 h) on the mRNA expression levels of IFT proteins in N1 cells (n = 3). *, p < 0.05 versus PBS-treated controls. B and C, a decrease in the mRNA and protein expression of IFT components in N1 cells overexpressing PTEN or Gsk3β (50 ng). *, p < 0.05 versus mock controls. D, effects of leptin treatment (100 nm) on IFT component expression in N1 cells overexpressing PTEN and Gskβ (n = 3). *, p < 0.05 versus leptin-untreated and mock DNA-transfected control. †, p < 0.05 versus the leptin-treated and mock DNA-transfected groups. Data are shown as the means ± S.E. (error bars). Each treatment was performed in triplicate and repeated ≥2 times.
In our previous study, leptin treatment augmented the expression of phosphorylated PTEN and GSK3β in hypothalamic neuronal cells (16), which led to repressed PTEN-GSK3β signaling. Moreover, the study showed that leptin treatment increased ciliary length by inhibiting the PTEN-GSK3β pathway. Therefore, we investigated whether PTEN and GSK3β mediate the effects of leptin on IFT expression. In contrast to leptin treatment, overexpressing either PTEN or Gsk3β in N1 cells reduced the mRNA expression levels of anterograde IFT proteins (Fig. 2B) as well as the protein expression levels of KIF3A and IFT88 (Fig. 2C). Furthermore, PTEN and Gsk3β overexpression blocked the stimulatory effects of leptin on these IFT proteins (Fig. 2D). These findings imply that leptin up-regulates IFT gene expression by inhibiting PTEN and GSK3β signaling.
RFX1 Mediates Transcriptional Regulation of Ciliary Proteins by Leptin
In Caenorhabditis elegans, metazoans, and mammals, RFX transcription factors regulate ciliogenesis by binding to an X-box motif in the promoter region of the genes that encode ciliary proteins (17,–19). We found potential RFX-binding sites in the promoter of the human KIF3A gene (Fig. 3A). To test the role of the RFXs in the transcriptional regulation of IFT proteins by leptin, we first examined the effects of leptin on RFX expression. Treatment with leptin (100 nm) for 4 h significantly increased the expression of Rfx1 mRNA but decreased expression of both Rfx2 and Rfx3 (Fig. 3B). Rfx4 expression was hardly detectable in N1 cells (data not shown).
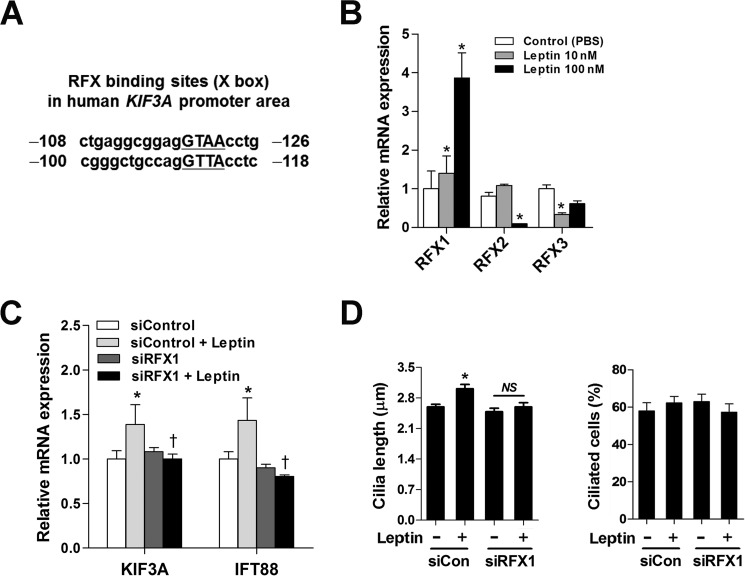
RFX1 mediates leptin-stimulated transcription of the ciliary genes. A, potential RFX-binding sites on the human KIF3A promoter. B, effects of leptin (100 nm for 2 h) on the mRNA expression of Rfxs in N1 cells. *, p < 0.05 versus PBS-treated controls. C and D, Rfx1 knockdown blocked leptin-stimulated IFT gene transcription and cilium elongation. N1 cells were transfected with either control siRNA (siControl or siCon) or siRfx1 (50 nm each). After transfection, cells were cultured for 48 h under serum-starved conditions and then treated with leptin (100 nm) for 2 h (C) or 16 h (D) before analysis. *, p < 0.05 versus siControl; †, p < 0.05 versus siControl + leptin. NS, not significant. Data are shown as the means ± S.E. (error bars). Each treatment was performed in triplicate and repeated ≥2 times.
To determine whether the leptin-regulated transcription of ciliary proteins is mediated by RFX1, we silenced endogenous RFX1 expression by transfecting a small interfering RNA to target murine Rfx1 (siRfx1). siRfx1 expression did not significantly reduce Kif3a or Ift88 mRNA expression or ciliary length in leptin-untreated cells (Fig. 3, C and D). However, siRfx1 expression completely blocked leptin-induced KIF3A and IFT88 expression as well as ciliary elongation (Fig. 3, C and D), demonstrating that RFX1 mediates leptin regulation of the transcription of these ciliary proteins.
Then, using a chromatin immunoprecipitation assay, we investigated whether leptin affects RFX1 binding to the X-box-containing promoter region (Fig. 3A) of the human KIF3A gene in human neuroblastoma SH-SY5Y cells. Indeed, leptin treatment significantly enhanced RFX1 binding to the KIF3A promoter region, including the RFX-binding sites (Fig. 4A). In contrast, the binding of RFX1 to the KIF3A promoter was significantly reduced in cells that overexpressed either PTEN or Gsk3β (Fig. 4A), suggesting that leptin and PTEN-GSK3β signaling may regulate the production of IFT proteins by controlling RFX1 binding to the promoter of the IFT proteins.

GSK3β inhibits RFX1 via direct phosphorylation. A, ChIP assay showing that leptin treatment (100 nm for 1 h) stimulated RFX1 binding to the KIF3A promoter, whereas PTEN and Gsk3β overexpression inhibited it. There are no differences in the amounts of input DNA between the groups. B, luciferase assay showing the inhibitory effects of GSK3β on RFX1-driven KIF3A gene expression. Using N1 cells, each luciferase reporter plasmid containing the KIF3A, Ift88, or Ift20 promoter was cotransfected with the expression vectors for RFX1 (500 ng) and Gsk3β (10, 50, and 100 ng) (n = 4). *, p < 0.05 versus mock DNA-transfected control. †, p < 0.05 versus cells overexpressing RFX1 alone. C, N1 cells were transfected with the Gsk3β-expressing plasmid. Cell lysates were immunoprecipitated (IP) using the IgG or RFX1 antibodies and then subjected to immunoblotting (IB) using antibodies against GSK3β, RFX1, phospho-serine/threonine (p-Ser/Thr), and β-actin. D, RFX1 mutations (100 ng) at potential GSK3β phosphorylation sites (Ser-120 and Thr-124) increased basal KIF3A transcriptional activity and blocked the GSK3β-mediated inhibition of RFX1 (n = 4). *, p < 0.05; **, p < 0.005 versus mock DNA-transfected control. †, p < 0.05 versus cells expressing wild-type RFX1 (100 ng). NS, not significant. Data are presented as the means ± S.E. (error bars). Cont, control.
RFX1 overexpression in N1 cells stimulated the promotor activities of KIF3A, Ift88, and Ift20 (Fig. 4B). Thus, we confirmed that RFX1 serves as a positive regulator of these ciliary genes in hypothalamic neuronal cells. GSK3β regulates a number of transcriptional factors through substrate phosphorylation (20). Therefore, we investigated whether GSK3β affects the transcriptional activity of RFX1. Notably, the co-expression of Gsk3β with RFX1 significantly inhibited the RFX1-stimulated transcription of KIF3A, Ift88, and Ift20 in a dose-dependent manner (Fig. 4B). These findings suggest that GSK3β negatively regulates RFX1 transcriptional activity.
To further investigate the physical interactions between RFX1 and GSK3β, protein extracts from N1 cells underwent immunoprecipitation using the anti-RFX1 antibody and then immunoblotting using antibodies specific to GSK3β and RFX1. As shown in Fig. 4C, RFX1 was directly associated with GSK3β. Moreover, in Gsk3β-overexpressing cells, RFX1 phosphorylation at the serine and threonine residues was higher, but total RFX1 protein expression appeared to be lower in comparison with the control cells that were transfected with mock DNA (Fig. 4C). These findings suggest that RFX1 may be a direct phosphorylation target of GSK3β.
Next, we examined whether GSK3β-mediated RFX1 phosphorylation affects its regulatory activities toward ciliary gene transcription. Using the Scansite program to predict the phosphorylation sites, we found consensus GSK3β phosphorylation sites (Ser-120 and Thr-124) in human RFX1. We then tested whether GSK3β-mediated RFX1 phosphorylation at these residues affects the ability of RFX1 to regulate IFT gene transcription. To do this, we generated phosphorylation-defective RFX1 mutants at Ser-120 and Thr-124 (S120A and S120A/T124A). The expression of these RFX1 mutants completely prevented GSK3β-induced inhibition of KIF3A promoter activity (Fig. 4D). Furthermore, basal KIF3A promoter activity was greater in the cells that expressed RFX1 mutants than in cells expressing wild-type RFX1 (Fig. 4D), suggesting that basal RFX1 transcriptional activity may be under inhibition of endogenous GSK3β. These results confirm that GSK3β inhibits RFX1 transcriptional activity via the direct phosphorylation of RFX1.
F-actin Depolymerization Is Important for Leptin-promoted Cilium Assembly
A recent study reported a connection between actin cytoskeleton and ciliogenesis (21). Because leptin treatment induces actin depolymerization in pancreatic β-cells and hypothalamic neuronal cells (22, 23), we examined the possible contribution of actin dynamics toward leptin-promoted ciliary growth. To do this, we treated the N1 cells with leptin (100 nm for 16 h) and evaluated F-actin network organization by immunostaining for F-actin using fluorescence-labeled phalloidin. The effects of leptin on the actin cytoskeleton were compared with the actin filament disruptor cytochalasin-D (0.2 μm for 16 h). As expected, cytochalasin-D treatment profoundly disrupted the linear arrangement of the F-actin fibers and decreased the intensity of F-actin fluorescence (Fig. 5A). Leptin treatment induced a modest but significant reduction in F-actin fiber intensity (Fig. 5A).
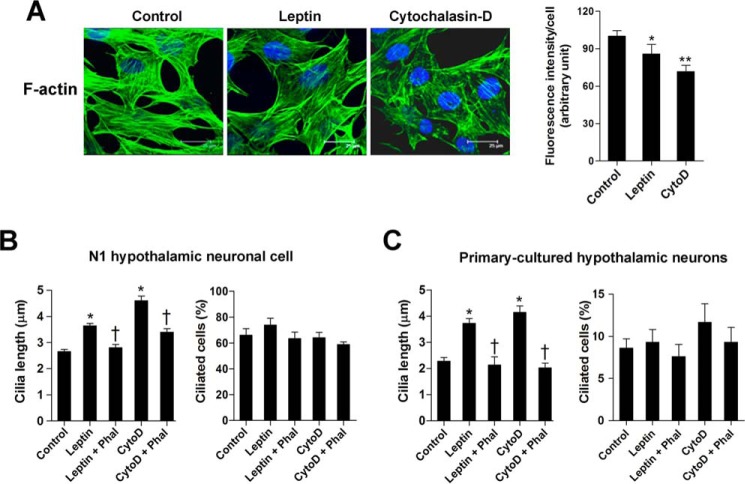
Leptin and cytochalasin-D induce ciliary elongation and F-actin depolymerization. A, F-actin polymerization in N1 cells treated with leptin (100 nm) or the actin depolymerizer cytochalasin-D (CytoD) (0. 2 μm) for 16 h. F-actin was stained using Alexa Fluor 488-conjugated phalloidin. The intensity of F-actin fluorescence was determined in 10 fields/well and divided by the number of cells counterstained with DAPI. *, p < 0.05; **, p < 0.005 versus control. B and C, effects of leptin (100 nm) or cytochalasin-D (0.2 μm) on the growth of primary cilia in N1 cells (B) and primary hypothalamic neurons (C) in the presence or absence of phalloidin (Phal) (10 nm). *, p < 0.05 versus controls. †, p < 0.05 versus leptin or cytochalasin-D treatment alone. Data are shown as the means ± S.E. (error bars). Scale bars, 25 μm.
Treating N1 cells with either leptin (100 nm) or cytochalasin-D (0.2 μm) for 16 h increased the ciliary length by 37% and 64%, respectively (Fig. 5B). Notably, co-treatment with the actin polymerizer phalloidin almost completely blocked the stimulatory effects of leptin and cytochalasin-D on ciliary length (Fig. 5B). Similar to N1 cells, both leptin and cytochalasin-D increased ciliary length in the primary cultures of hypothalamic neurons (Fig. 5C). In contrast, no significant changes were observed in the ciliated cell percentages in either cell type (Fig. 5, B and C). These data strongly suggest that F-actin depolymerization is an important downstream signaling pathway involved in leptin regulation of ciliary assembly in hypothalamic neurons.
PTEN and GSK3β Control Ciliary Length by Repressing F-actin Polymerization
In previous studies, leptin treatment induced F-actin depolymerization in pancreatic β-cells and hypothalamic neurons via PTEN-GSK3β signaling (22). Therefore, we investigated whether PTEN and GSK3β regulate ciliary lengths by modulating the actin cytoskeleton. To do this, we depleted PTEN and GSK3β expression in N1 cells by transfecting siRNAs that target murine PTEN and GSK3β. Similarly to leptin and cytochalasin-D, depleting PTEN and GSK3β induced F-actin destabilization, which was reversed by co-treatment with the F-actin polymerizer phalloidin (Fig. 6, A and B). Ciliary analysis, which was performed in parallel, revealed that phalloidin co-treatment prevented ciliary elongation that was induced by PTEN and GSK3β knockdown (Fig. 6C). These data highlight how actin filament rearrangement is a critical downstream pathway in the control of cilium growth by PTEN-GSK3β signaling in hypothalamic neurons.

PTEN and GSK3β control ciliary lengths via F-actin reorganization. A and B, F-actin staining in N1 cells transfected with siRNAs (si) (50 nm each for 48 h) that targeted murine Pten and Gsk3β and treated with or without phalloidin (10 nm for 16 h). The intensity of F-actin fluorescence was determined in 10 fields/well and divided by the number of DAPI-stained nuclei. *, p < 0.05 for the indicated groups. C, changes in ciliary lengths in the N1 cells transfected with Pten siRNA or Gsk3β siRNA (50 nm each for 48 h) with or without phalloidin (Phal) (10 nm for 16 h). *, p < 0.01 between the indicated groups. Data are presented as the means ± S.E. (error bars). D, schematic diagram depicting molecular mechanisms for leptin control of hypothalamic neuronal cilia. Cont, control. Scale bars, 25 μm.
Discussion
In our current study, leptin increased the ciliary lengths in N1 hypothalamic neuronal cells and primary cultures of hypothalamic neurons. In contrast, leptin did not cause any ciliary changes in RPE-1 cells, which are commonly used for ciliary studies (21, 24). Hypothalamic neurons are a primary target for the in vivo actions of leptin (25). Moreover, altered metabolism or leptin treatment selectively causes ciliary changes in hypothalamic neurons (13). Thus, leptin-mediated regulation of ciliary length may specifically occur in hypothalamic neurons.
Ciliogenesis is closely linked to the cell cycle. Ciliary assembly usually occurs in the G1 or G0 phase of the cell cycle and resolves before entry into the M phase as the basal body is converted to the centriole and then used for spindle attachment during mitosis. Serum starvation is widely used to stimulate ciliogenesis in cultured cells as these cells do not undergo mitosis under serum-deprived conditions. Consistently, in this study, 48 h of serum starvation markedly increased both ciliary length and ciliogenesis in N1 hypothalamic cells. Notably, leptin-induced ciliary elongation was apparent in the cells that were cultured to full confluence under serum-free medium when most cells would remain in the quiescent state. In contrast, the effects of leptin were insignificant in N1 cells cultured with serum, implying that leptin regulates ciliary growth via cell cycle-independent mechanisms.
Conversely, despite its significant effect on ciliary length, leptin did not increase the percentage of ciliated cells in hypothalamic neuronal cells. These findings suggest that leptin may not stimulate ciliogenesis in these cells. Because terminally differentiated neurons already have a single primary cilium, controlling ciliogenesis via leptin in hypothalamic neurons might be unnecessary in adulthood.
Ciliary assembly and disassembly occur at the tip by adding or removing axonemal subunits. Steady-state assembly is balanced by the continuous removal of subunits from the tip of the cilium (5). Because cilia disassemble at a constant rate, ciliary length is thought to be determined by modulating ciliary assembly (26). For ciliary assembly, the ciliary proteins are synthesized in the cytoplasm and then transported to the ciliary tip by kinesin motor-driven IFT (5). Therefore, IFT plays an indispensable role in ciliary formation and elongation. In particular, the IFT B-complex responsible for anterograde transport is essential for the assembly and maintenance of cilia. Indeed, the loss of B-complex components causes the shortening and absence of cilia (27, 28). Here, leptin treatment increased the cellular expression of IFT B-complex components (Ift20, Ift27, Ift54, and Ift88) as well as kinesin II subunits (Kif3a and Kif3b), which may have led to the increased delivery flux of ciliary components. Therefore, the effect of leptin on ciliary assembly may be mediated by the enhanced production of the anterograde IFT complex and kinesin components, which is similar to FGF regulation of ciliary length (29).
RFX transcription factors, which are homologs of Daf-19 in C. elegans and bind to the X-box motifs in the promotor region, are known as major regulators of ciliary gene expression (17, 26, 30, 31). Seven RFX genes, RFX1–7, have been identified in mammals. Different mammalian RFX genes show differential but overlapping expression patterns (19), thus suggesting that they have complementary and cooperative roles in regulating the genes involved in many different biological pathways. RFX2 is preferentially expressed in ciliated tissues and is implicated in ciliogenesis in vertebrates (31,–33). RFX3 plays a critical role in the development of nodal cilia and left-right asymmetry. Depleting RFX3 in mice results in abnormal cilium development in both the brain (34) and pancreas (35). RFX4 mediates sonic hedgehog signaling during the development of the central nervous system (36). RFX7 controls ciliogenesis in the neural tube by regulating RFX4 expression (30). To the best of our knowledge, there are no studies on the involvement of RFX1, RFX5, and RFX6 in the regulation of ciliogenesis.
We discovered the X-box motifs in the promoter of the human KIF3A gene. To identify the RFXs that mediate the actions of leptin on ciliary genes, we examined the effects of leptin on the expression of various Rfxs in N1 cells. Interestingly, leptin treatment selectively increased Rfx1 mRNA expression in N1 cells but suppressed Rfx2 and Rfx3 expression. Thus, we hypothesized that RFX1 may play an important role in the regulation of ciliary genes via leptin in hypothalamic neuronal cells. In support of this theory, RFX1 overexpression stimulated the promotor activities of KIF3A, Ift88, and Ift20 in N1 cells. Leptin treatment increased RFX1 binding to the X-box on the human KIF3A promotor. Conversely, depleting RFX1 inhibited the stimulatory effects of leptin on Kif3a and Ift88 expression, indicating that RFX1 is an important downstream mediator of leptin regulation of ciliary gene transcription. Foxj1 is another important transcriptional regulator that mediates FGF-induced ciliogenesis (29). Therefore, it would be worth examining the involvement of Foxj1 in leptin control of ciliary assembly.
Substantial evidence suggests the involvement of GSK3β in the control of ciliary length. Treatment with lithium chloride, a GSK3β inhibitor, alters flagellar lengths in Chlamydomonas (37). Moreover, lithium chloride induces ciliary elongation in mammalian cells (38). In the kidney tubules, GSK3β plays a role in maintaining the cilia via interactions with the von Hippel-Lindau tumor suppressor. Inactivating both genes causes ciliary loss and renal cyst formation (39). Similarly, PTEN suppresses kidney cyst formation in cooperation with the von Hippel-Lindau tumor suppressor (40). In our previous study, leptin caused serial phosphorylation of PTEN and GSK3β and thus repressed theses enzymes in hypothalamic neurons. Furthermore, leptin-induced cilium elongation was abrogated by PTEN and GSK3β overexpression (13). However, the downstream mechanisms of PTEN and GSK3β remain unknown.
Here, we demonstrate that PTEN and GSK3β serve as critical signaling nodes in leptin regulation of ciliary genes. In hypothalamic neuronal cells, overexpressing PTEN and Gsk3β significantly suppressed the mRNA and protein expression of Ift genes. Moreover, overexpressing PTEN and Gsk3β inhibited the effect of leptin on these genes, suggesting the pivotal role of PTEN-GSK3β signaling in the leptin signaling pathway. Regarding molecular mechanisms, we found that PTEN and Gsk3β overexpression inhibited RFX1 binding to the KIF3A gene promotor. In addition, GSK3β inhibited the ability of RFX1 to stimulate the transcriptional activity of ciliogenic genes. These data imply that PTEN and GSK3β regulate ciliary genes by inhibiting RFX1. Notably, an immunoprecipitation study reported that GSK3β directly binds RFX1 in N1 cells. Furthermore, Gsk3β overexpression increased RFX1 phosphorylation in serine and threonine residues, suggesting that RFX1 may be a potential substrate of GSK3β. In support of this, we found that Ser-120 and Thr-124 in human RFX1 are potential GSK3β phosphorylation sites. Indeed, mutation at these sites blocked the inhibitory effects of GSK3β on RFX1-induced KIF3A transcription, confirming that GSK3β negatively regulates RFX1 via direct phosphorylation at Ser-120 and Thr-124 residues.
Emerging evidence suggests a close link between actin dynamics and ciliogenesis or cilium elongation (23). In mammalian cells, blocking F-actin polymerization induced by cytochalasin-D treatment or silencing F-actin polymerization regulators (e.g. gelsolin, advillin, and ARP3) stimulates ciliogenesis and cilium elongation (21). Consistently, mir-129-3p overexpression promotes ciliary assembly by down-regulating the positive regulators of F-actin, such as ABLIM1, ABLIM3, TOCA1, and ARP2 (24). Branched F-actin may affect ciliary growth by modulating the trafficking of ciliary protein-containing vesicles to the mother centriole or basal body (41). In support of this, inhibiting F-actin polymerization by ARP3 ablation, cytochalasin-D treatment, or mir-129-3p overexpression can induce the centrosomal accumulation of ciliogenic vesicles (21, 24).
Like cytochalasin-D, leptin treatment caused F-actin destabilization, although this effect was modest in comparison with cytochalasin-D. The effects of leptin on the cilia were completely blocked by co-treatment with the F-actin polymerizer phalloidin, indicating that disrupting actin filaments is an important part of how leptin stimulates ciliary assembly. Similarly, Pten and Gsk3β knockdown caused F-actin destabilization. Thus, PTEN and GSK3β seem to serve as downstream signals for leptin-caused F-actin rearrangement. In agreement with our results, leptin treatment also causes F-actin depolymerization via PTEN and GSK3β in hypothalamic neuronal cells (16, 22). PTEN directly regulates actin-depolymerizing factor cofilin-1 in alveolar macrophages (42). GSK3β controls actin filament dynamics by activating Rac/Arl6 and Rho GTPase (43). Further studies are needed to identify the molecular targets of PTEN and GSK3β in leptin-induced actin reorganization. In summary, leptin positively regulates the length of hypothalamic neuron cilia via its actions on the transcription of ciliogenic genes and the actin cytoskeleton.
Author Contributions
G. M. K. and Y. M. H. conducted and designed experiments, performed data analyses, and wrote the manuscript. H. W. K. and J. K. designed experiments. B. C. O. provided the mutant RFX1 construct. I. K. wrote the revised manuscript. M.-S. K. supervised the project, designed experiments, and edited the manuscript.
*This work was supported by National Research Foundation of Korea Grants NRF-2013M3C7A1056024 and NRF-2014R1A6A3A01057664 and Asan Institute for Life Sciences Grant 13-326. The authors declare that they have no conflicts of interest with the contents of this article.
3The abbreviations used are:
- IFT
- intraflagellar transport
- PTEN
- phosphatase and tensin homolog
- GSK3β
- glycogen synthase kinase-3β
- RPE-1
- retinal pigmented epithelial
- RFX
- regulatory factor X.
References
Articles from The Journal of Biological Chemistry are provided here courtesy of American Society for Biochemistry and Molecular Biology
Full text links
Read article at publisher's site: https://doi.org/10.1074/jbc.m115.639468
Read article for free, from open access legal sources, via Unpaywall:
http://www.jbc.org/content/290/29/18146.full.pdf
Citations & impact
Impact metrics
Citations of article over time
Alternative metrics
Smart citations by scite.ai
Explore citation contexts and check if this article has been
supported or disputed.
https://scite.ai/reports/10.1074/jbc.m115.639468
Article citations
Primary cilia-associated signalling in squamous cell carcinoma of head and neck region.
Front Oncol, 14:1413255, 21 Aug 2024
Cited by: 0 articles | PMID: 39234399 | PMCID: PMC11372790
Review Free full text in Europe PMC
Postnatal Dynamic Ciliary ARL13B and ADCY3 Localization in the Mouse Brain.
Cells, 13(3):259, 30 Jan 2024
Cited by: 0 articles | PMID: 38334651 | PMCID: PMC10854790
The role of primary cilia in thyroid diseases.
Front Endocrinol (Lausanne), 14:1306550, 08 Jan 2024
Cited by: 0 articles | PMID: 38260150 | PMCID: PMC10801159
Review Free full text in Europe PMC
Regulation of the length of neuronal primary cilia and its potential effects on signalling.
Trends Cell Biol, 33(11):979-990, 10 Jun 2023
Cited by: 8 articles | PMID: 37302961 | PMCID: PMC7615206
Review Free full text in Europe PMC
LCRMP-1 is required for spermatogenesis and stabilises spermatid F-actin organization via the PI3K-Akt pathway.
Commun Biol, 6(1):389, 10 Apr 2023
Cited by: 4 articles | PMID: 37037996 | PMCID: PMC10086033
Go to all (27) article citations
Data
Similar Articles
To arrive at the top five similar articles we use a word-weighted algorithm to compare words from the Title and Abstract of each citation.
Leptin-promoted cilia assembly is critical for normal energy balance.
J Clin Invest, 124(5):2193-2197, 25 Mar 2014
Cited by: 62 articles | PMID: 24667636 | PMCID: PMC4001529
Mechanisms of Weight Control by Primary Cilia.
Mol Cells, 45(4):169-176, 01 Apr 2022
Cited by: 12 articles | PMID: 35387896 | PMCID: PMC9001153
Review Free full text in Europe PMC
A novel leptin signalling pathway via PTEN inhibition in hypothalamic cell lines and pancreatic beta-cells.
EMBO J, 25(11):2377-2387, 04 May 2006
Cited by: 74 articles | PMID: 16675953 | PMCID: PMC1478173
Leptin-dependent phosphorylation of PTEN mediates actin restructuring and activation of ATP-sensitive K+ channels.
J Biol Chem, 284(14):9331-9340, 10 Feb 2009
Cited by: 26 articles | PMID: 19208634 | PMCID: PMC2666585